Head and vestibular kinematics during vertical semicircular canal impulses
Abstract
BACKGROUND:
The video head impulse test (vHIT) is a common assessment of semicircular canal function during high-speed impulses. Reliability of the vHIT for assessing vertical semicircular canals is uncertain. Vertical head impulses require a complex head movement, making it difficult to isolate a single semicircular canal and interpret resulting eye rotations.
OBJECTIVE:
The purpose of this study was to provide descriptive head kinematics and vestibular stimuli during vertical plane impulses to ultimately improve impulse delivery and interpretation of vHIT results for vertical semicircular canals.
METHODS:
Six participants received right anterior (RA) and left posterior (LP) semicircular canal impulses. Linear displacements, rotational displacements, and rotational velocities of the head were measured. Peak velocities in semicircular canal planes and peak-to-peak gravitoinertial accelerations at the otolith organs were derived from head kinematics.
RESULTS:
The largest rotational velocities occurred in the target semicircular canal plane, with non-negligible velocities occurring in non-target planes. Larger vertical displacements and accelerations occurred on the right side of the head compared to the left for RA and LP impulses.
CONCLUSIONS:
These results provide a foundation for designing protocols to optimize stimulation applied to a singular vertical semicircular canal and for interpreting results from the vHIT for vertical semicircular canals.
1Introduction
The video head impulse test (vHIT) is a widely used tool for the assessment of semicircular canal function, especially the horizontal semicircular canals [11]. The vHIT consists of applying rapid rotational head impulses reaching 150°/s –250°/s while an individual fixates a visual target about one meter away [2, 4, 15, 17]. The vHIT evaluates the vestibulo-ocular reflex (VOR) by estimating the gain of the VOR [15, 17, 24]. A reduced gain suggests a reduction of function in a particular semicircular canal [15, 17, 24]. The primary function of the VOR is to maintain gaze stability and preserve visual acuity during head movement [8]. When the VOR is impaired, the individual is no longer able to sustain visual fixation on a target during head movement, which can result in dizziness, visual blurring, and imbalance [8]. Diagnosing deficits in the VOR can be accomplished with the vHIT, which can augment other objective vestibular tests such as caloric testing and rotational chair testing [3, 25]. However, the vHIT is emerging as the preferred choice of test modality because of the portability of the equipment and the purported ability to test all six semicircular canals rather than only the horizontal semicircular canals [5, 6, 15, 16, 20, 25]. With the increasing popularity of the vHIT, several manufacturers have developed equipment and software designed to enable vHIT to be used clinically [18, 24]. When assessing the horizontal semicircular canals, the vHIT has shown good reliability [15, 18, 24].
Despite the demonstrated reliability of the vHIT for assessing the horizontal semicircular canals [19, 23], the reliability of vHIT for assessing the vertical semicircular canals does not appear to be high [24]. A primary challenge for using vHIT to assess the vertical semicircular canals is the orientation of the vertical semicircular canals in the head. To stimulate a vertical semicircular canal, a complex 3D movement of the head is required, consisting of equal amounts of pitch and roll with minimal yaw [12, 24]. On average, the horizontal semicircular canals lie in a plane pitched 18.8° up from Reid’s plane [13]. The plane of each vertical semicircular canal, i.e. the right anterior (RA) left posterior (LP), and left anterior (LA) right posterior (RP), are approximately perpendicular to the nominal plane of the horizontal semicircular canals and are angled about +/–45° with respect to the mid-sagittal head plane [13]. The nominal planes of the RA and LP semicircular canals are parallel and form the so-called “RALP plane”, while the nominal planes of the LA and RP semicircular canals are parallel and lie in the so-called “LARP plane”, which is nominally perpendicular to the “RALP plane”. The basis of the vHIT is to provide a rotational velocity stimulus in a single semicircular canal plane, thereby exciting a single semicircular canal, while inhibiting the other semicircular canal in that plane [9]. Complementary goals include 1) minimizing the linear displacement of the head to reduce otolithic contributions to eye rotations and 2) minimizing rotations in other semicircular canal planes to reduce contributions from other semicircular canals to eye rotations. These goals are more easily attained for the horizontal semicircular canals than for the vertical semicircular canals. A rotational stimulus can be aligned with the horizontal semicircular canals easily by flexing the individual’s head forward to align the horizontal semicircular canals with earth-horizontal and then rotating the head in the earth-horizontal plane [19]. However, vertical semicircular canal plane impulses require a complex combination of head rotation and head displacement because of the orientation of the vertical semicircular canals in the head and the biomechanics of head rotation on the neck. Unfortunately, unlike the primary axial rotation of the cervical spine during horizontal semicircular canal impulses, the impulse movement for vertical semicircular canals does not align with a single rotational axis of the cervical spine. Therefore, to accurately assess vertical semicircular canal function, there must first be a foundational understanding of the complex 3D head movements and resulting stimuli applied to the vestibular system during vertical semicircular canal impulses.
The primary purpose of this study was to describe the 3D kinematics of head movements and the resulting stimuli applied to the vestibular apparatus, i.e. the semicircular canals and otolith organs, during vertical semicircular canal impulses. These data can serve as the basis for a better understanding of the eye rotations during the vHIT and for accurately calculating VOR gains that can ultimately improve the reliability of detecting vertical semicircular canal dysfunction.
2Methods
2.1Participants
Six healthy participants were recruited for this study (22.5±2.0 years old; 3 F). Exclusion criteria included a history of neurologic disease, vestibular disorder, or serious neck injury. Participants were screened for current neck pain or reduced neck range of motion. No participants were excluded for these reasons. A physical therapist with seven years of experience in vestibular testing and rehabilitation performed the testing on each participant. All participants provided informed consent before testing. IRB approval was given by the University of Pittsburgh (Study ID: 19070191).
2.2Equipment and data processing
A fourteen-camera VICON motion capture system (Vicon Motion Systems Ltd., Centennial, CO) was used to track head movements during impulses. Markers were placed on the left and right side of the forehead slightly anterior to the tragus of each ear and over the zygomatic process inferior to each eye (Fig. 1A). A static trial in which the participant was aligned with head straight ahead and with Reid’s plane earth-horizontal was recorded. Reid’s plane is defined as the best-fit plane containing the center of each bony external auditory canal and the most inferior aspect of each infraorbital rim [13]. During the set-up for the impulses, the Vicon reflective eye markers were removed and the vHIT goggles (Neurolign USA, formerly known as Neuro Kinetics, Inc., Pittsburgh, PA) were placed on the participant’s head. Gyroscope, accelerometer, and eye rotation data from the goggles are not reported in this study, but were collected for aspects of a larger study investigating the effects of different starting head positions on head impulse test biomechanics. Four additional markers were placed on the goggles to account for potential loss of marker visibility (Fig. 1B). Marker data were low-pass filtered using a zero phase minimum (54th) order infinite impulse response digital filter with a cut-off frequency of 5 Hz. All filtering and differentiation were performed in MATLAB (Version R2022a, MathWorks, Inc., Natick, MA).
