Effects of L-arginine on impaired blood fluidity after high-intensity exercise: An in vitro evaluation
Abstract
BACKGROUND:
Exercise-induced impairment of blood fluidity is considered to be associated with thrombosis development. However, the effects of L-arginine on blood fluidity after exercise remain unclear.
OBJECTIVE:
We investigated the mechanisms of impaired blood fluidity after high-intensity exercise, and examined whether L-arginine improves exercise-induced blood fluidity impairment in vitro.
METHODS:
Ten healthy male participants performed 15 minutes of ergometer exercise at 70% of their peak oxygen uptake levels. Blood samples were obtained before and after exercise. L-arginine and NG-monomethyl-L-arginine acetate (L-NMMA)—a nitric oxide (NO) synthase inhibitor—were added to the post-exercise blood samples. Using Kikuchi’s microchannel method, we measured the blood passage time, percentage of obstructed microchannels, and the number of adherent white blood cells (WBCs) on the microchannel terrace.
RESULTS:
Exercise increased the hematocrit levels. The blood passage times, percentage of obstructed microchannels, and the number of adherent WBCs on the microchannel terrace increased after exercise; however, they decreased in a dose-dependent manner after the addition of L-arginine. L-NMMA inhibited the L-arginine-induced decrease in blood passage time.
CONCLUSIONS:
High-intensity exercise impairs blood fluidity by inducing hemoconcentration along with increasing platelet aggregation and WBC adhesion. The L-arginine–NO pathway improves blood fluidity impairment after high-intensity exercise in vitro.
1Introduction
Exercise is important for promoting health. Regular exercise prevents the progression of atherosclerosis, and reduces the incidence of coronary heart disease and venous thromboembolism [1–3]. It also reduces all-cause and cardiovascular mortality [4]. Furthermore, long-term exercise training is reported to improve blood fluidity by increasing red blood cell (RBC) deformability and lowering hematocrit levels via an increase in plasma volume [5, 6]. However, a single bout of vigorous exercise is reported to increase the risk of acute myocardial infarction, especially in habitually sedentary individuals [2, 7]. Rupture of an atherosclerotic plaque caused by elevated vascular shear stress and decrease in blood fluidity due to strenuous exercise may contribute to the development of thrombosis [7–9]. Gaudard et al. reported a markedly reduced post-exercise blood fluidity in a 50-year-old marathon runner, who developed a central retinal vein thrombosis after a marathon, compared to that of healthy 21- to 36-year-old adults [10]. Increased levels of hematocrit caused due to fluid shift, increased circulating blood cells, and water loss cause blood fluidity impairment after exercise [5]. We previously reported that high-intensity exercise impairs blood fluidity by changing the cloggy status of the blood [9]. Although this status was considered to be caused due to hematopoietic cells, including platelets and white blood cells (WBCs), the mechanism remains unclear.
No report has shown an improvement in impaired blood fluidity after exercise so far; we hypothesized that L-arginine improves blood fluidity after exercise. L-arginine is the physiological precursor of nitric oxide (NO), which has been reported to be an inhibitor of platelet aggregation and WBC adhesion, as well as a vasodilator [11]. However, the effects of L-arginine on blood fluidity after exercise have not been clarified.
This study aimed to investigate the mechanisms of blood fluidity impairment after high-intensity exercise. Additionally, we investigated whether L-arginine can improve blood fluidity impairment after high-intensity exercise in vitro.
2Materials and methods
2.1Subjects
Ten healthy male volunteers (age, 24.3±2.1 years; body mass index, 21.8±2.9 kg/m2) were included in this study. Smokers, patients with internal disorders, and those who use medicine or dietary supplements were excluded from the study. A sample size of seven was required to detect the effect of L-arginine on post-exercise blood passage time and to obtain the required α significance level of 0.05, statistical power of 0.95, and an effect size of 0.69 calculated during our preliminary study. All participants were instructed to avoid any kind of vigorous exercise 24 hours before the experiments, and to avoid eating and drinking 2 hours before the experiments. The participants passed urine and then drank 200 mL of water 20 minutes before the experiments.
The Ethical Committee for Epidemiology of Hiroshima University approved this study (approval number: E-487-1). Written informed consent was obtained from all participants after a full explanation of the purpose, contents, and risks of the study.
2.2Maximal exercise test
All participants performed an incremental maximal exercise test using a bicycle ergometer (Ergometer 232CXL; Combi Corporation, Tokyo, Japan) to determine the peak oxygen uptake (peak
2.3Exercise protocol
The participants performed ergometric exercise for 15 minutes using a bicycle ergometer (STB-2400; Nihon Kohden Co., Ltd., Tokyo, Japan) at least 2 days after the maximal exercise test. The bicycle workload was set at a power output equivalent to 70% of the peak
2.4Blood collection
Blood samples were collected from the antecubital vein (with the participant seated) before and immediately after the exercise. The blood obtained for hematological measurements (2 mL) was immediately transferred to tubes containing 1.5 mg/mL ethylenediaminetetraacetic acid-2Na (Terumo Corporation, Tokyo, Japan) and analyzed using an automated hematology analyzer (XN-9000; Sysmex Corporation, Hyogo, Japan). The blood samples used for the hemorheological measurements and amino acids analysis contained 5% 1000 IU/mL heparin solution (Mochida Pharmaceutical Co., Ltd., Tokyo, Japan) and 95% whole blood.
2.5Preparation of RBC suspension
The RBC suspension was prepared using a previously reported method [12]. Briefly, the blood was layered on a lymphocyte separation solution (d = 1.119) (Nacalai Tesque, Inc., Kyoto, Japan) and centrifuged at 400×g for 30 minutes. After centrifugation, the obtained RBCs were washed with phosphate-buffered saline (PBS) (Wako Pure Chemical Industries, Ltd., Osaka, Japan) containing 0.1% albumin (Sigma-Aldrich, Co., St. Louis, MO, USA) by centrifugation at 300×g for 10 minutes. We suspended the RBCs in PBS containing 0.1% albumin and prepared RBC suspensions with a hematocrit level of 50%.
2.6Addition of L-arginine to blood samples
We added 20μL of normal saline (0.9% NaCl solution; Otsuka Pharmaceutical Factory, Inc., Tokushima, Japan) containing L-arginine (L-(+)-arginine hydrochloride; Tokyo Chemical Industry Co., Ltd., Tokyo, Japan) to a 980-μL post-exercise blood sample and RBC suspension. The final concentrations of L-arginine were 0 mM, 0.01 mM, 0.1 mM, 1 mM, and 10 mM. Additionally, we added 20μL of normal saline to the 980-μL pre-exercise blood sample and RBC suspension.
