Chronic corticosterone administration reduces dendritic complexity in mature, but not young granule cells in the rat dentate gyrus
Abstract
Background: Our previous work has shown that exposure to the stress hormone corticosterone (40 mg/kg CORT) for two weeks induces dendritic atrophy of pyramidal neurons in the hippocampal CA3 region and behavioral deficits. However, it is unclear whether this treatment also affects the dentate gyrus (DG), a subregion of the hippocampus comprising a heterogeneous population of young and mature neurons.
Objective: We examined the effect of CORT treatment on the dendritic complexity of mature and young granule cells in the DG.
Methods: We utilized a Golgi staining method to investigate the dendritic morphology and spine density of young neurons in the inner granular cell layer (GCL) and mature neurons in the outer GCL in response to CORT application. The expressions of glucocorticoid receptors during neuronal maturation were examined using Western blot analysis in a primary hippocampal neuronal culture.
Results: Sholl analysis revealed that CORT treatment decreased the number of intersections and shortened the dendritic length in mature, but not young, granule cells. However, the spine density of mature and young neurons was not affected. Western blot analysis showed a progressive increase in the protein levels of glucocorticoid receptors (GRs) in the cultured primary hippocampal neurons during neuronal maturation.
Conclusion: These data suggest that mature neurons are likely more vulnerable to chronic exposure to CORT; this may be due to their higher expression of GRs when compared to younger DG neurons.
1Introduction
The granule cell layer (GCL) of the adult hippocampal dentate gyrus (DG) is a unique region of the adult mammalian brain in that it is one of a few brain regions that retains the capacity to generate neurons throughout life. This process, known as adult neurogenesis, is initiated by progenitor cells located in the subgranular zone of the DG. These progenitor cells give rise to daughter cells that must then differentiate into an immature neuronal phenotype and migrate into their final position in the GCL (Ming & Song, 2005).
The rate of neurogenesis in the DG is inversely related to the chronological age in rodents. Neurogenesis is highest early in life when the DG is undergoing rapid development, with approximately 17,000 cells born each day in the DG of peri-pubescent rats (Cameron & McKay, 2001). The rate of neurogenesis drops to a more moderate 2000– 4000 cells/day in 2– 6 month old adult animals (Cameron & McKay, 2001; Gil-Mohapel et al., 2013) and declines to a few hundred cells a day in the aged brain (Gil-Mohapel et al., 2013). One consequence of the age-related decrease in neurogenesis is the establishment of a chronological relationship between the age of a cell and its position in the GCL. In part this may also reflect physical constraints, as neurons generated in the mature brain are also tasked with migrating into an already extensively populated GCL (van Praag et al., 2002). As a result, the majority of adult born neurons appear to be preferentially located in the inner layer of the GCL (Esposito et al., 2005; Overstreet et al., 2004). Conversely, the outer layer appears to be primarily composed of more mature granule cells that were likely generated during the peri-pubescent period (Esposito et al., 2005).
While electrophysiological differences between young and mature neurons have been reported consistently (van Praag et al., 2002; Wang, Scott, & Wojtowicz, 2000), there also appears to be consistent morphological differences between young and mature neurons. Granule cells located in the outer aspects of the GCL tend to have several primary dendrites and more dendritic branching. In contrast, granule cells located in the inner aspects of the GCL are more likely to have only one primary dendrite emerging from the cell body and less dendritic branching (Claiborne, Amaral, & Cowan, 1990; Desmond & Levy, 1985; Green & Juraska, 1985). These morphological differences may be due to a temporal sequence of granule cells generation and dendritic development proceeding from the outer to inner granule cell layer, which leads to the gradient of neuronal ages found in the GCL (Schlessinger, Cowan, & Gottlieb, 1975; Wang et al., 2000). In the present study, we used cell morphology to determine the effects of prolonged corticosterone (CORT) administration on young and mature granule cells.
The effects of CORT are mediated through glucocorticoid receptors (GRs) and mineralocorticoid receptors (MRs). GRs are expressed by 50% of the radial glia-like progenitor cells, whereas MRs are expressed only in mature granule cells. Notably, fifty percent of dividing precursor cells don’t expression MR, but 50% of them express GR, while the old age calretinin-positive young neurons are both GR and MR positive. In particular, approximately 27% of dividing cells in the GCLs express GRs, and this percentage increases to 87% after 4 weeks of division, suggesting increased steroid sensitivity (Garcia, Steiner, Kronenberg, Bick-Sander, & Kempermann, 2004).
Given the differential expression of these two types of receptors on neurons at different stages of neuronal development, we speculated there would be different responses between young and mature granule neurons to CORT treatment. Exposure to chronic restraint, or treatment with 10 mg/kg CORT for three weeks, has led to dendritic atrophy in CA3 pyramidal neurons, but surprisingly these authors did not observe any changes in CA1 pyramidal cells or DG granule cells in the hippocampus (Watanabe, Gould, & McEwen, 1992; Woolley, Gould, & McEwen, 1990). It is possible that CORT may have a greater influence on mature granule cells because of the relatively high expression of GRs in these neurons. No differences being found in the DG by CORT in previous studies may be due to the fact that a population of young neurons with no changes in dendritic structure was included in the data analysis. In the present study we examined the effects of 40 mg/kg CORT on dendritic complexity, and spine density, in young and mature granular cells in the DG.