Fig. 1
Illustration of markers used for Vicon motion capture system. A) Initial static trials were used to define head coordinates using markers placed bilaterally on the ear tragus, zygomatic process, and lateral forehead. The participant was aligned with Reid’s plane earth-horizontal with no head yaw. B) Goggles and additional markers were placed on the participant for recording movements during the impulses.
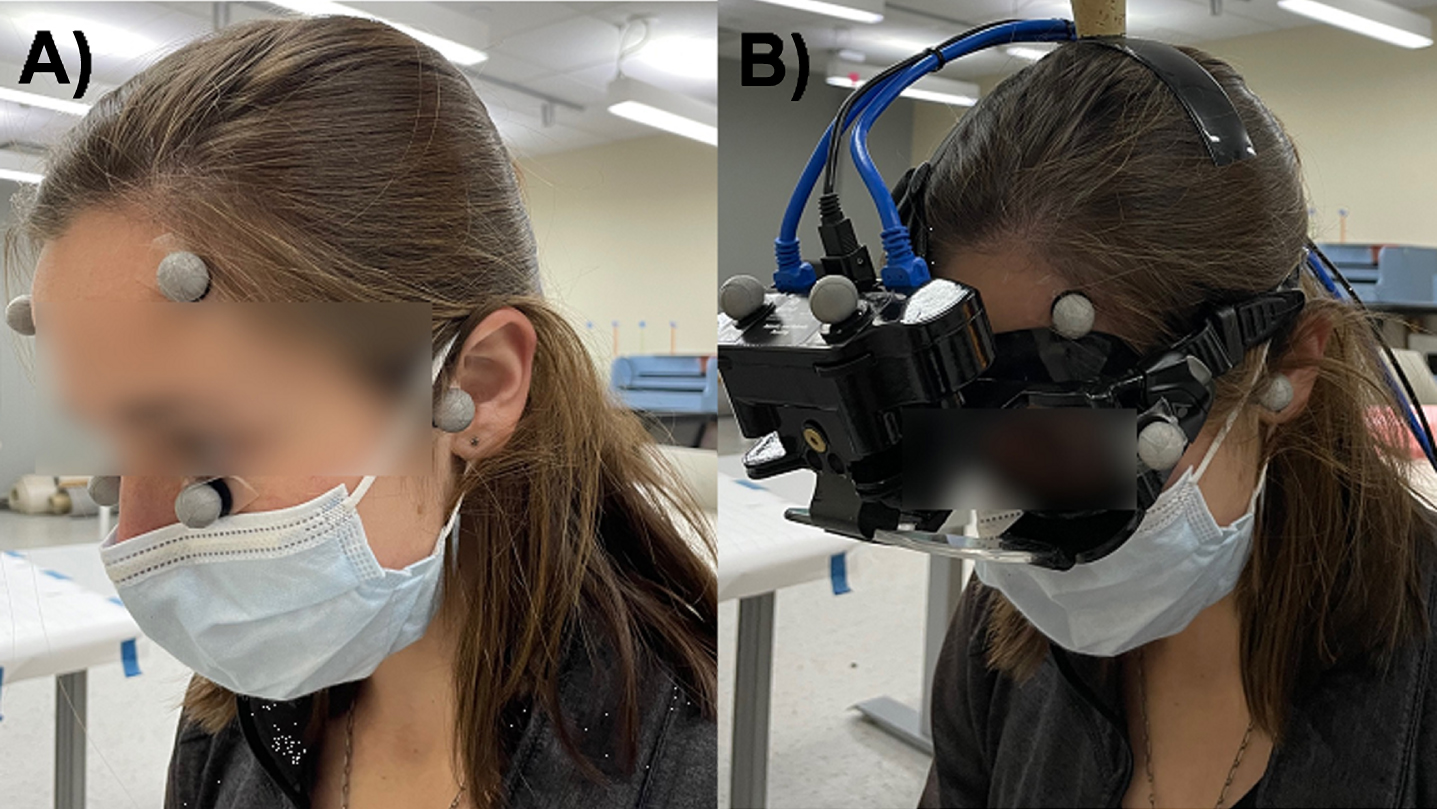
2.3Protocol
Participants sat one meter in front of a wall where visual targets were placed. The participant’s head was positioned such that Reid’s plane was aligned with earth horizontal, which was verified using a digital level aligned to the infraorbital rims and external auditory canals. The head was then pitched down another 18.8° to place the horizontal semicircular canal plane earth horizontal. The head was then rotated 22° to the left about the vertical axis, which is the mid-point between starting head positions used in commercially available existing protocols [1, 21]. From this starting head position, only RALP plane impulses were applied, with an amplitude of about 20° [1, 4]. A visual guide was placed on the vHIT goggles aligned with the RALP plane to assist the examiner in applying impulses in the correct direction. For the data reported here, each participant received five right anterior (RA) and five left posterior (LP) impulses. Additional impulses occurred in other initial head positions, which will be the focus of another study. Trials were blocked by semicircular canal (RA or LP). After each impulse, the head was slowly returned to the starting position. The order in which the semicircular canals were tested first was randomized across participants.
2.4Head kinematics
The processed marker data were used to calculate the head kinematics using 3D rigid body mechanical analysis [26]. Head rotations and translations in space were computed. From these kinematic data, the movements of the vestibular apparatus and the orbits were then calculated.
2.5Head rotation and translation
Rotations of the head in pitch, roll, and yaw along anatomically meaningful axes were determined using the following coordinate system (Fig. 2). The pitch axis was directed from the tragus marker on the left ear toward the tragus marker on the right ear, the yaw axis was defined by the cross product between the pitch axis and a vector directed from the marker on the tragus of the left ear to the marker on the left zygomatic process just below the orbit, and the roll axis was defined as the cross product between the yaw axis and the pitch axis (Fig. 2A). The origin of the coordinate system was placed at the midpoint between tragus markers placed on the left and right ear (mid-ear) with positive directions toward the right, anterior, and superior. By this definition, the plane containing the pitch and roll axes was aligned with Reid’s plane. Pitch, roll, and yaw rotations were determined using an Euler angle decomposition [26]. The head rotations were determined with respect to the position of the head in the static trial. The head rotations were subsequently used to determine the rotations in the canal planes, as described below (see Vestibular Stimuli section). The displacements of the head origin (mid-ear location) in global space were also determined. Rotational head velocities and linear displacements of the head origin were computed. The vertical displacements of the right and left orbits were determined by using their relative position with respect to the defined head coordinate system. Vertical displacements of the orbits were calculated, as this is the direction in which displacements of the otolith organs may affect the resultant vertical eye movements used to calculate VOR gain for the vertical semicircular canals.
Fig. 2
Coordinate systems for the study. A) The head coordinate system was defined with the origin at the midpoint between the ears. The roll and pitch axes were aligned in Reid’s plane and the yaw axis was perpendicular to Reid’s plane. B) The semicircular canal coordinate system was pitched upward 18.8° and subsequently yawed about the new vertical axis 45° to the left with respect to the head coordinate system to define the RALP, LARP, and horizontal (HORZ) axes.