2.7Determination of the blood and RBC suspension passage times
The passage times of the blood samples and RBC suspensions were defined as the time required for 100μL of the sample to pass through the microchannel array (width, 7μm; length, 30μm; depth, 4.5μm; 8736 in parallel) fabricated on a single crystal silicon substrate within the microchannel array flow analyzer (MC-FAN; Hitachi Haramachi Electronics Co., Ltd., Japan), as previously described [9, 12]. After the addition of L-arginine, blood samples and RBC suspensions were kept at room temperature (24°C) for 30 minutes before the passage times were measured.
2.8Determination of microchannel obstruction and WBC adhesion
We analyzed the percentage of microchannels obstructed by aggregated platelets and adherent WBCs, and we counted the number of adherent WBCs on the microchannel terrace using a previously reported method [12]. The blood flow through the microchannels was recorded and stored on a digital recorder (BDZ-ZW1700; Sony Marketing Inc., Tokyo, Japan) for offline analysis. Five micrographs with a final blood volume of 20μL were captured and analyzed by an investigator who was blinded to the participants’ information.
2.9NO synthase inhibition study
To investigate the effects of L-arginine on blood fluidity in vitro, NG-monomethyl-L-arginine acetate (L-NMMA; Dojindo Molecular Technologies, Inc., Kumamoto, Japan), an NO synthase inhibitor, was added to the post-exercise blood samples and RBC suspensions 5 minutes before the addition of L-arginine. Subsequently, 10μL of normal saline containing L-NMMA and 10μL of normal saline containing L-arginine were added to the 980μL post-exercise blood samples and RBC suspensions. The final concentrations of L-arginine were 0 mM and 1 mM. The final concentrations of L-NMMA were 0 mM, 0.1 mM, and 0.5 mM. The passage times of blood and RBC suspensions were measured 30 minutes after the addition of L-arginine.
2.10Measurement of plasma L-arginine concentrations
We performed an amino acid analysis to investigate whether the plasma L-arginine concentration decreased after 30 minutes of incubation at room temperature (24°C). Then, 10μL of normal saline containing L-NMMA and 10μL of normal saline containing L-arginine were added to the 980-μL post-exercise blood samples. The final concentrations of L-NMMA and L-arginine in whole blood were 0.5 mM and 1 mM, respectively. L-NMMA was added to the post-exercise blood samples 5 minutes before the addition of L-arginine. The post-exercise blood samples immediately after and 30 minutes after the addition of L-arginine and L-NMMA were centrifuged at 3,000×g for 10 minutes at 4°C. The plasma L-arginine concentration was determined using an amino acid analyzer (L-8900; Hitachi High-Tech Corporation, Tokyo, Japan) [13].
2.11Statistical analysis
All statistical data are expressed as means±standard deviation. The passage times of blood and RBC suspensions were measured twice, and the means were analyzed. Blood cell counts and body weights before and after exercise were compared using paired t-tests. The passage times of blood and RBC suspensions, the percentage of obstructed microchannels, the number of adherent WBCs on the microchannel terrace, and the plasma L-arginine concentrations were analyzed using Dunnett’s test and one-way analysis of variance. Statistical analysis was performed using IBM SPSS Statistics for Windows version 27.0 (IBM Japan Ltd., Tokyo, Japan); p < 0.05 was considered statistically significant. The necessary sample size was estimated by G*power software, version 3.1 (Franz Faul, University of Kiel, Kiel, Germany) [14].
3Results
3.1Physical characteristics
The peak
Table 1
Blood cell counts and body weight before and after exercise
Before exercise | After exercise | p-value | |
White blood cells (×103/μL) | 5.34±0.94 | 8.25±1.45 | < 0.001 |
Red blood cells (×106/μL) | 5.18±0.50 | 5.46±0.50 | < 0.001 |
Platelets (×105/μL) | 2.21±0.29 | 2.56±0.33 | < 0.001 |
Hematocrit level (%) | 45.7±3.76 | 48.5±3.99 | < 0.001 |
Body weight (kg) | 63.7±10.6 | 63.4±10.5 | < 0.001 |
Values are expressed as means±standard deviation.
3.2Effects of exercise and L-arginine on blood passage time
The blood passage time was significantly increased after exercise (before exercise versus after exercise without L-arginine treatment, p < 0.001) (Fig. 1A). The blood passage time after exercise decreased in an L-arginine dose-dependent manner. Post-exercise blood passage times were significantly lower with 0.1-mM, 1-mM, and 10-mM L-arginine treatments than that without L-arginine treatment (p < 0.05, p < 0.01, and p < 0.001, respectively).
Fig. 1
Blood passage time, microchannel obstruction, and white blood cell adhesion. (A) Blood passage time, (B) percentage of obstructed microchannels, and (C) number of adherent white blood cells (WBCs) on the microchannel terrace after the addition of L-arginine in samples collected before and after exercise. *p < 0.05, **p < 0.01, and ***p < 0.001 vs. before exercise; †p < 0.05, ††p < 0.01, †††p < 0.001, and n.s., not significant; LPF: low power field; Before: before exercise; After: after exercise.