2Materials and methods
2.1Animals and housing conditions
All experimental procedures were approved by the Committee on the Use of Live Animals in Teaching and Research at the University of Hong Kong. Adult male Sprague-Dawley rats (250±20 g) and P0 rat pups were provided by the Animal Unit of The University of Hong Kong. The adult rats were housed 2 or 3 at a time in polyethylene cages with dimensions 48×27×20 cm on a 12 h light-dark cycle with ad libitum access to food and water at room temperature ∼22°C. Animals were randomly assigned to the vehicle-treated group (Control, n = 4) or the CORT-treated group (CORT, n = 6), where the rats were treated daily with sesame oil or CORT (40 mg/kg) for 14 days, respectively. Animals were then sacrificed 24 h after the last injection of CORT and fresh brains were isolated for Golgi staining. The P0 postnatal pups were used for harvesting hippocampal primary neurons for the in vitro study.
2.2Administration of CORT and sesame oil
Application of CORT at a dose of 40 mg/kg has been reported to elevate blood CORT levels over a 24-h period (Sapolsky, Krey, & McEwen, 1985). CORT (Sigma-Aldrich, St Louis, MO) was prepared according to the method of Hellsten et al.(Hellsten et al., 2002), and administered as previously described (Yau et al., 2011a, 2011b). In brief, a stock emulsion of CORT at a concentration of 33.3 mg/ml was prepared by suspending CORT in sesame oil, followed by sonication. Prior to every injection, the emulsion was vortexed briefly. A subcutaneous injection was made in the neck region daily at 4 : 00 p.m. for 14 days. The control animals were injected with sesame oilonly.
2.3Golgi staining and analysis
Golgi staining was performed according to the manufacturer’s instruction (FD Neurotechnologies Inc., Columbia, MD). Briefly, the freshly-dissected brain was impregnated at room temperature for two weeks in a mixture of Solution A and Solution B that contained potassium dichromate and chromate, then bathed in Solution C at 4°C for 48 hours. The brain was sectioned into 150-μm slices and transferred onto 2% gelatin-coated slides. The slices were dried at room temperature for 3 days and then stained with a mixture of Solution D, Solution E and distilled water (1 : 1:2 ratio) for 10 min, followed by gradient dehydration with 75%, 95% and 100% ethanol. Thereafter, the samples were cleared by xylene and mounted with Permount Medium (Fisher Scientific, Pittsburgh, PA). Sections were analyzed using Neurolucida software (MBF Bioscience, Williston, VT) and dendritic morphology was analyzed with a 40X objective lens. Sholl analysis was performed to quantify the number of dendritic branches and measure dendritic length at concentric10-μm intervals.
2.4Selection of granule cells
For each animal, 5-6 immature and mature neurons that met certain criteria were selected for analysis. The granule cells were selected and classified according to their position and morphology in the GCL of the DG. Cells in the inner cell layer that showed simple dendritic trees with one typical primary dendrite extension were considered young cells, whereas those in the outer cell layer with more complicated dendritic morphology and long dendrites were considered to be mature neurons (Bartesaghi & Serrai, 2001; Wang et al., 2000) (Fig. 1).
Granule cells were selected based on the criteria described by Woolley et al. (Woolley et al., 1990). In brief, the selected granule cells were isolated from neighboring cells to avoid interference in the analysis. Their cell bodies were consistently impregnated along the entire extent of all dendrites and positioned in the middle part of the section so as to minimize the cut of branch segments. Any cells with truncated dendritic branches were excluded from the analysis.
Spine density was estimated by randomly selecting a terminal segment of a dendritic branch longer than 10 μm for high-magnification tracing (60X lens). Three to five tertiary dendrites with at least one branch point were chosen for counting, and the visible spines along the branch segment were quantified. Ten branches per neuron were selected for spine count. The analysis was performed in a sample-blind manner. The data were expressed in units of spine numbers/10 μm.
2.5Culture of hippocampal neurons and immunoassay of GR expressions
Primary neurons were isolated from hippocampi of postnatal SD rats as described previously (Beaudoin et al., 2012; Fatima-Shad & Barry, 1998). Briefly, brains of P0 rat pups were isolated after decapitation and hippocampi were dissected immediately. Hippocampal pyramidal neurons were enzymatically dissociated with papain and DNase (Invitrogen, Carlsbad, CA), and maintained at a density of 80 cells/mm2 surface area in 6-well plates (Corning Life Science, Tewksbury MA) with the addition of cytosine arabinoside (Sigma-Aldrich) to inhibit the growth of dividing non-neuronal cells. Neurons were harvested on the designated DIVs (days in vitro) in order to obtain the lysates of cells with different maturities following a previously published protocol (Li et al., 2012). Total proteins were separated by SDS-PAGE and transferred to PVDF membranes (Millipore, Billerica, MA), followed by sequential incubations at room temperature with 3% bovine serum albumin in Tris-HCl buffer containing 0.1% Tween-20 (TBST) for 1 hr, primary antibodies for 3 hrs, and secondary antibodies conjugated to horseradish peroxidase (HRP) for 2 hrs. Blots were developed by chemiluminescence detection (Luminata Forte, Millipore), and protein expression levels were determined by densitometry with Image J software (Version 1.48, NIH, Bethesda, MD). The antibodies used were: mouse anti-β-actin (1 : 8000); mouse anti-GR (1 : 200; Abcam, Cambridge, MA), and goat anti-mouse IgG-HRP (1 : 8000, DAKO, Carpinteria, CA).
2.6Statistical analysis
Data was analyzed by Two-way repeated measure ANOVA, One-way ANOVA, and Students’ t-test using SPSS 16.0 as appropriate. A p-value less than 0.05 was considered significant.