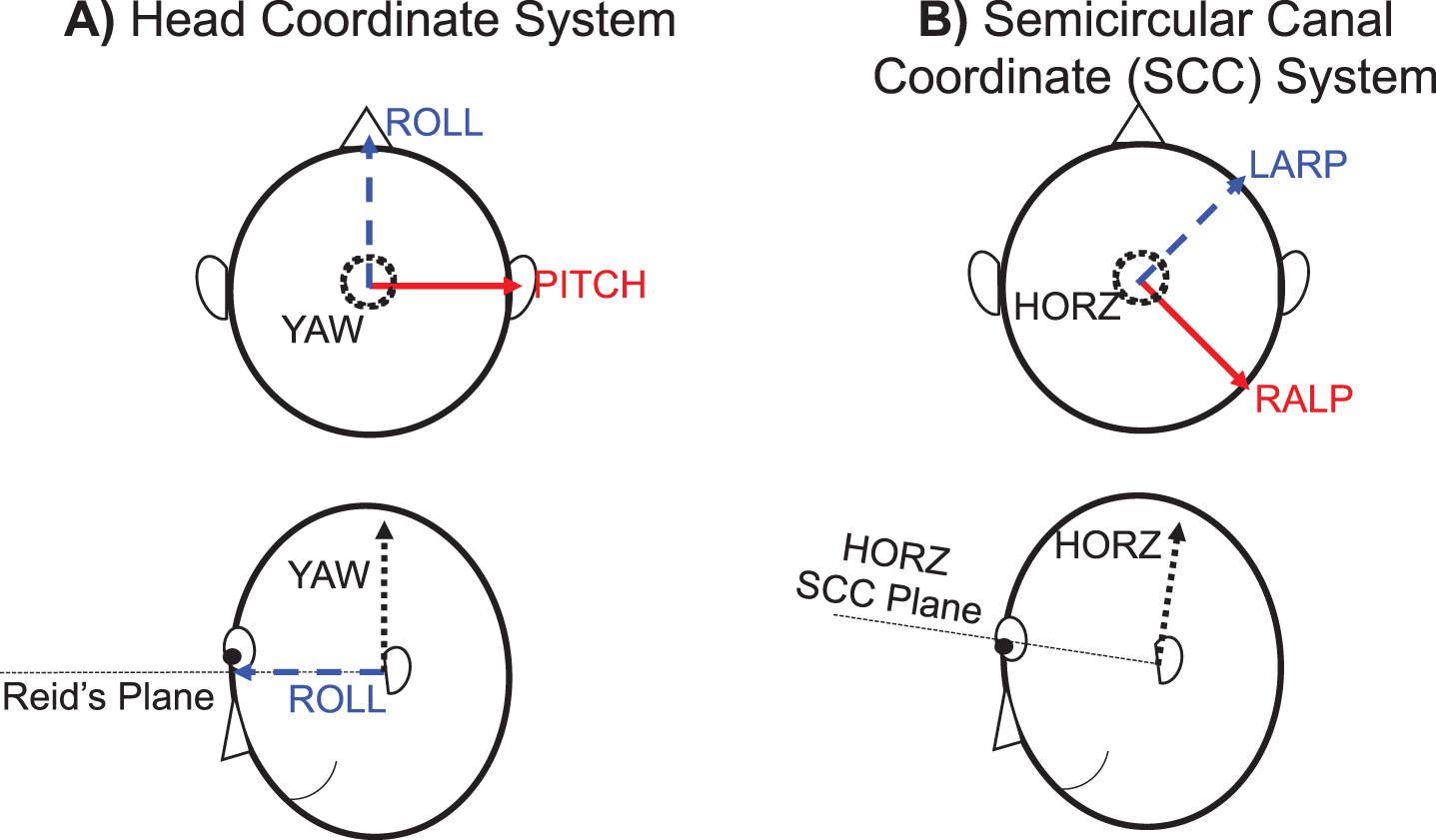
2.6Vestibular stimuli
Rotations in the three semicircular canal planes were found by rotating the head coordinate system to align with the semicircular canal orientations according to the following anatomical data. The semicircular canals were assumed to be pitched upwards 18.8° from Reid’s plane and yawed 45° from the sagittal plane, in accordance with Della Santina et al. [13] (Fig. 2B). The rotational velocities experienced in RALP, LARP, and horizontal canal planes were calculated by differentiating the rotational position time series for each plane.
The gravitoinertial accelerations experienced at the otoliths were determined using the calculated translational trajectories of each vestibular apparatus. The utricle and saccule were approximated at the same relative location with respect to the mid-ear. They were approximated as 38.4 mm lateral, 4.9 mm posterior, and 7.7 mm superior to the mid-ear, based on anatomical data [13]. The gravitoinertial acceleration magnitudes along the axes defined previously (Fig. 2A) were calculated using numerical double differentiation for both right and left otoliths. The gravitoinertial accelerations combine the stimuli from the linear acceleration of the head and the effects of gravity in the given direction. The peak-to-peak values in gravitoinertial accelerations over the course of the impulses were calculated. Only peak-to-peak accelerations in the vertical direction were calculated, as this is the direction in which accelerations may affect resultant vertical eye movements used to calculate VOR gain for the vertical semicircular canals.
2.7Analysis
Data from each impulse start to impulse end were interpolated to 100 time points. Impulse start and end points were identified as when the magnitude of the pitch component of the head velocity exceeded and fell below 10°/s, respectively. Each participant’s average impulse trajectory was found by averaging the interpolated data at each 1% of the impulse across the five impulses applied. Ensemble averages across the participant average trajectories were then taken at each 1% of the impulse (participant average trajectories illustrated in Figs. 3–7 over “% of impulse”). These inter-subject ensemble average trajectories were used to identify the average and standard deviations of features of interest (e.g. displacements, peak velocities, and peak-to-peak gravitoinertial accelerations). RA and LP impulses were analyzed separately.
Fig. 3
Ensemble averages of head rotations in the pitch, roll, and yaw planes for right anterior (RA) (A) and left posterior (LP) (B) impulses. Ensemble averages of head rotational velocities in the pitch, roll, and yaw planes for right anterior (C) and left posterior (D) impulses. Shading represents ± 1 standard deviation of inter-subject variability.