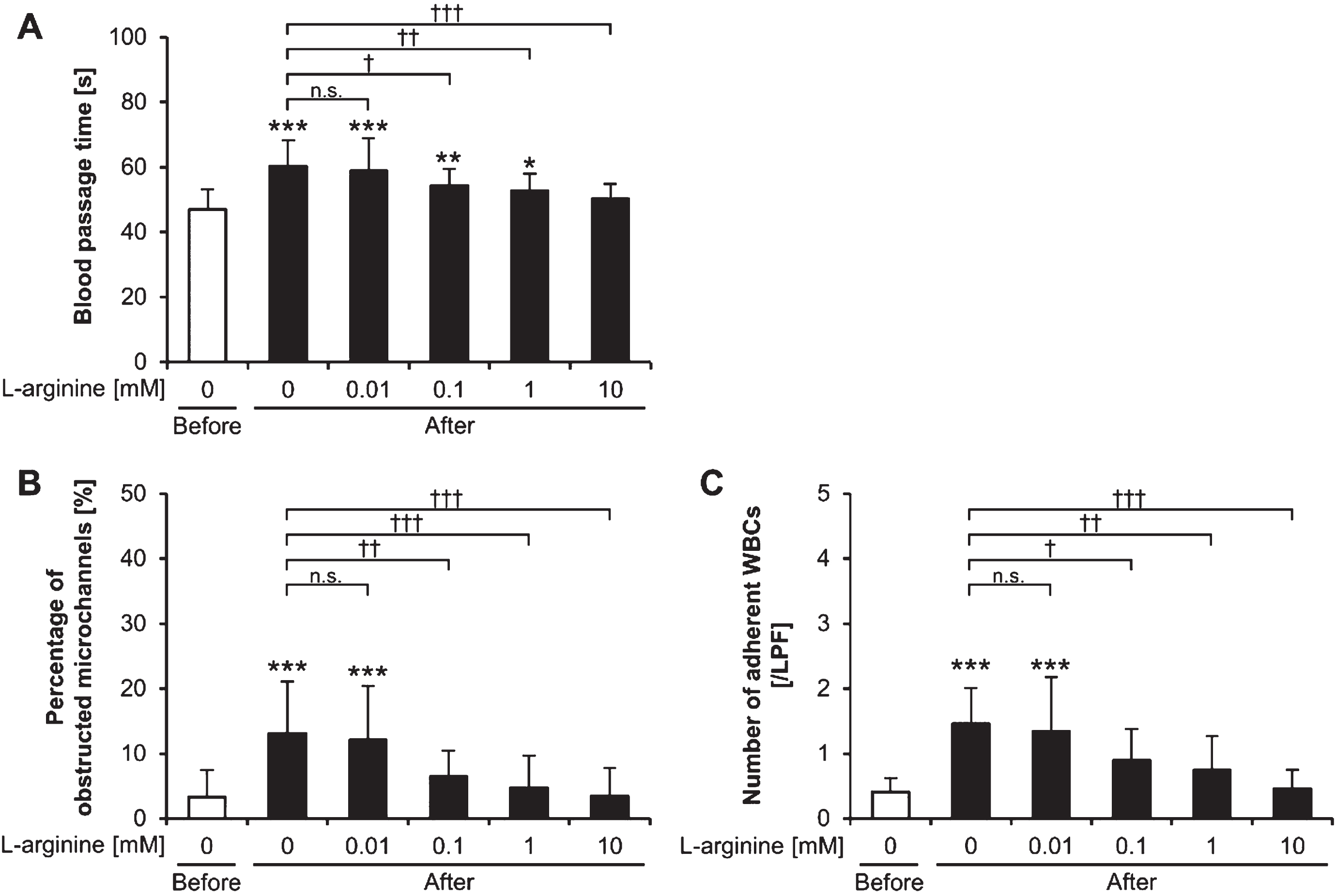
3.3Effects of exercise and L-arginine on microchannel obstruction and WBC adhesion
The percentage of obstructed microchannels was significantly increased after exercise (before exercise versus after exercise without L-arginine treatment, p < 0.001) (Fig. 1B). Microchannel obstruction after exercise decreased upon addition of L-arginine in a concentration-dependent manner. The percentages of obstructed microchannels after exercise with the 0.1-, 1-, and 10-mM L-arginine treatments were significantly lower than that without L-arginine treatment (p < 0.01, p < 0.001, and p < 0.001, respectively).
The number of adherent WBCs on the microchannel terrace significantly increased after exercise (before exercise versus after exercise without L-arginine treatment; p < 0.001) (Fig. 1C). Adherent WBCs after exercise decreased with addition of L-arginine in a dose-dependent manner. The numbers of adherent WBCs on the microchannel terrace after exercise with the 0.1-, 1-, and 10 mM L-arginine treatments were significantly lower than that without L-arginine treatment (p < 0.05, p < 0.01, and p < 0.001, respectively).
Figure 2 shows three micrographs of blood flow. Thirty-three percent (4 of 12) of the microchannels were obstructed by aggregated platelets and adherent WBCs; two adherent WBCs were observed on the microchannel terrace in post-exercise blood samples without L-arginine treatment. However, no obstructed microchannels and one adherent WBC were observed in samples treated with 1 mM of L-arginine.
Fig. 2
Micrographs of blood flow. Flow channel images (A) before exercise with 0 mM of L-arginine, (B) after exercise without L-arginine, and (C) after exercise with 1 mM of L-arginine. Arrow indicates aggregated platelets; arrowhead indicates adherent white blood cells.
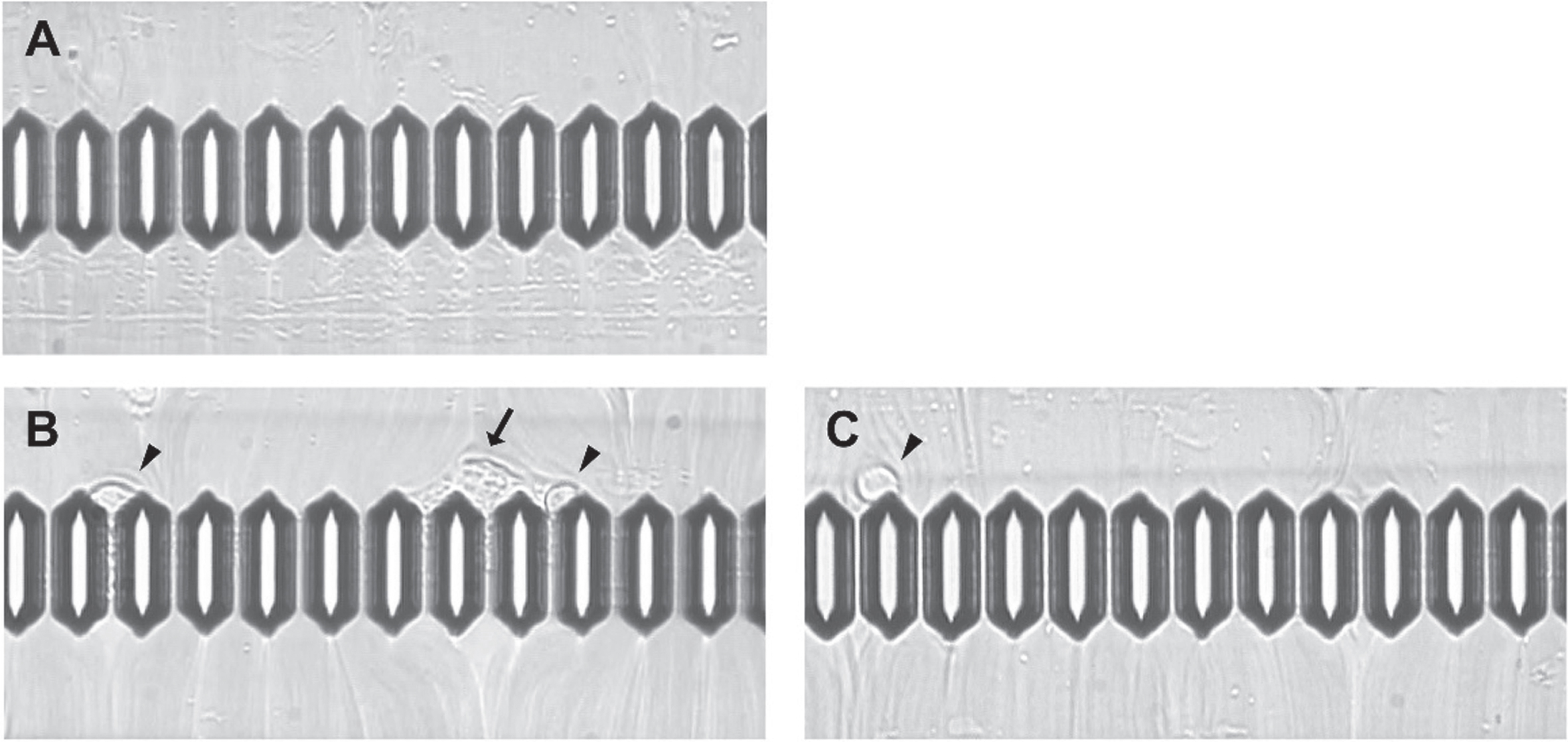
3.4Effects of L-arginine and NO synthase inhibitor on blood passage time after exercise