3Results
3.1CORT treatment induced dendritic atrophy specifically in mature, but not young granule cells
Mature granule cells showed more intersections (Fig. 2A effect of maturity: F3,29 = 13.647, P = 0.000122; effect of CORT: F3,29 = 2.378, P = 0.000100, Two-way repeated measure ANOVA) and longer dendrites compared to the young granule cells (Fig. 2B effect of maturity: F3,29 = 15.126, P = 0.000519; effect of CORT: F3,29 = 3.959, P = 0.000903. Repeated measure Two-way ANOVA). The 2-week CORT treatment significantly decreased dendritic branching as evident by the fewer intersections in mature granule cells of CORT-treated rats (Fig. 2C F1,29 = 2.294, P = 0.000367. Repeated measure One-way ANOVA). Significant decreases in the number of intersections were observed at shell distances of 160, 170, 180, 210, 220 and 270 μm (P < 0.05 vs. Controls, Student’s t-test). Additionally, the dendritic length was also substantially reduced by CORT treatment (F1,29 = 2.406, P = 0.000104, Repeated measure One-way ANOVA). Significant differences between the CORT-treated and control groups at shell distances of 170, 210, 220, 230 and 290 μm were observed (Fig. 2D P < 0.05 vs. Controls, Student’s t-test.). In contrast, CORT showed no significant effect on the number of intersections (Fig. 2E F1,29 = 0.797, P = 0.763) and dendritic length of young neurons (Fig. 2F F1,29 = 1.503, P = 0.054). Taken together, these data suggest that mature neurons in the outer cell layer were more susceptible to changes in dendritic complexity in response to CORT exposure than young neurons.
3.2CORT treatment did not affect cell body area and spine density in granule cells
In contrast to the differential responses of young and mature cells to CORT treatment, there was no significant decrease in cell body area or spine density in either cell population, indicating that CORT specifically affects dendritic outgrowth of dentate granule cells (Fig. 3 P > 0.05 vs. Controls, Student’s t-test).
3.3Increased expression of glucocorticoid receptors during neuronal maturation
Primary cultures of hippocampal neurons obtained from P0 rat pups underwent typical maturation stages, including the extension of lamellopodia, specification of axons and dendrites, and synapse formation and extension, as documented by others (Beaudoin et al., 2012). Expression of GRs appeared higher on DIV 4 and was significantly elevated on DIV12 (12 days in vitro) when compared to that on DIV 0 (3 hrs after plating cells) (Fig. 4), suggesting a progressive increase in the expression of GRs during neuronalmaturation.
4Discussion
Subcutaneous administration of CORT to rodents has been shown to elevate levels of CORT in the blood over a 24-h period (Sousa, Almeida, Holsboer, Paula-Barbosa, & Madeira, 1998). We have previously shown that 40 mg/kg CORT treatment for two weeks leads to an approximate 2-fold increase in plasma CORT levels when compared to the rats which were subjected to 2-weeks exposure of chronic mild stress (CMS) (40 mg/kg CORT: 613±120 ng/ml versus CMS: 295±91 ng/ml) (Yau et al., 2011a), suggesting treatment with 40 mg/kg CORT for two weeks could mimic the detrimental effect of an aversive stress response in the animals. In the present study, we demonstrated that CORT differentially affected dendritic complexity of granule cells located in the inner and outer granule cell layers, indicating that younger and more mature granule cells can respond differently to CORT exposure. This may be due to an increased sensitivity to CORT in mature granule cells as suggested by increased expressions of GRs in hippocampal primary neurons undergoing maturation.
In a rat model of depression induced by a two-week CORT treatment, the rats showed dendritic atrophy in the CA3 pyramidal neurons and an aberrant adult neurogenesis in the DG region. These rats also showed impairment in hippocampal dependent learning and memory and increased depression-like behavior (Yau et al., 2011a). Furthermore, the present study showed that these CORT-treated rats demonstrated decreased dendritic complexity specific to a population of more mature granule cells, which has not been reported previously. Depressed patients often show chronic elevation in blood cortisol levels and reduction in hippocampal volume (Videbech & Ravnkilde, 2004). Therefore, it is likely that structural deficits appeared in the hippocampal subregions, including impaired adult neurogenesis and dendritic atrophy in the DG and CA regions induced by chronic corticosteroid exposure, may contribute to behavioral deficits associated with depression (Kim & Diamond, 2002; McEwen & Magarinos, 2001).
Prolonged exposure to chronic stress or 21 days of corticosterone administration at a dose of 10 mg/kg has been shown to directly induce dendritic atrophy, particularly in the hippocampal CA3 region, but not in the CA1 or dentate regions (Watanabe et al., 1992; Woolley et al., 1990). Also, postpartum CORT administration can reduce dendritic arborization in basal dendrites of the CA3 pyramidal neurons (Workman, Brummelte, & Galea, 2013). In contrast, a 3-month CORT treatment at the dose which elevates blood CORT to a comparable level of that exposed to mild stress or 3-week stress exposure significantly impairs induction of LTP in the CA1, CA3 and DG regions (Alfarez, Joels, & Krugers, 2003; Bodnoff et al., 1995; Pavlides, Nivon, & McEwen, 2002). This suggests that a longer treatment of CORT could exert adverse effects on all hippocampal regions. Exposure to chronic mild stress for six weeks has been shown to decrease the total dendritic length of mature granule neurons in the DG, but not in the CA1 (Bessa et al., 2009). However, it has been reported that exposure to 14 days of stress can induce dendritic retraction and decrease spine density in CA1 pyramidal neurons (Lambert et al., 1998). Sousa and colleagues have reported that exposure to chronic unpredictable stress or 40 mg/kg CORT treatment for four weeks, decreased dendritic complexity in the CA3, CA1 and DG regions; however, spine density was not affected in these regions (Sousa, Lukoyanov, Madeira, Almeida, & Paula-Barbosa, 2000). Similarly, Alfarez and colleagues reported that CORT reduced dendritic complexity, but not spine density in CA1 pyramidal neurons (Alfarez et al., 2003). We have shown a similar observation in this study where two-week CORT treatment induced dendritic atrophy without affecting spine density in mature granule cells. The varied effects of CORT on dendritic morphology may be due to varied levels of the stress insult to animals, different doses or durations of CORT treatments, or sex differences of animals utilized in studies, as the detrimental effect of stress/CORT exposure is dose-, duration- and sex-dependent (Gregus, Wintink, Davis, & Kalynchuk, 2005; Johnson, Fournier, & Kalynchuk, 2006; Leuner & Gould, 2010; Sebastian, Estil, Chen, Schrott, & Serrano, 2013).