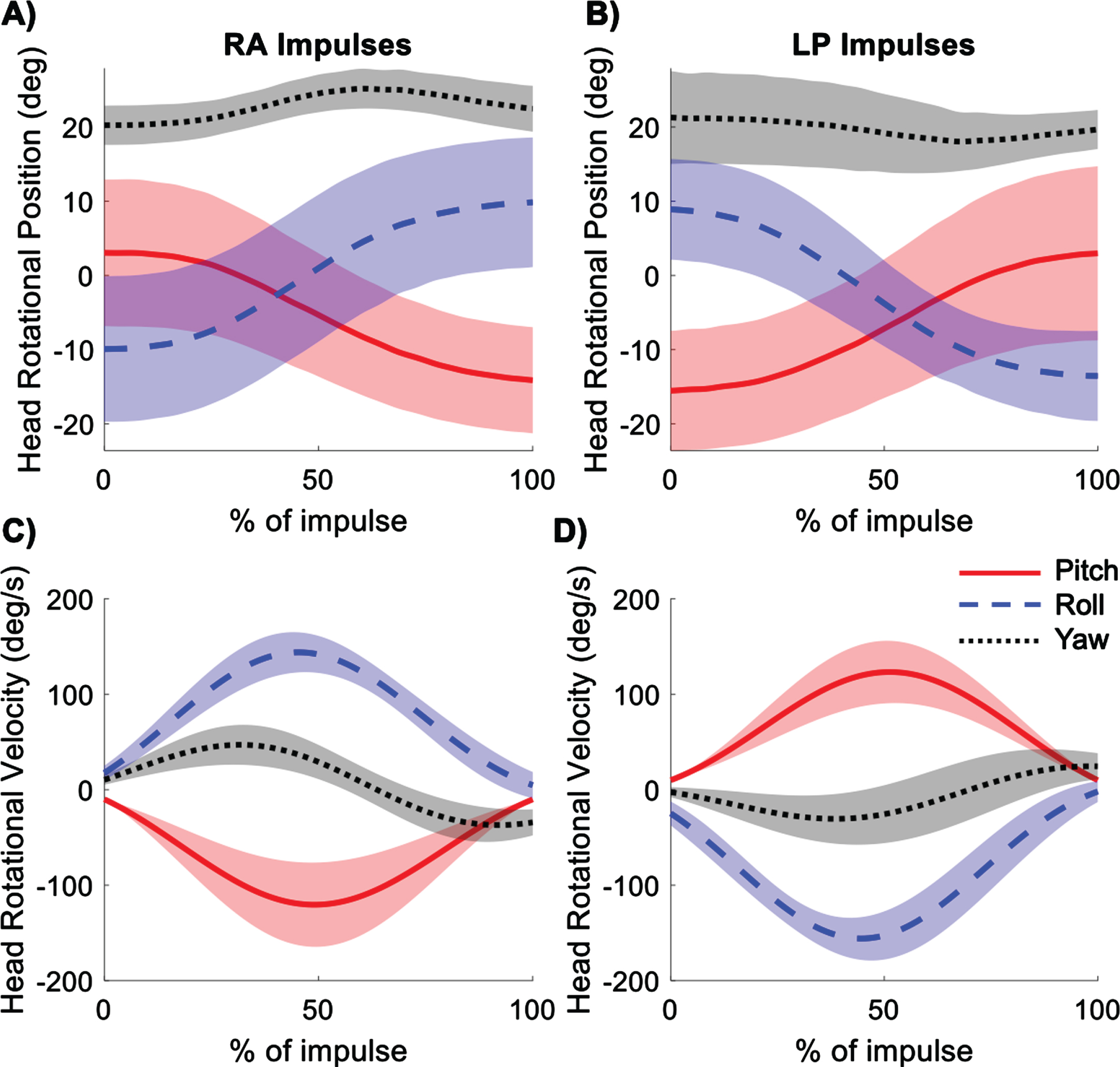
Fig. 4
Average displacement of the origin of the head coordinate system (midpoint between right and left ears) during right anterior (RA) (A) and left posterior (LP) (B) impulses. Shading represents ± 1 standard deviation of inter-subject variability.
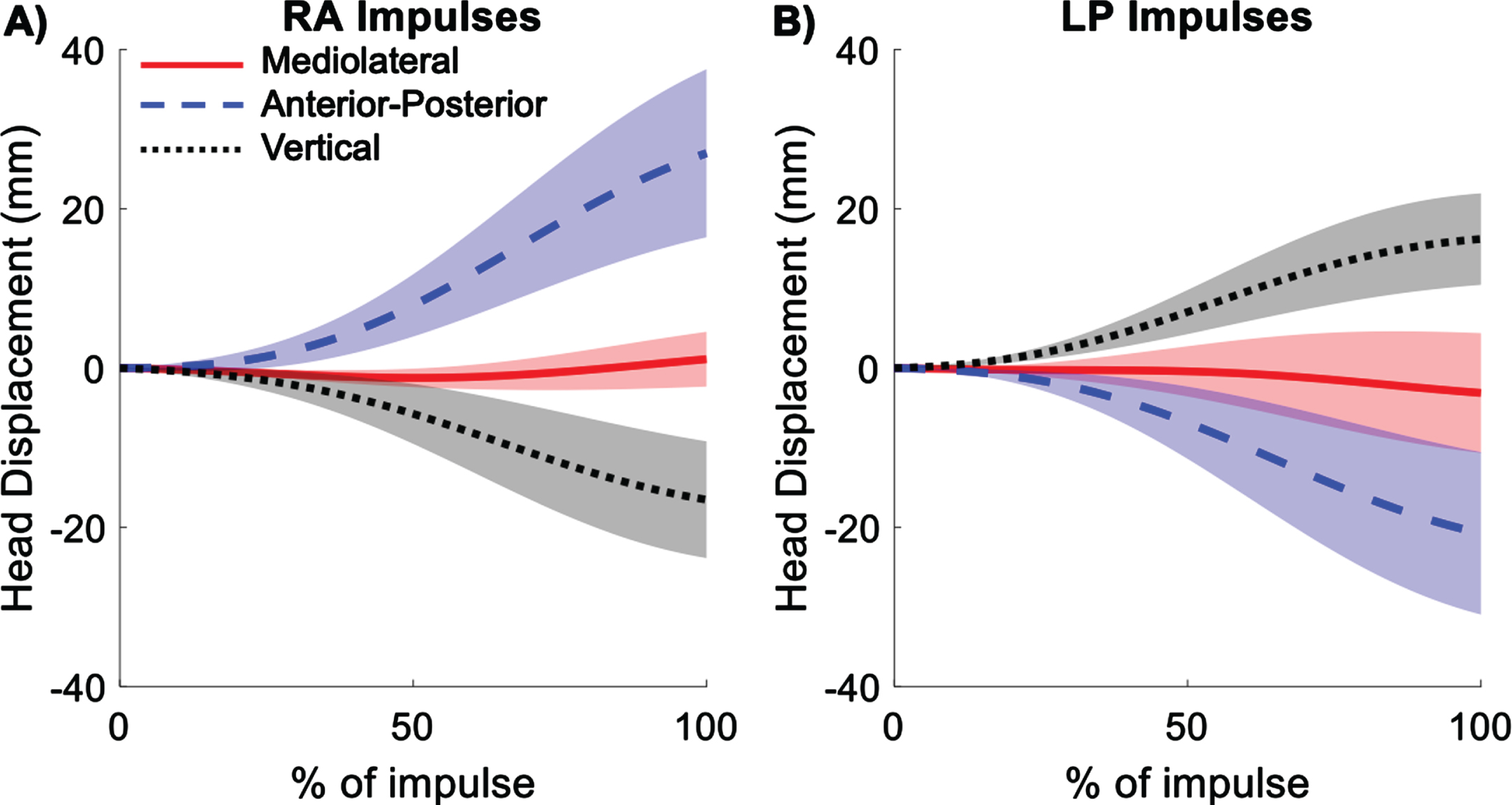
Fig. 5
Average displacement of the right and left orbits during right anterior (RA) (A) and left posterior (LP) (B) impulses. Shading represents ± 1 standard deviation of inter-subject variability.