We used L-NMMA, an NO synthase inhibitor, to clarify the involvement of NO in the L-arginine-induced decrease in blood passage time after exercise. The post-exercise blood passage time with 1 mM of L-arginine increased in an L-NMMA dose-dependent manner (Fig. 3A). The post-exercise blood passage time with the 1 mM L-arginine plus 0.5 mM L-NMMA treatment was significantly longer than that with the 1 mM L-arginine without L-NMMA treatment (p < 0.05). L-NMMA treatment alone had no significant effect on the post-exercise blood passage times (p≥0.05).
Fig. 3
Blood passage time, microchannel obstruction, and white blood cell adhesion. (A) Blood passage time, (B) percentage of obstructed microchannels, and (C) number of adherent white blood cells (WBCs) on the microchannel terrace after the addition of L-arginine and L-NMMA in samples collected before and after exercise. ***p < 0.001. †p < 0.05. †††p < 0.001. n.s., not significant; LPF: low power field; L-NMMA, NG-monomethyl-L-arginine acetate; Before: before exercise; After: after exercise.
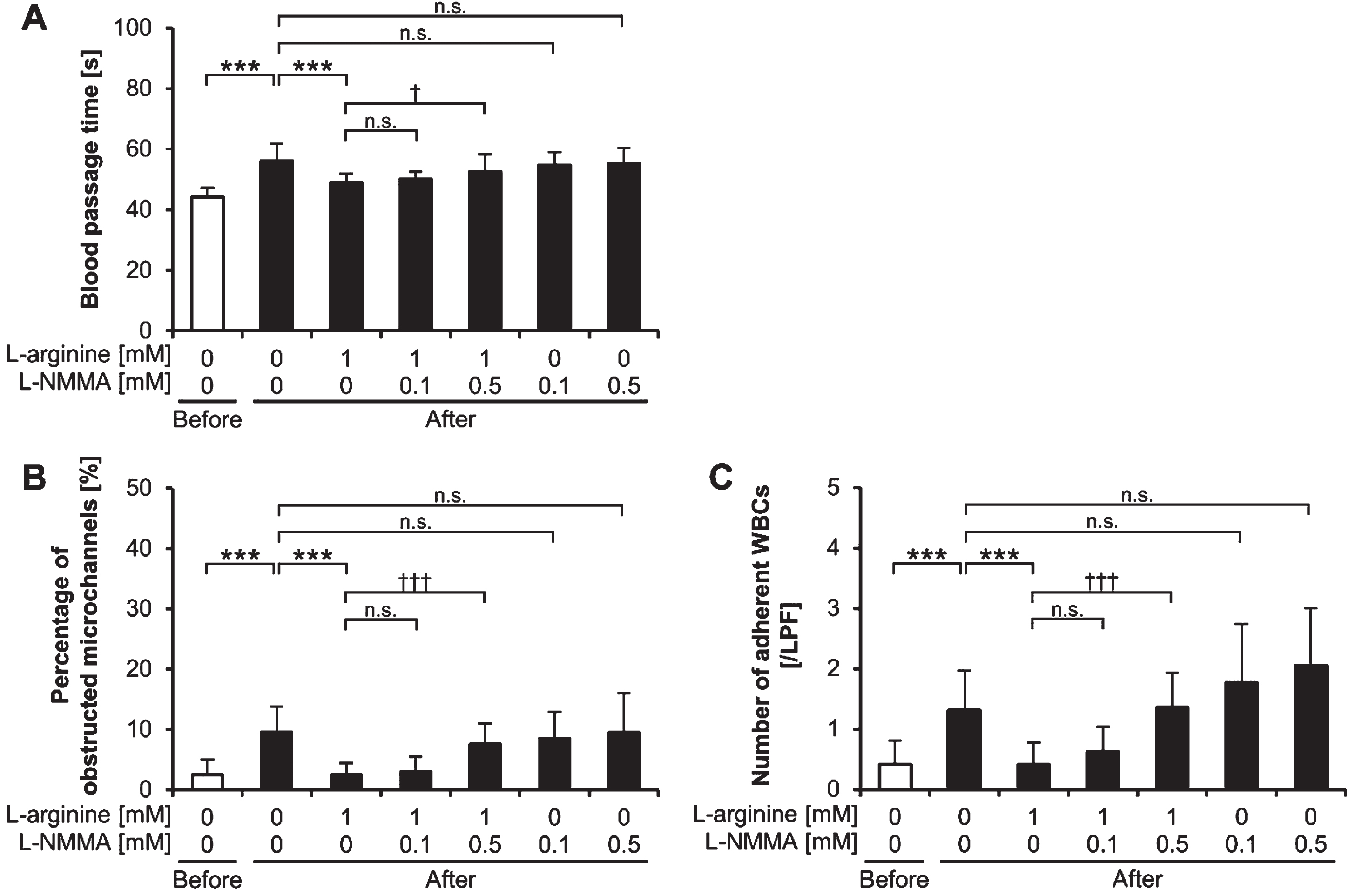
3.5Effects of L-arginine and NO synthase inhibitor on microchannel obstruction and WBC adhesion
The percentage of obstructed microchannels and the number of adherent WBCs on the microchannel terrace for post-exercise samples treated with 1 mM of L-arginine increased in an L-NMMA concentration-dependent manner (Fig. 3B, 3C). The percentage of obstructed microchannels and the number of adherent WBCs on the microchannel terrace for post-exercise samples with the 1 mM L-arginine plus 0.5 mM L-NMMA treatment were significantly higher than those of the 1 mM L-arginine without L-NMMA treatment (p < 0.001 and p < 0.001, respectively). L-NMMA treatment alone had no significant effect on the percentage of obstructed microchannels and the number of adherent WBCs on the microchannel terrace in post-exercise samples (p≥0.05).