Through our analysis of young and mature neurons, we found that dendritic retraction following the 2-week treatment with 40 mg/kg CORT specifically occurred to mature neurons at the outer cell layer. This raises the possibility that previous failures to detect the effects of CORT treatment on the DG region may be attributed by the heterogeneity of cell populations in the granular cell layer. Our findings support the suggestion of Green and Juraska (Green & Juraska, 1985) that the position of the soma in the GCL should be taken into account when analyzing dendritic complexity in dentate granule cells. However, the limitation of the current study is that neurons chosen as young neurons was not confirmed with a secondary measure, such as doublecortin immunostaining with Golgi-stained neurons. In light of the difficulty in performing the co-labeling of doublecortin with Golgi-stained neurons, the current study may have included a population with immature neurons with varied stages of maturity.
Our data has suggested that mature neurons could be more vulnerable to the detrimental effect of CORT treatment when compared to young neurons. Garcia et al., (Garcia et al., 2004) have suggested that expression of GRs and MRs increases as time elapses after cell division of neural progenitor cells. The higher expression levels of GRs and MRs in mature neurons may account for their greater sensitivity to CORT treatment. We have shown that expression levels of GRs were significantly increased in primary hippocampal neurons during maturation. This result may suggest a relatively higher level of GRs in mature neurons compared to young neurons. However, hippocampal cell cultures adopted in the current study may have included a mixture of cells, and changes of glucocorticoid receptors may not be only produced by granule cells. Examining the expression of GRs and MRs in young and mature granule neurons may help to provide more evidence in the in vivo environment in the future study.
Differential sensitivities to CORT within the hippocampus may be attributed to different expression levels of GRs and MRs in hippocampal sub-regions. Both the changes of neurogenesis in the DG, and remodeling of dendrites and synapses of neurons in hippocampal sub-regions, may contribute to neuronal adaptation in response to external stimuli. With the limitations of Golgi staining where only a subset of neurons could be stained, as well as the selection process of isolated cells for analysis, our results may not fully represent the whole population of young and mature granule cells. However, our data demonstrates that treatment with 40 mg/kg CORT for two weeks induces dendritic retraction in mature, but not young granule cells in the DG. Differential responses of mature and young neurons to CORT exposure suggests mature neurons have an increasing susceptibility to the detrimental effect of stress (Garcia et al., 2004).
Conflict of interest
The authors report no conflicts of interest.
Acknowledgments
The study is supported by Funds of Leading Talents of Guangdong (2013), Programme of Introducing Talents of Discipline to Universities (B14036), and Project of International, as well as Hong Kong, Macao & Taiwan Science and Technology Cooperation Innovation Platform in Universities in Guangdong Province (2013gjhz0002).
References
1 | Alfarez D.N. , Joels M. , & Krugers H.J. ((2003) ). Chronic unpredictable stress impairs long-term potentiation in rat hippocampal CA1 area and dentate gyrus in vitro. European Journal of Neuroscience, 17: , 1928–1934. |
2 | Bartesaghi R. , & Serrai A. ((2001) ). Effects of early environment on granule cell morphology in the dentate gyrus of the guinea-pig. Neuroscience, 102: , 87–100. |
3 | Beaudoin G.M. 3rd , Lee S.H. , Singh D. , Yuan Y. , Ng Y.G. , Reichardt L.F. , & Arikkath J. . ((2012) ). Culturing pyramidal neurons from the early postnatal mouse hippocampus and cortex. Nature Protocol, 7: , 1741–1754. |
4 | Bessa J.M. , Ferreira D. , Melo I. , Marques F. , Cerqueira J.J. , Palha J.A. , … & Sousa N. ((2009) ). The mood-improving actions of antidepressants do not depend on neurogenesis but are associated with neuronal remodeling. Molecular Psychiatry, 14: , 764–773, 739. |