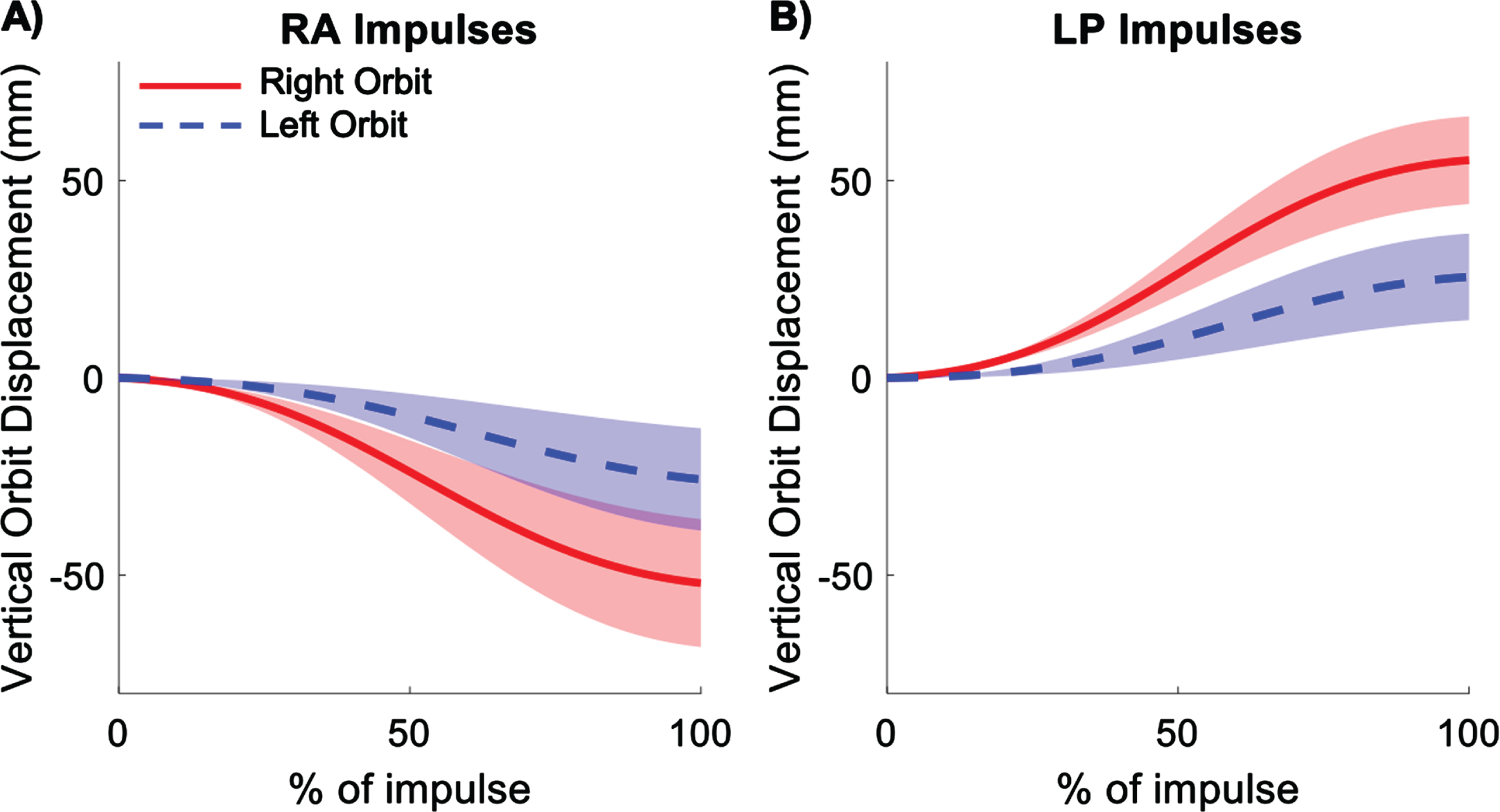
Fig. 6
Average rotational velocities in the semicircular canal (SCC) planes (RALP, LARP, and horizontal) for right anterior (RA) (A) and left posterior (LP) (B) impulses. Shading represents ± 1 standard deviation of inter-subject variability.
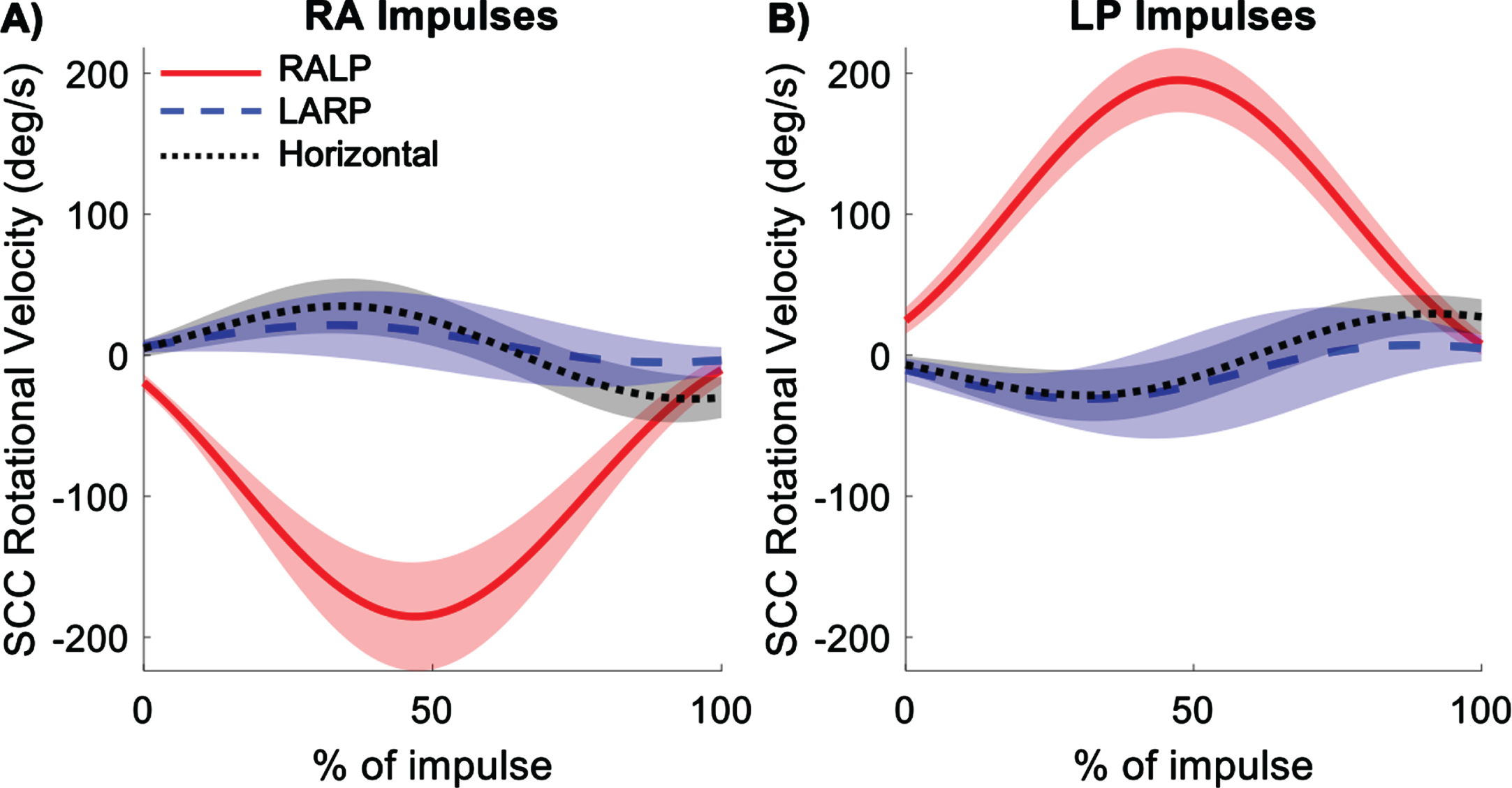
Fig. 7
Average gravitoinertial accelerations experienced by the right (red) and left (blue) otolith organs in the mediolateral (ML), anterior-posterior (AP), and vertical directions for right anterior (RA) (A, C, E) and left posterior (LP) (B, D, F) impulses. Shading represents ± 1 standard deviation of inter-subject variability.