3.6Changes in the plasma L-arginine concentration
The plasma L-arginine concentration of post-exercise blood samples before adding L-arginine was 0.09±0.02 mM. Figure 4 shows the plasma L-arginine concentration of post-exercise blood samples immediately after and 30 minutes after the addition of L-arginine and L-NMMA. The plasma L-arginine concentrations of post-exercise blood samples 30 minutes after adding 1 mM of L-arginine and without L-NMMA were significantly lower than those immediately after adding 1 mM of L-arginine and without L-NMMA (p < 0.001). The plasma L-arginine concentrations of post-exercise blood samples 30 minutes after adding 1 mM of L-arginine and 0.5 mM of L-NMMA were significantly higher than those 30 minutes after adding 1 mM of L-arginine and without L-NMMA (p < 0.01).
Fig. 4
Plasma L-arginine concentrations. Plasma L-arginine concentrations of post-exercise blood samples immediately after and 30 minutes after the addition of L-arginine and L-NMMA. **p < 0.01. ***p < 0.001. L-NMMA: NG-monomethyl-L-arginine acetate; Immediately after: immediately after L-arginine addition; 30 minutes after: 30 minutes after L-arginine addition.
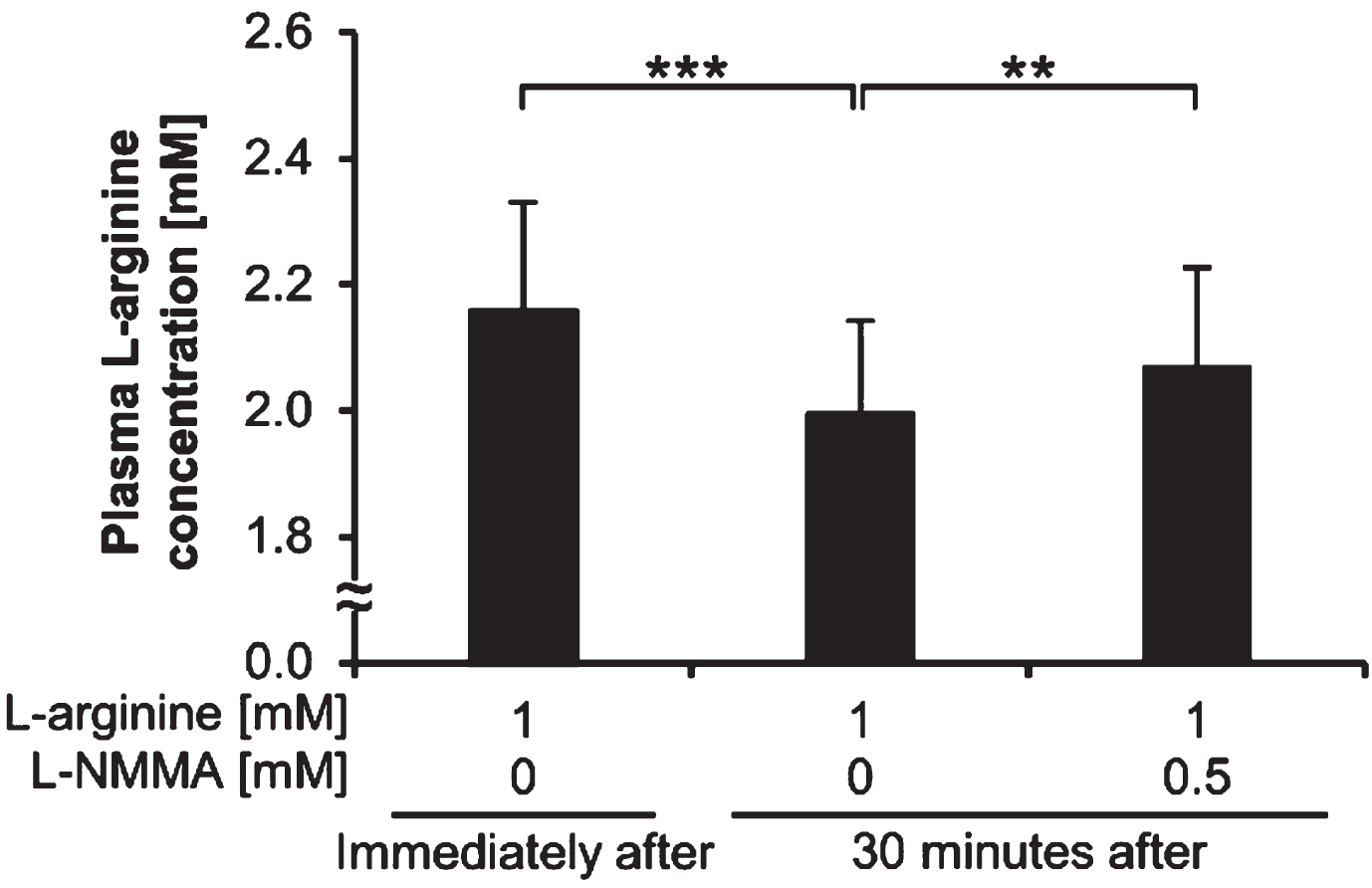
3.7Effects of exercise, L-arginine, and NO synthase inhibitor on the RBC suspension passage time
There were no significant effects of exercise, L-arginine, and NO synthase inhibitor on the RBC suspension passage time (p≥0.05) (Fig. 5A, 5B).