5 | Bodnoff S.R. , Humphreys A.G. , Lehman J.C. , Diamond D.M. , Rose G.M. , & Meaney M.J. ((1995) ). Enduring effects of chronic corticosterone treatment on spatial learning, synaptic plasticity, and hippocampal neuropathology in young and mid-aged rats. Journal of Neuroscience, 15: , 61–69. |
6 | Cameron H.A. , & McKay R.D. ((2001) ). Adult neurogenesis produces a large pool of new granule cells in the dentate gyrus. Journal of Comparative Neurology, 435: , 406–417. |
7 | Claiborne B.J. , Amaral D.G. , & Cowan W.M. ((1990) ). Quantitative, three-dimensional analysis of granule cell dendrites in the rat dentate gyrus. Journal of Comparative Neurology, 302: , 206–219. |
8 | Desmond N.L. , & Levy W.B. ((1985) ). Granule cell dendritic spine density in the rat hippocampus varies with spine shape and location. Neuroscience Letter, 54: , 219–224. |
9 | Esposito M.S. , Piatti V.C. , Laplagne D.A. , Morgenstern N.A. , Ferrari C.C. , Pitossi F.J. , & Schinder A.F. ((2005) ). Neuronal differentiation in the adult hippocampus recapitulates embryonic development. Journal of Neuroscience, 25: , 10074–10086. |
10 | Fatima-Shad K. , & Barry P.H. ((1998) ). Morphological and electrical characteristics of postnatal hippocampal neurons in culture: The presence of bicuculline- and strychnine-sensitive IPSPs. Tissue and Cell, 30: , 236–250. |
11 | Garcia A. , Steiner B. , Kronenberg G. , Bick-Sander A. , & Kempermann G. ((2004) ). Age-dependent expression of glucocorticoid- and mineralocorticoid receptors on neural precursor cell populations in the adult murine hippocampus. Aging Cell, 3: , 363–371. |
12 | Gil-Mohapel J. , Brocardo P.S. , Choquette W. , Gothard R. , Simpson J.M. , & Christie B.R. ((2013) ). Hippocampal neurogenesis levels predict WATERMAZE search strategies in the aging brain. PLoS One, 8: , e75125. |
13 | Green E.J. , & Juraska J.M. ((1985) ). The dendritic morphology of hippocampal dentate granule cells varies with their position in the granule cell layer: A quantitative Golgi study. Experimental Brain Research, 59: , 582–586. |
14 | Gregus A. , Wintink A.J. , Davis A.C. , & Kalynchuk L.E. ((2005) ). Effect of repeated corticosterone injections and restraint stress on anxiety and depression-like behavior in male rats. Behavioral Brain Research, 156: , 105–114. |
15 | Hellsten J. , Wennstrom M. , Mohapel P. , Ekdahl C.T. , Bengzon J. , & Tingstrom A. ((2002) ). Electroconvulsive seizures increase hippocampal neurogenesis after chronic corticosterone treatment. European Journal of Neuroscience, 16: , 283–290. |
16 | Johnson S.A. , Fournier N.M. , & Kalynchuk L.E. ((2006) ). Effect of different doses of corticosterone on depression-like behavior and HPA axis responses to a novel stressor. Behavioral Brain Research, 168: , 280–288. |
17 | Kim J.J. , & Diamond D.M. ((2002) ). The stressed hippocampus, synaptic plasticity and lost memories. Nature Review Neuroscience, 3: , 453–462. |
18 | Lambert K.G. , Buckelew S.K. , Staffiso-Sandoz G. , Gaffga S. , Carpenter W. , Fisher J. , & Kinsley C.H. ((1998) ). Activity-stress induces atrophy of apical dendrites of hippocampal pyramidal neurons in male rats. Physiological Behavior, 65: , 43–49. |
19 | Leuner B. , & Gould E. ((2010) ). Structural plasticity and hippocampal function. Annunal Review of Psychology, 61: , 111–140, C111-C113. |
20 | Li A. , Leung C.T. , Peterson-Yantorno K. , Stamer W.D. , Mitchell C.H. , & Civan M.M. ((2012) ). Mechanisms of ATP release by human trabecular meshwork cells, the enabling step in purinergic regulation of aqueous humor outflow. Journal of Cellular Physiology, 227: , 172–182. |
21 | McEwen B.S. , & Magarinos A.M. ((2001) ). Stress and hippocampal plasticity: Implications for the pathophysiology of affective disorders. Human Psychopharmacology, 16: , S7–S19. |
22 | Ming G.L. , & Song H. ((2005) ). Adult neurogenesis in the mammalian central nervous system. Annual Review of Neuroscience, 28: , 223–250. |
23 | Overstreet L.S. , Hentges S.T. , Bumaschny V.F. , de Souza F.S. , Smart J.L. , Santangelo A.M. , … & Rubinstein M. ((2004) ). A transgenic marker for newly born granule cells in dentate gyrus. Journal of Neuroscience, 24: , 3251–3259. |