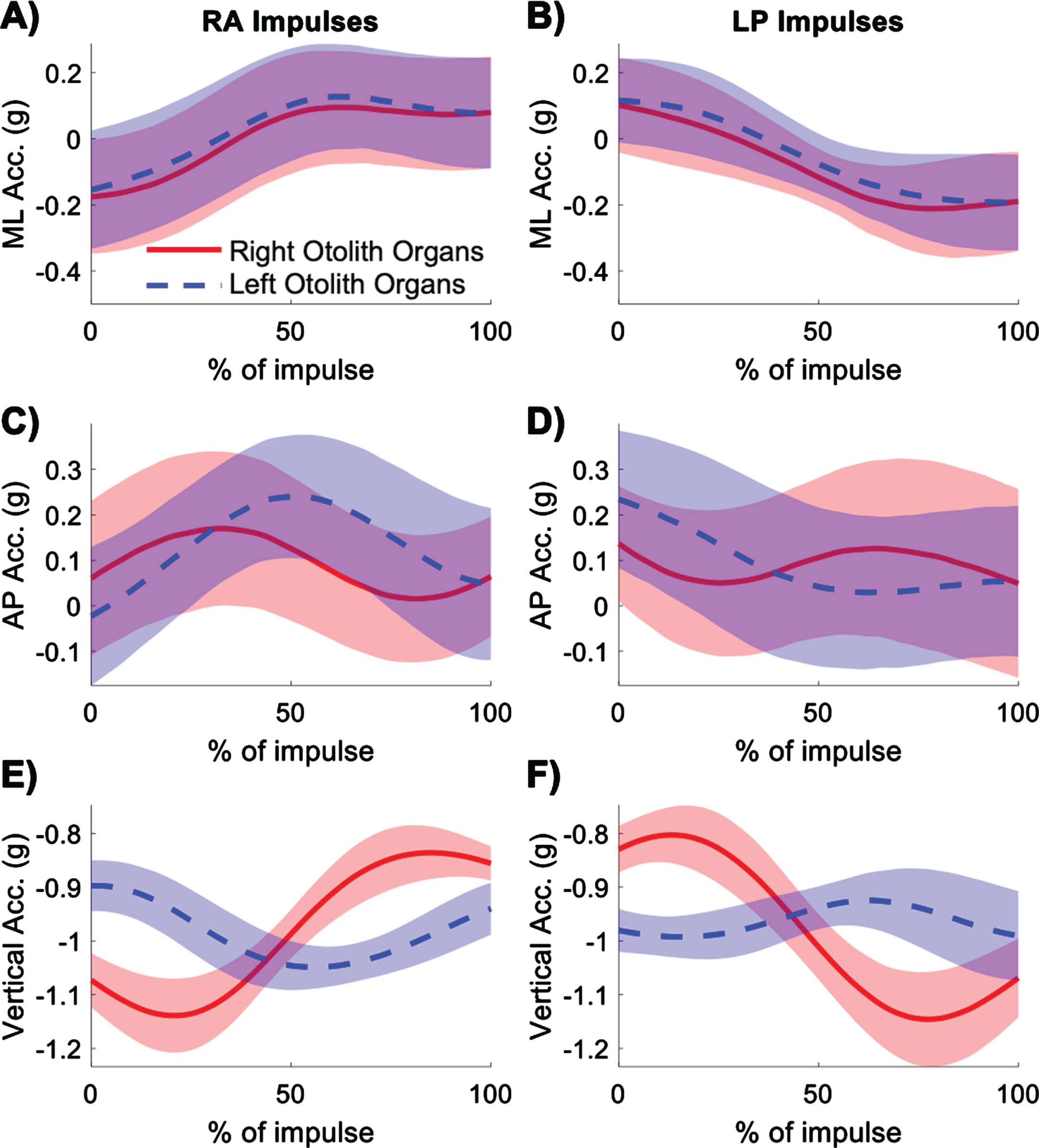
3Results
3.1Head rotation and translation
Head rotations were measured in pitch, roll, and yaw planes of the head coordinate system for the RA and LP impulses. The rotations were mostly in pitch and roll, as expected for movements in the RALP plane (Fig. 3). The excursions were approximately 20° in both pitch and roll with less than 5° excursion in yaw. The velocities in pitch and roll peaked at about 50% of the duration of the impulse for both RA and LP impulses, with the peak yaw at about 40% of the duration of the impulse. Peak velocities of the head are given in Table 1. Table 1 includes standard deviations indicating between-subject variability. The within-subject variability, particularly for the positional and gravitoinertial acceleration data, was generally lower than the between-subject variability reported in Table 1 and shown graphically in Figs. 3–7. Thus, the repeatability within subjects was much higher.
Table 1
Summary statistics for head kinematics and the resulting vestibular stimuli during right anterior (RA) and left posterior (LP) impulses
RA | LP | |
Peak Pitch Velocity (deg/s) | –120.9±44.4 | 124.4±33.8 |
Peak Roll Velocity (deg/s) | 145.7±21.9 | –159.3±21.7 |
Peak Yaw Velocity (deg/s) | 56.3±17.4 | –44.8±21.3 |
Mediolateral Head Displacement (mm) | 1.1±3.4 | –3.1±7.5 |
Anterior-Posterior Head Displacement (mm) | 27.0±10.6 | –20.7±10.2 |
Vertical Head Displacement (mm) | –16.5±7.3 | 16.2±5.7 |
Right Orbit Vertical Displacement (mm) | –55.5±15.5 | 58.9±6.9 |
Left Orbit Vertical Displacement (mm) | –29.2±11.0 | 29.1±7.7 |
Peak RALP Velocity (deg/s) | –186.6±35.6 | 197.5±24.0 |
Peak LARP Velocity (deg/s) | 33.9±12.6 | –45.2±15.5 |
Peak Horizontal Velocity (deg/s) | 43.2±16.4 | –40.0±13.4 |
Right Otolith peak-to-peak Vertical Acceleration (g) | 0.30±0.16 | 0.34±0.13 |
Left Otolith peak-to-peak Vertical Acceleration (g) | 0.08±0.14 | 0.09±0.12 |
Translations of the head from the initial starting location were computed for the mid-ear origin. Most of the translation of the mid-ear occurred in the anterior-posterior direction, with some translation in the vertical direction, and very little translation in the mediolateral direction (Fig. 4). For RA impulses, the head translated forward, downward, and slightly toward the right. The opposite occurred for LP impulses, where the head translated backward, upward, and slightly leftward. Translations in all three directions are given in Table 1.