Fig. 5
Passage times of red blood cell suspensions. Passage times of red blood cell (RBC) suspensions (A) treated with L-arginine and (B) treated with L-arginine and L-NMMA, before and after exercise. L-NMMA: NG-monomethyl-L-arginine acetate; Before: before exercise; After: after exercise.
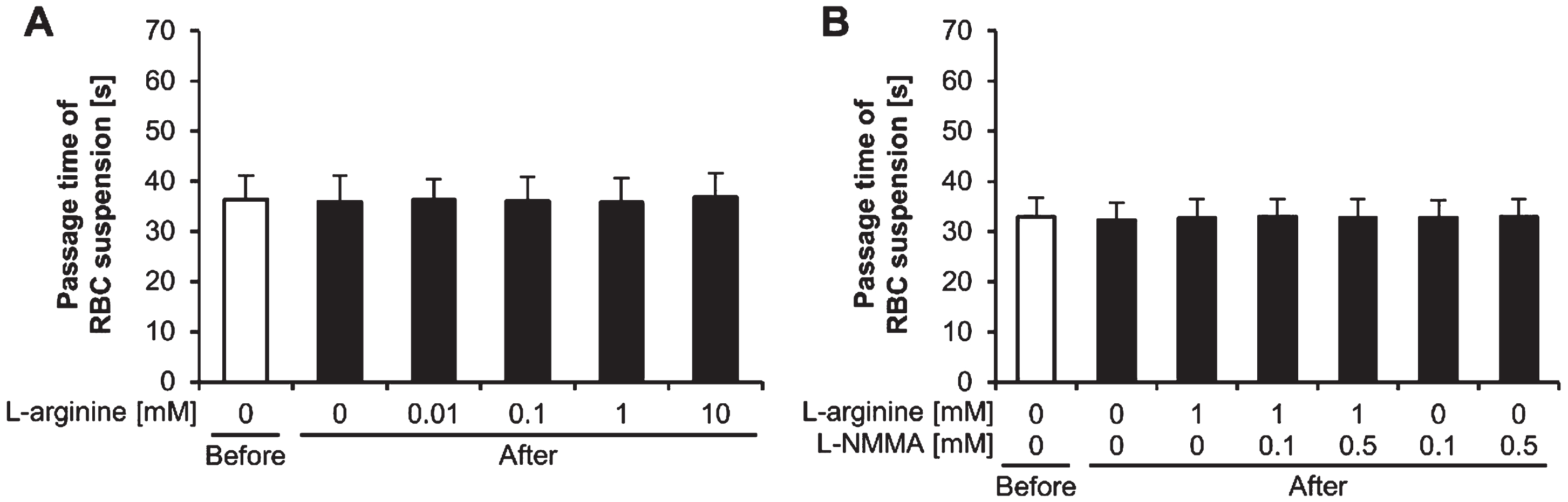
4Discussion
This study investigated the mechanism of blood fluidity impairment after high-intensity exercise, and whether L-arginine can improve blood fluidity impaired after exercise in vitro. The blood passage time, percentage of obstructed microchannels, and number of adherent WBCs on the microchannel terrace increased after exercise; however, they decreased in a dose-dependent manner after the addition of L-arginine. The hematocrit levels increased after exercise. The RBC suspension passage time was unaffected by exercise and L-arginine addition. High-intensity exercise impairs the blood fluidity not only by inducing hemoconcentration, but also by increasing platelet aggregation and WBC adhesion. However, L-arginine improves blood fluidity after exercise by inhibiting platelet aggregation and WBC adhesion.
This is the first study to show that platelet aggregation and WBC adhesion are involved in the impairment of blood fluidity after exercise via evaluation using a capillary model. We have previously reported the cloggy status of the blood observed after exercise using MC-FAN video images [9]. In the present study, the percentage of obstructed microchannels and the number of adherent WBCs on the microchannel terrace were increased after exercise, suggesting that platelet aggregation and WBC adhesion were responsible for the increase in the blood passage time. Activated platelets obstruct the microchannels by forming aggregates [15, 16], and activated WBCs may contribute to microchannel obstruction by adhering to the silicon substrate of the MC-FAN via plasma proteins. The silicon substrate of the MC-FAN is covered with silicon dioxide (SiO2), which absorbs plasma proteins such as albumin, fibrinogen, and fibronectin [17, 18]. These plasma proteins mediate cell adhesion by binding to integrins [18, 19]. Moreover, in the present study, high hematocrit levels after exercise might also have contributed to the impairment of blood fluidity. Previous studies reported that exercise causes a fluid shift to extra-vascular spaces, an increase in the number of circulating blood cells, entrapment of water in muscle cells, and loss of water with sweating, which increase the hematocrit levels and impair blood fluidity [5, 9, 20, 21]. Tripette et al. reported that water loss (2.0% body weight) after 40 minutes of ergometer exercise at 55% peak power output did not affect hematocrit levels and blood viscosity [22]. In the present study, because of the low amount of sweating (0.4% body weight), the effect of water loss on hematocrit levels might be small; rather, fluid shift, increased blood cell counts, and water entrapment by muscle cells might have contributed to the increase in hematocrit levels.
RBCs might not be involved in the impairment of blood fluidity after exercise, as evaluated by the MC-FAN. In the present study, RBC suspension passage time did not change after exercise, which suggests that platelets and WBCs, rather than RBCs, contributed to the increase in the blood passage time after exercise. The reasons that exercise did not affect the RBC suspension passage times are unknown. The type, intensity, and duration of exercise might affect the rheological properties of RBCs [23–26]. Exercise decreases RBC deformability because of several factors such as lactate accumulation and oxidative stress [26, 27]. Şentürk et al. demonstrated that exhaustive treadmill exercise caused lipid peroxidation of RBCs and decreased RBC filterability through 5-μm pores during a cell transit time analysis of sedentary rats [28]. However, Neuhaus et al. reported that marathon running did not affect RBC suspension filterability through 5-μm pores of a nucleopore filter during an analysis involving healthy marathon runners [29]. McNamee et al. reported that the RBC rigidity and capillary transit velocity of RBCs were not associated with each other [30]. Nevertheless, the present capillary model involving the MC-FAN might not be able to evaluate the changes in RBC mechanics.