24 | Pavlides C. , Nivon L.G. , & McEwen B.S. ((2002) ). Effects of chronic stress on hippocampal long-term potentiation. Hippocampus, 12: , 245–257. |
25 | Sapolsky R.M. , Krey L.C. , & McEwen B.S. ((1985) ). Prolonged glucocorticoid exposure reduces hippocampal neuron number: Implications for aging. Journal of Neuroscience, 5: , 1222–1227. |
26 | Schlessinger A.R. , Cowan W.M. , & Gottlieb D.I. ((1975) ). An autoradiographic study of the time of origin and the pattern of granule cell migration in the dentate gyrus of the rat. Journal of Comparative Neurology, 159: , 149–175. |
27 | Sebastian V. , Estil J.B. , Chen D. , Schrott L.M. , & Serrano P.A. ((2013) ). Acute physiological stress promotes clustering of synaptic markers and alters spine morphology in the hippocampus. PLoS One, 8: , e79077. |
28 | Sousa N. , Lukoyanov N.V. , Madeira M.D. , Almeida O.F. , & Paula-Barbosa M.M. ((2000) ). Reorganization of the morphology of hippocampal neurites and synapses after stress-induced damage correlates with behavioral improvement. Neuroscience, 97: , 253–266. |
29 | Sousa N. , Almeida O.F. , Holsboer F. , Paula-Barbosa M.M. , & Madeira M.D. ((1998) ). Maintenance of hippocampal cell numbers in young and aged rats submitted to chronic unpredictable stress. Comparison with the effects of corticosterone treatment. Stress, 2: , 237–249. |
30 | van Praag H. , Schinder A.F. , Christie B.R. , Toni N. , Palmer T.D. , & Gage F.H. ((2002) ). Functional neurogenesis in the adult hippocampus. Nature, 415: , 1030–1034. |
31 | Videbech P. , & Ravnkilde B. ((2004) ). Hippocampal volume and depression: A meta-analysis of MRI studies. American Journal of Psychiatry, 161: , 1957–1966. |
32 | Wang S. , Scott B.W. , & Wojtowicz J.M. ((2000) ). Heterogenous properties of dentate granule neurons in the adult rat. Journal of Neurobiology, 42: , 248–257. |
33 | Watanabe Y. , Gould E. , & McEwen B.S. ((1992) ). Stress induces atrophy of apical dendrites of hippocampal CA3 pyramidal neurons. Brain Research, 588: , 341–345. |
34 | Woolley C.S. , Gould E. , & McEwen B.S. ((1990) ). Exposure to excess glucocorticoids alters dendritic morphology of adult hippocampal pyramidal neurons. Brain Research, 531: , 225–231. |
35 | Workman J.L. , Brummelte S. , & Galea L.A. ((2013) ). Postpartum corticosterone administration reduces dendritic complexity and increases the density of mushroom spines of hippocampal CA3 arbours in dams. Journal of Neuroendocrinology, 25: , 119–130. |
36 | Yau S.Y. , Lau B.W. , Tong J.B. , Wong R. , Ching, Y.P. , Qiu G. , … & So K.F. , ((2011) a). Hippocampal neurogenesis and dendritic plasticity support running-improved spatial learning and depression-like behaviour in stressed rats. PLoS One, 6: , e24263. |
37 | Yau S.Y. , Lee J.C.D. , Lau B.W.M. , Lee T.M.C. , Ching Y.P. , Tang S.W. , & So K.F. . ((2011) b). Low dose of corticosterone treatment with exercise increases hippocampal cell proliferation,and improves cognition. Neural Regeneration Research, 6: , 2645–2655. |
Figures and Tables
Fig.1
Classification of young and mature neurons in the DG. (A) Representative images of Golgi-impregnated granule cells. (B) Cells residing in the inner granule cell layer with single dendritic extensions were classified as young cells, whereas those with multiple dendrites that resided in the outer cell layer were classified as mature. Scale bars: 50 μm. (C) Dendritic tracing of a young neuron and (D) Dendritic tracing of a mature neurons by using the software Neurolucida.
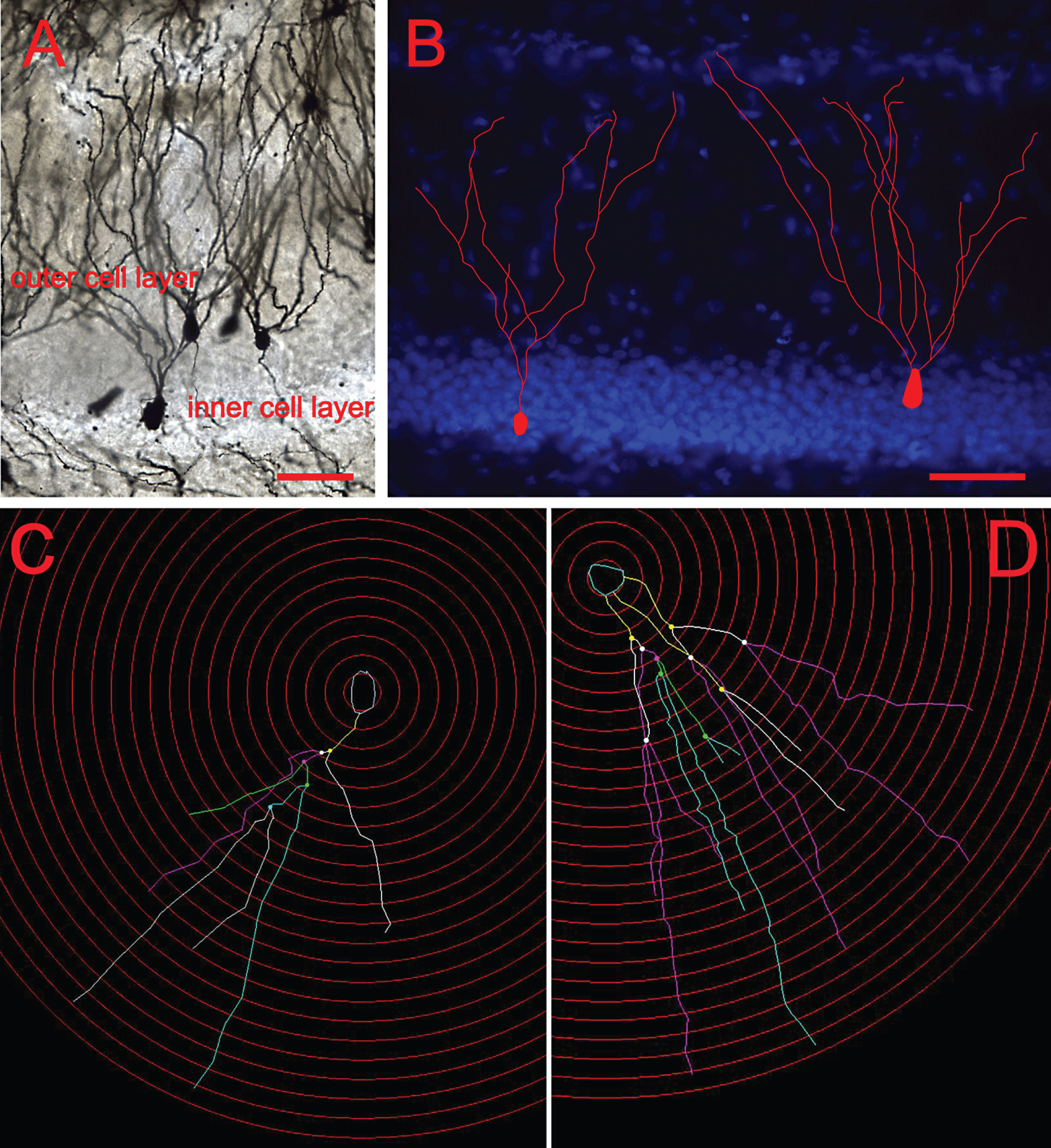
Fig.2
CORT treatment reduces number of intersection and dendritic length in mature granule cells. Mature granule cells showed significant higher number of intersections (A) and dendritic length (B) when compared to young granule cells. Two-way repeated measure ANOVA. *p < 0.05 main effect of maturity of neurons. CORT significantly reduced both the number of intersections (C) and the dendritic length of mature granule cells in the outer cell layer (D) at the designated Shell distances. In contrast, dendritic branching (E) and length (F) of immature granule cells were not significantly affected in the inner cell layer. *P < 0.05 compared to controls.