For both RA and LP impulses, the right orbit displacements were greater in magnitude than the left orbit displacements (Fig. 5). For RA impulses, both orbits moved downward and for LP impulses, both orbits moved upward. Vertical displacements of the right and left orbits are given in Table 1.
3.2Vestibular stimuli
As expected, the highest magnitude semicircular canal plane velocities occurred in the RALP plane (Fig. 6). However, non-negligible velocities occurred in the LARP and horizontal planes. Peak RALP plane velocities occurred at about 50% of the duration of the impulse. The rotational velocity profiles in all three semicircular canal planes were approximately equal in magnitude but opposite in direction for RA and LP impulses. Peak velocities in the semicircular canal planes are given in Table 1.
The gravitoinertial accelerations experienced by the right and left otolith organs along the anatomical head axes showed similar patterns in the mediolateral direction during both RA and LP impulses (Fig. 7). The right and left otoliths experienced rightward acceleration for RA impulses and leftward acceleration for LP impulses. In the anterior-posterior direction, the right otolith organs experienced a peak in forward acceleration at the start of RA impulses and a peak in backward acceleration at the start of LP impulses. The left otolith organs showed these acceleration peaks slightly later in the trajectory of the impulse compared to the right otolith organs. For RA impulses, in the vertical direction, the right otolith organs experienced initial downward followed by upward acceleration, whereas the left otolith organs experienced upward followed by downward acceleration. The opposite trend is seen for LP impulses. The left otolith organs experienced smaller accelerations than the right otolith organs in the vertical direction. The magnitude of the peak-to-peak vertical gravitoinertial accelerations at the otoliths are given in Table 1.
4Discussion
This study describes the kinematics of the head during protocols designed to assess the vertical semicircular canals with the vHIT and the resulting rotational stimuli to the semicircular canals and linear accelerations to the otolith organs. Non-target semicircular canal plane velocities reached approximately 20% of those in the target canal plane, even when performed by a trained clinician. For RALP plane impulses, the right orbit and right otolith organs underwent larger displacements and accelerations than the left orbit and left otolith organs, which may explain different rotations observed for right and left eyes [24].
The RALP plane impulses resulted in head rotations that were approximately equal in roll and pitch. The head moved through approximately 20° excursions and attained peak velocities between 120°/s and 160°/s in roll and pitch for both RA and LP impulses. Smaller excursions and peak velocities occurred in yaw. These high velocities and large ranges of motion were attained in this young population with good neck mobility. In testing older populations with reduced neck mobility and range of motion, these excursions and peak velocities are likely to be reduced. Future work should consider investigating how impulses of sufficient velocity can best be applied in populations with reduced neck range of motion.
For RALP impulses, there was a large difference in linear displacement between the right and left eyes, with the right orbit undergoing greater vertical displacement than the left orbit. Previous work has suggested that the opposite is true for LARP impulses [24]. This difference can be attributed to the off-axis rotation of the orbits, with the left orbit located much closer to the axis of rotation than the right orbit for RALP impulses [24]. Because the orbits translate differently in space, the eyes need to rotate different amounts to remain fixated on a visual target. For RALP impulses, the larger vertical displacements of the right orbit would theoretically lead to a greater rotation of the right eye to remain fixated on the target. The greater rotation and corresponding greater rotational velocity of the right eye would have a higher signal to noise ratio than the left eye because of its greater velocity (i.e., signal) and presumably equivalent measurement error between eyes (i.e., noise). However, the stimulus of interest in the vHIT is the ocular movement response to rotational head velocity (i.e., stimulus to the semicircular canals) and not to linear head translations and accelerations (i.e., stimulus to the otolith organs). While using the left eye rotation may reduce linear translations and be more physiologically appropriate, the lower signal to noise ratio may lead to poorer estimates of the VOR gain. This reasoning is from assumptions of the required eye rotations in the head, as opposed to the actual eye rotation data. Including eye responses is outside of the scope of this paper, but will be a point of focus in future work. Regardless, the data shown in this paper already suggest that the selection of which eye to use when calculating the VOR gain could significantly influence clinical results. Certain vHIT devices only measure right eye movements, which may impact the gains and cause systematic differences between those for RALP versus LARP [24]. Future work should be done to identify which eye should be used to reliably calculate VOR gain.
The largest head rotational velocities occurred in the plane of interest (RALP plane); however, non-negligible velocities occurred in the LARP and horizontal planes as well, with off-plane movement velocities up to 20% of the target velocity. This demonstrates the difficulty of selectively stimulating the semicircular canal of interest, likely due to the complexity of the cervical spine biomechanics. Since these off-plane movements will inevitably occur, it is important to determine how large these off-plane velocities can be before they significantly affect the reliability of the results. Previous normative data shows that there is approximately 5–7° of inter-subject variability in semicircular canal orientation [13]. The data analysis for this paper assumed average semicircular canal orientation. Subject deviation from average orientation may have had some effect on the assumed stimulation of the semicircular canals.
As the objective of the vHIT is to determine the gain of the eye responses to semicircular canal stimulation, input from the otolith organs should be minimized. In the case of the vertical vHIT, the vertical acceleration stimuli to the otolith organs should be minimized to reduce the potential influence of the otolithic-ocular reflex on the resultant eye movements [10, 14]. For RALP impulses, the right otolith organs experienced larger changes in vertical gravitoinertial accelerations than the left otolith organs. Quantifying otolithic contribution to resultant eye rotation can be challenging [7, 10, 14]. However, this may be an important consideration for recommending standardized protocols for performing and interpreting vertical vHIT results.
While this study has provided foundational data on the head and vestibular kinematics occurring during the vertical vHIT, the following limitations must be considered. First, only six young healthy adults were tested. This sample size is small and includes individuals without neck mobility limitations, which are likely to be more prevalent in an older population. Dizziness is the number one reason people over the age of 70 seek the care of a physician [22]. Therefore, an older population should be considered in future work. However, the small sample size still provides valuable information describing head and vestibular kinematics during vertical semicircular canal impulses. Documenting head kinematics and vestibular stimuli in a population without the complications of neck mobility is an important initial step. This study is also limited by only including one examiner. As some aspects of the trajectories reported here may be attributed to the personal tendencies of the examiner, future studies should include multiple examiners, preferably of different experience levels.
This study describes the head kinematics and vestibular stimuli experienced during vertical semicircular canal impulses. These results demonstrate the complexities of the head movement, differential movement of the right and left orbits, and the combined effects of the rotation and displacement of the head on vestibular end organs. The kinematic data provided should engender future research studies that address remaining questions regarding the use of the vHIT to assess the vertical semicircular canals, including the required precision of the impulses and which eye to use when calculating VOR gain.