This is the first report to demonstrate the possibility of improving blood fluidity impairments after exercise in vitro. L-arginine is the physiological precursor of NO, produced by the action of NO synthase on L-arginine [11]. NO prevents platelet aggregation by inhibiting the increase in platelet calcium ion levels [31]. NO also suppresses WBC adhesion by inactivating integrins through the S-nitrosylation of cytoplasmic actin [19]. We hypothesized that NO synthesized from the action of NO synthase on exogenous L-arginine inhibits platelet aggregation and WBC adhesion, thereby improving blood fluidity after exercise. In the present study, L-arginine addition decreased platelet aggregation and WBC adhesion and improved blood fluidity after exercise. These actions of L-arginine were inhibited by L-NMMA, an NO synthase inhibitor. Furthermore, L-NMMA addition prevented a decrease in plasma L-arginine concentrations. The L-arginine–NO pathway may be able to improve blood fluidity impaired after exercise.
This study had several limitations. First, the experiments were performed at 24°C, not at 37°C. Our experiments should have been performed at 37°C, because blood cell function and enzyme activity are temperature dependent. Williamson et al. reported that RBC elongation at a shear stress of 6 dynes/cm2 was greater at 37°C than at 25°C [32]. In addition, our preliminary study found that NO synthase activity was higher at 37°C than at 24°C. Furthermore, sample temperature should not be changed during the experiments. Our preliminary study revealed that a decrease in sample temperature from 37°C to 24°C markedly increased platelet aggregation. All our experiments were performed at 24°C because our device did not have a heater, and we had no way of maintaining the temperature at 37°C during our experiments. Second, we failed to address the increase in shear rate due to exercise and vascular endothelial factors. A high shear rate after exercise compensates for the increase in blood viscosity [27]. In addition, an increase in blood viscosity caused by exercise may decrease vascular resistance, because increased wall shear stress may stimulate endothelium-dependent NO production [33]. Third, our study did not consider the various in vivo effects exerted on blood after exercise. Blood rheology after exercise is altered by physiological and biochemical effects such as fluid shifts and lactate and oxidative stresses [5, 21, 27, 34]. However, the rheological data of collected blood samples may be static. Our preliminary study found that post-exercise blood passage time remained unchanged with time after blood collection. In spite of these limitations, the present study might contribute to the development of a new strategy to decrease the risk of thrombosis induced by exercise. A clinical study investigating the effects of oral L-arginine supplementation on blood fluidity after exercise is necessary.
In conclusion, high-intensity exercise impairs blood fluidity not only by inducing hemoconcentration but also by increasing platelet aggregation and WBC adhesion. However, the L-arginine–NO pathway may be able to improve blood fluidity after exercise by inhibiting platelet aggregation and WBC adhesion.
Acknowledgments
This work was supported by the Fundamental Budget for Education and Research and the Ohkagakuen Special Research Expense. We would like to thank Editage (https://www.editage.jp) for English language editing.
References
[1] | Thompson PD , Buchner D , Pina IL , Balady GJ , Williams MA , Marcus BH , et al. Exercise and physical activity in the prevention and treatment of atherosclerotic cardiovascular disease: a statement from the council on clinical cardiology (subcommittee on exercise, rehabilitation, and prevention) and the council on nutrition, physical activity, and metabolism (subcommittee on physical activity), Circulation. (2003) ;107: (24):3109–16. doi:10.1161/01.CIR.0000075572.40158.77. |
[2] | Franklin BA , Thompson PD , Al-Zaiti SS , Albert CM , Hivert M-F , Levine BD , et al. Exercise-related acute cardiovascular events and potential deleterious adaptations following long-term exercise training: placing the risks into perspective-an update: a scientific statement from the american heart association, Circulation. (2020) ;141: (13):e705–36.doi:10.1161/CIR.0000000000000749. |
[3] | Kunutsor SK , Mäkikallio TH , Seidu S , de Araújo CGS , Dey RS , Blom AW , et al. Physical activity and risk of venous thromboembolism: systematic review and meta-analysis of prospective cohort studies, Eur J Epidemiol. (2020) ;35: (5):431–42. doi:10.1007/s10654-019-00579-2. |
[4] | Kraus WE , Powell KE , Haskell WL , Janz KF , Campbell WW , Jakicic JM , et al. Physical activity, all-cause and cardiovascular mortality, and cardiovascular disease, Med Sci Sports Exerc. (2019) ;51: (6):1270–81. doi:10.1249/MSS.0000000000001939. |
[5] | Brun JF , Khaled S , Raynaud E , Bouix D , Micallef JP , Orsetti A . The triphasic effects of exercise on blood rheology: which relevance to physiology and pathophysiology? Clin Hemorheol Microcirc. (1998) ;19: (2):89–104. |
[6] | Kilic-Toprak E , Ardic F , Erken G , Unver-Kocak F , Kucukatay V , Bor-Kucukatay M . Hemorheological responses to progressive resistance exercise training in healthy young males, Med Sci Monit. (2012) ;18: (6):CR351-60. doi:10.12659/MSM.882878. |
[7] | Olsen LN , Fischer M , Evans PA , Gliemann L , Hellsten Y . Does exercise influence the susceptibility to arterial thrombosis? An integrative perspective, Front Physiol. (2021) ;2: :636027. doi:10.3389/fphys.2021.636027. |
[8] | Thompson PD , Franklin BA , Balady GJ , Blair SN , Corrado D , Estes NAM 3rd , et al. Exercise and acute cardiovascular events placing the risks into perspective: a scientific statement from the american heart association council on nutrition, physical activity, and metabolism and the council on clinical cardiology, Circulation. (2007) ;115: (17):2358–68. doi:10.1161/CIRCULATIONAHA.107.181485. |