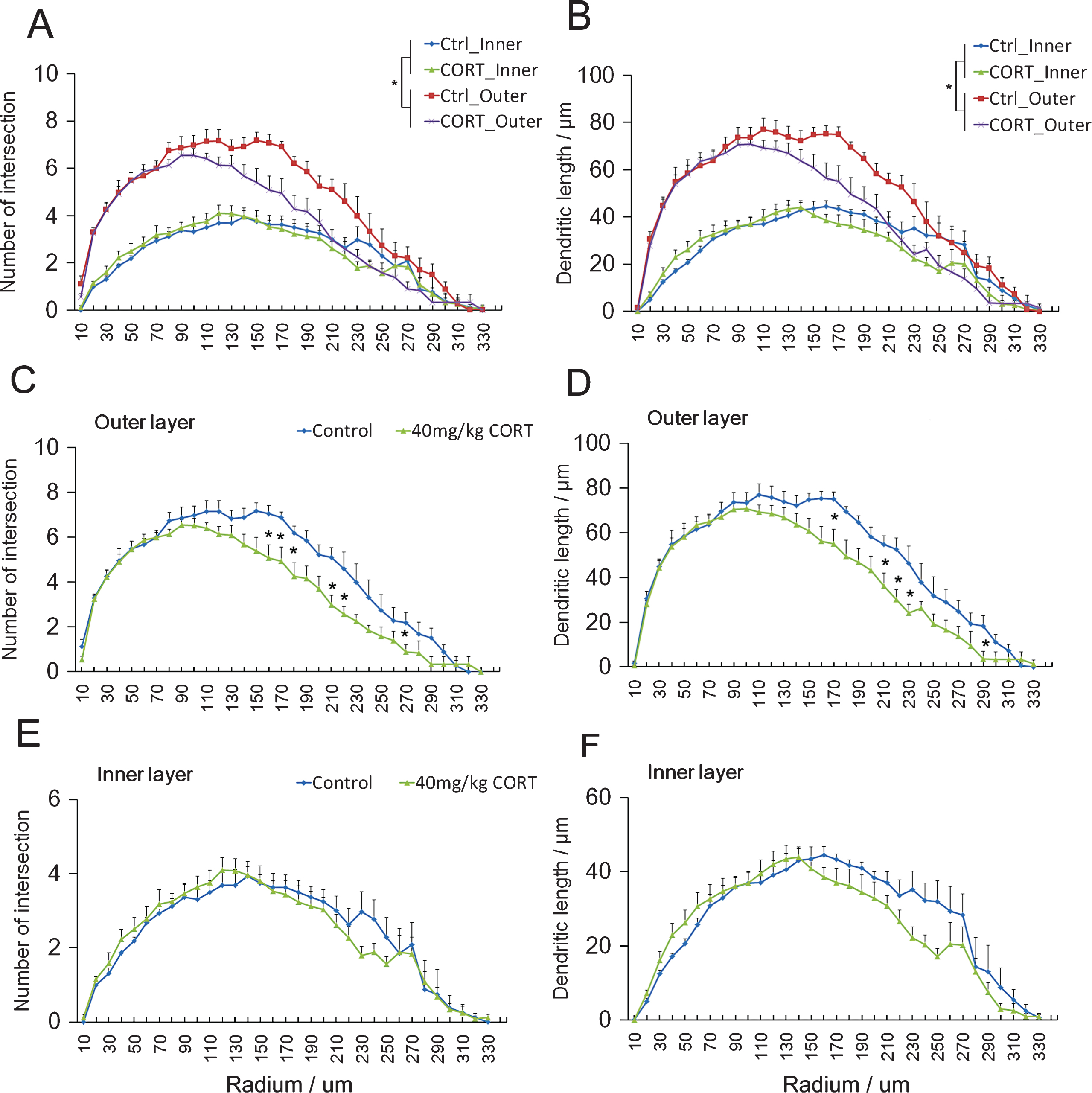
Fig.3
CORT treatment does not affect spine density of neurons in inner and outer cell layers CORT treatment did not affect the soma area. (A) or the spine density (B) in both the inner and the outer cell layers. Scale bar: 20 μm.