Acknowledgments
This work was funded in part by the Telemedicine and Advanced Technology Research Center (TATRC) at the United States Army Medical Research and Development Command through the Advanced Medical Technology Initiative (AAMTI) and the National Institutes of Health (F31DC020110). The content is solely the responsibility of the authors and does not necessarily represent the official views of the National Institutes of Health. The authors thank Richard Smith for help with data collection.
References
[1] | Abrahamsen E.R. , Christensen A.-E. and Hougaard D.D. , Intra- and Interexaminer Variability of Two Separate Video Head Impulse Test Systems Assessing All Six Semicircular Canals, Otology & Neurotology 39: ((2018) ), e113–e122. |
[2] | Agrawal Y. , Schubert M.C. , Migliaccio A.A. , Zee D.S. , Schneider E. , Lehnen N. and Carey J.P. , Evaluation of quantitative head impulse testing using search coils versus video-oculography in older individuals, Otology & Neurotology: Official Publication of the American Otological Society, American Neurotology Society [and] European Academy of Otology and Neurotology 35: ((2014) ), 283. |
[3] | Alhabib S.F. and Saliba I. , Video head impulse test: a review of the literature, Eur Arch Otorhinolaryngol 274: ((2017) ), 1215–1222. |
[4] | Au–Hougaard D.D. and Au –Abrahamsen E.R. , Testing of all Six Semicircular Canals with Video Head Impulse Test Systems, JoVE (2019), e59012. |
[5] | Bartolomeo M. , Biboulet R. , Pierre G. , Mondain M. , Uziel A. and Venail F. , Value of the video head impulse test in assessing vestibular deficits following vestibular neuritis, Eur Arch Otorhinolaryngol 271: ((2014) ), 681–688. |
[6] | Bell S.L. , Barker F. , Heselton H. , MacKenzie E. , Dewhurst D. and Sanderson A. , A study of the relationship between the video head impulse test and air calorics, Eur Arch Otorhinolaryngol 272: ((2015) ), 1287–1294. |
[7] | Bronstein A. and Gresty M. , Short latency compensatory eye movement responses to transient linear head acceleration: a specific function of the otolith-ocular reflex, Experimental Brain Research 71: ((1988) ), 406–410. |
[8] | Bronstein A.M. , Patel M. and Arshad Q. , A brief review of the clinical anatomy of the vestibular-ocular connections-how much do we know? Eye (Lond) 29: ((2015) ), 163–170. |
[9] | Bronstein A.M. , Patel M. and Arshad Q. , A brief review of the clinical anatomy of the vestibular-ocular connections—how much do we know? Eye 29: ((2015) ), 163–170. |
[10] | Castro P. , Esteves S.S. , Lerchundi F. , Buckwell D. , Gresty M.A. , Bronstein A.M. and Arshad Q. , Viewing target distance influences the vestibulo-ocular reflex gain when assessed using the video head impulse test, Audiology and Neurotology 23: ((2018) ), 285–289. |
[11] | Cleworth T.W. , Carpenter M.G. , Honegger F. and Allum J.H. , Differences in head impulse test results due to analysis techniques, Journal of Vestibular Research 27: ((2017) ), 163–172. |
[12] | Cremer P.D. , Halmagyi G.M. , Aw S.T. , Curthoys I.S. , McGarvie L.A. , Todd M.J. , Black R.A. and Hannigan I.P. , Semicircular canal plane head impulses detect absent function of individual semicircular canals, Brain: A Journal of Neurology 121: ((1998) ), 699–716. |
[13] | Della Santina C.C. , Potyagaylo V. , Migliaccio A.A. , Minor L.B. and Carey J.P. , Orientation of human semicircular canals measured by three-dimensional multiplanar CT reconstruction, Journal of the Association for Research in Otolaryngology 6: ((2005) ), 191–206. |
[14] | Gresty M.A. and Bronstein A.M. , Otolith stimulation evokes compensatory reflex eye movements of high velocity when linear motion of the head is combined with concurrent angular motion, Neuroscience Letters 65: ((1986) ), 149–154. |
[15] | Halmagyi G.M. , Chen L. , MacDougall H.G. , Weber K.P. , McGarvie L.A. and Curthoys I.S. , The Video Head Impulse Test, Frontiers in Neurology 8: ((2017) ). |
[16] | Kitazawa M. , Morita Y. , Yagi C. , Takahashi K. , Ohshima S. , Yamagishi T. , Izumi S. , Koizuka I. and Horii A. , Test Batteries and the Diagnostic Algorithm for Chronic Vestibular Syndromes, Front Neurol 12: ((2021) ), 768718. |
[17] | MacDougall H. , Weber K. , McGarvie L. , Halmagyi G. and Curthoys I. , The video head impulse test: diagnostic accuracy in peripheral vestibulopathy, Neurology 73: ((2009) ), 1134–1141. |
[18] | Macdougall H.G. , McGarvie L.A. , Halmagyi G.M. , Curthoys I.S. and Weber K.P. , The video Head Impulse Test (vHIT) detects vertical semicircular canal dysfunction, PLoS One 8: ((2013) ), e61488. |
[19] | MacDougall H.G. , Weber K.P. , McGarvie L.A. , Halmagyi G.M. and Curthoys I.S. , The video head impulse test: diagnostic accuracy in peripheral vestibulopathy, Neurology 73: ((2009) ), 1134–1141. |
[20] | Mangabeira Albernaz P.L. and Zuma E.M.F.C. , The video head impulse test, Acta Otolaryngol 134: ((2014) ), 1245–1250. |
[21] | McGarvie L.A. , Martinez-Lopez M. , Burgess A.M. , MacDougall H.G. and Curthoys I.S. , Horizontal Eye Position Affects Measured Vertical VOR Gain on the Video Head Impulse Test, Frontiers in Neurology 6: ((2015) ). |
[22] | Sloane P.D. , Dizziness in primary care. Results from the National Ambulatory Medical Care Survey, J Fam Pract 29: ((1989) ), 33–38. |
[23] | van Esch B.F. , Nobel-Hoff G.E. , van Benthem P.P. , van der Zaag-Loonen H.J. and Bruintjes T.D. , Determining vestibular hypofunction: start with the video-head impulse test, Eur Arch Otorhinolaryngol 273: ((2016) ), 3733–3739. |
[24] | Wittmeyer Cedervall L. , Magnusson M. , Karlberg M. , Fransson P.-A. , Nyström A. and Tjernström F. , vHIT Testing of Vertical Semicircular Canals With Goggles Yield Different Results Depending on Which Canal Plane Being Tested, Frontiers in Neurology 12: ((2021) ). |
[25] | Yacovino D.A. , Zanotti E. and Cherchi M. , The spectrum of acute vestibular neuropathy through modern vestibular testing: A descriptive analysis, Clin Neurophysiol Pract 6: ((2021) ), 137–145. |
[26] | Zatsiorsky V.M. and Zaciorskij V.M. , Kinetics of Human Motion, Human kinetics, 2002. |