[9] | Kimura T , Hamada H , Taito S , Takahashi M , Sekikawa K .The effect of exercise on blood fluidity: use of the capillary model to assess the clogginess of blood, Clin Hemorheol Microcirc. (2016) ;61: (4):559–69. doi:10.3233/CH-141893. |
[10] | Gaudard A , Varlet-Marie E , Monnier JF , Janbon C , Quéré I , Bressolle F , et al. Exercise-induced central retinal vein thrombosis: possible involvement of hemorheological disturbances, A case report. Clin Hemorheol Microcirc. (2002) ;27: (2):115–22. |
[11] | Moncada S , Higgs A . The L-arginine-nitric oxide pathway, N Engl J Med. (1993) ;329: (27):2002–12. doi:10.1056/NEJM199312303292706. |
[12] | Otoyama I , Hamada H , Kimura T , Namba H , Sekikawa K , Kamikawa N , et al. L-cysteine improves blood fluidity impaired by acetaldehyde: in vitro evaluation, PLoS One. (2019) ;14: (3):e0214585. doi:10.1371/journal.pone.0214585. |
[13] | Watts ST . Advancements in amino acid analysis: introduction of the new Hitachi L-Amino Acid Analyzer, LC GC NORTH Am. (2006) ;17: :20. |
[14] | Faul F , Erdfelder E , Lang A-G , Buchner A . G*Power a flexible statistical power analysis program for the social, behavioral, and biomedical sciences, Behav Res Methods. (2007) ;39: (2):175–91. doi:10.3758/bf03193146. |
[15] | Kikuchi Y . Effect of leukocytes and platelets on blood flow through a parallel array of microchannels: micro- and macroflow relation and rheological measures of leukocyte and platelet activities, Microvasc Res. (1995) ;50: (2):288–300. doi:10.1006/mvre.1995.1059. |
[16] | Kamada H , Okamoto T , Hayashi T , Suzuki K . An in vitro method for screening anti-platelet agents using a microchannel array flow analyzer, Biorheology. (2010) ;47: (2):153–61. doi:10.3233/BIR-2010-0566. |
[17] | Ruh H , Kühl B , Brenner-Weiss G , Hopf C , Diabaté S , Weiss C . Identification of serum proteins bound to industrial nanomaterials, Toxicol Lett. (2012) ;208: (1):41–50. doi:10.1016/j.toxlet.2011.09.009. |
[18] | Delivopoulos E , Ouberai MM , Coffey PD , Swann MJ , Shakesheff KM , Welland ME . Serum protein layers on parylene-C and silicon oxide: effect on cell adhesion, Colloids Surf B Biointerfaces. (2015) ;126: :169–77. doi:10.1016/j.colsurfb.2014.12.020. |
[19] | Bhopale VM , Yang M , Yu K , Thom SR . Factors associated with nitric oxide-mediated β2 integrin inhibition of neutrophils, J Biol Chem. (2015) ;290: (28):17474–84. doi:10.1074/jbc.M115.651620. |
[20] | Ikeda N , Yasu T , Tsuboi K , Sugawara Y , Kubo N , Umemoto T , et al. Effects of submaximal exercise on blood rheology and sympathetic nerve activity, Circ J. (2010) ;74: (4):730–4. doi:10.1253/circj.cj-09-0758. |
[21] | Nader E , Skinner S , Romana M , Fort R , Lemonne N , Guillot N , et al. Blood rheology: key parameters, impact on blood flow, role in sickle cell disease and effects of exercise, Front Physiol. (2019) ;10: :1329. doi:10.3389/fphys.2019.01329. |
[22] | Tripette J , Loko G , Samb A , Gogh BD , Sewade E , Seck D , et al. Effects of hydration and dehydration on blood rheology in sickle cell trait carriers during exercise, Am J Physiol Heart Circ Physiol. (2010) ;299: (3):H908–14. doi:10.1152/ajpheart.00298.2010. |
[23] | Simmonds MJ , Connes P , Sabapathy S . Exercise-induced blood lactate increase does not change red blood cell deformability in cyclists, Lucia A, editor. PLoS One. (2013) ;8: (8):e71219. doi:10.1371/journal.pone.0071219. |
[24] | Romagnoli M , Alis R , Martinez-Bello V , Sanchis-Gomar F , Aranda R , Gómez-Cabrera M-C . Blood rheology effect of submaximal exercise on young subjects, Clin Hemorheol Microcirc. (2014) ;56: (2):111–7. doi:10.3233/CH-121657. |
[25] | Tomschi F , Bizjak D , Bloch W , Latsch J , Predel HG , Grau M . Deformability of different red blood cell populations and viscosity of differently trained young men in response to intensive and moderate running, Clin Hemorheol Microcirc. (2018) ;69: (4):503–14. doi:10.3233/CH-189202. |
[26] | Nemkov T , Skinner SC , Nader E , Stefanoni D , Robert M , Cendali F , et al. Acute cycling exercise induces changes in red blood cell deformability and membrane lipid remodeling, Int J Mol Sci. (2021) ;22: (2):896. doi:10.3390/ijms22020896. |
[27] | Connes P , Simmonds MJ , Brun J-F , Baskurt OK . Exercise hemorheology: classical data, recent findings and unresolved issues, Clin Hemorheol Microcirc. (2013) ;53: (1–2):187–99. doi:10.3233/CH-2012-1643. |
[28] | Sentürk ÜK , Gündüz F , Kuru O , Aktekin MR , Kipmen D , YalÇin Ö , et al. Exercise-induced oxidative stress affects erythrocytes in sedentary rats but not exercise-trained rats, J Appl Physiol. (2001) ;91: (5):1999–2004. doi:10.1152/jappl.2001.91.5.1999. |
[29] | Neuhaus D , Behn C , Gaehtgens P . Haemorheology and exercise: intrinsic flow properties of blood in marathon running, Int J Sports Med. (1992) ;13: (7):506–11. doi:10.1055/s-2007-1021307. |
[30] | McNamee AP , Tansley GD , Simmonds MJ . Sublethal mechanical shear stress increases the elastic shear modulus of red blood cells but does not change capillary transit velocity, Microcirculation . (2020) ;27: (8):e12652. doi:10.1111/micc.12652. |
[31] | Smolenski A . Novel roles of cAMP/cGMP-dependent signaling in platelets, J Thromb Haemost. (2012) ;10: (2):167–76. doi:10.1111/j.1538-7836.2011.04576.x. |
[32] | Williamson JR , Shanahan MO , Hochmuth RM . The influence of temperature on red cell deformability, Blood. (1975) ;46: (4):611–24. |
[33] | Connes P , Pichon A , Hardy-Dessources M-D , Waltz X , Lamarre Y , Simmonds MJ , et al. Blood viscosity and hemodynamics during exercise, Clin Hemorheol Microcirc. (2012) ;51: (2):101–9. doi:10.3233/CH-2011-1515. |
[34] | Yalcin O , Erman A , Muratli S , Bor-Kucukatay M , Baskurt OK . Time course of hemorheological alterations after heavy anaerobic exercise in untrained human subjects, J Appl Physiol. (2003) ;94: (3):997–1002. doi:10.1152/japplphysiol.00368.2002. |