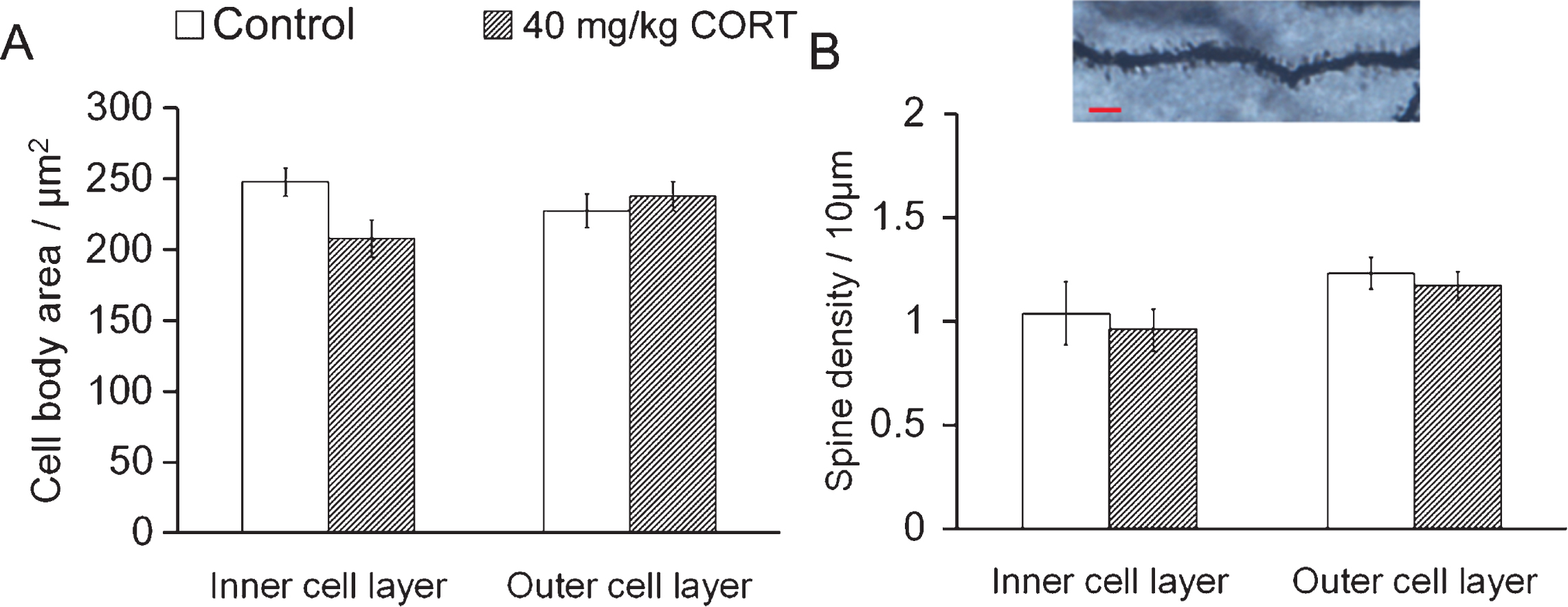
Fig.4
Increased expression of glucocorticoid receptors during neuronal maturation. (A) Immunoblotting analysis was concluded from 4-5 independent experiments using primary hippocampal neurons with β-actin as the loading control. (B) Quantitative results showed that the expression of GRs on Day 12 was significantly higher than that on Day 0 (3 hrs after plating cells), suggesting a progressive increase in GR expression during neuronal maturation. **P < 0.01 vs. Day 0 controls by one-way ANOVA with Tukey’s post-hoc test.
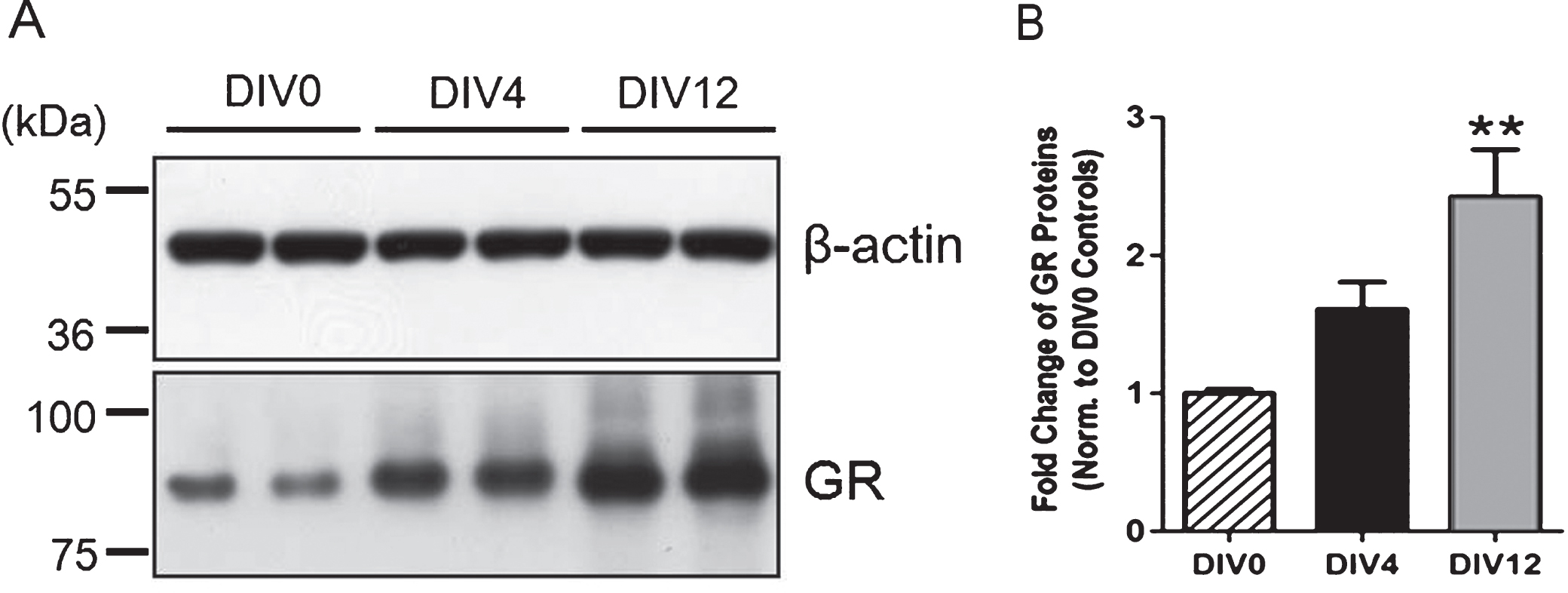