Flavonoids as an Intervention for Alzheimer’s Disease: Progress and Hurdles Towards Defining a Mechanism of Action1
Abstract
Attempts to develop a disease modifying intervention for Alzheimer’s disease (AD) through targeting amyloid β (Aβ) have so far been unsuccessful. There is, therefore, a need for novel therapeutics against alternative targets coupled with approaches which may be suitable for early and sustained use likely required for AD prevention. Numerous in vitro and in vivo studies have shown that flavonoids can act within processes and pathways relevant to AD, such as Aβ and tau pathology, increases in BDNF, inflammation, oxidative stress and neurogenesis. However, the therapeutic development of flavonoids has been hindered by an ongoing lack of clear mechanistic data that fully takes into consideration metabolism and bioavailability of flavonoids in vivo. With a focus on studies that incorporate these considerations into their experimental design, this review will evaluate the evidence for developing specific flavonoids as therapeutics for AD. Given the current lack of success of anti-Aβ targeting therapeutics, particular attention will be given to flavonoid-mediated regulation of tau phosphorylation and aggregation, where there is a comparable lack of study. Reflecting on this evidence, the obstacles that prevent therapeutic development of flavonoids will be examined. Finally, the significance of recent advances in flavonoid metabolomics, modifications and influence of the microbiome on the therapeutic capacity of flavonoids in AD are explored. By highlighting the potential of flavonoids to target multiple aspects of AD pathology, as well as considering the hurdles, this review aims to promote the efficient and effective identification of flavonoid-based approaches that have potential as therapeutic interventions for AD.
ALZHEIMER’S DISEASE: THE NEED FOR NOVEL THERAPEUTICS
At the time of writing, there are at least 50 million people living with dementia worldwide [1]. Due to increasing life expectancies, this is predicted to triple by 2050, and yet there are still no therapeutics capable of preventing or slowing the onset of dementia. The most common cause of dementia, Alzheimer’s disease (AD), is a neurodegenerative disease that is traditionally characterized by deposition of amyloid plaques and neurofibrillary tangles (NFTs), caused by amyloid β peptide (Aβ) and tau aggregation respectively. More recently, chronic neuroinflammation and gliosis have joined plaques and tangles as hallmarks of AD. One of the major obstacles to preventing AD progression is that by the time a clinical diagnosis has been given, irreversible brain atrophy has already occurred (Fig. 1), and pathological cascades are well developed.
Fig. 1
Flavonoids can act as multi-modal inhibitors of AD pathology. (A) APP is processed in two pathways. In the non-amyloidogenic pathway, APP is cleaved by α-secretase (ADAM10) to produce α-C-terminal fragment (α-CTF) and secretory APP (sAPPα) whereas the pro-amyloidogenic pathway involves cleavage of APP β-secretase (BACE1) to produce β-CTF and sAPPβ. α-CTF and β-CTF is then cleaved by γ-secretase to release P3 and Aβ, respectively, as well as the APP intracellular domain (AICD). Flavonoids have been shown to inhibit βγ-secretase processing as well as promoting α-secretase processing. This causes a shift towards the non-amyloidogenic pathway and reduces the levels of Aβ produced. (B) Aβ can self-aggregate to form oligomers and eventually amyloid plaques. Flavonoids may be able to inhibit the formation of amyloid plaques by binding to Aβ and inhibiting aggregation or promoting the formation of non-toxic off-target oligomers. (C) Toxic Aβ monomers and oligomers have been shown to induce microglial activation and proliferation. Animal models have shown that flavonoid intervention can reduce the levels of gliosis in the brain. (D) Activated microglia secrete pro-inflammatory cytokines such as IL-1β and IL-6. Several flavonoids have been shown to reduce the levels of these cytokines in vivo. (E) The microtubule (MT) associated protein tau, which is predominantly located in the axon, is hyperphosphorylated in AD, perhaps as a result of pro-inflammatory cytokine release. This causes the dissociation of tau from the microtubule and mislocalisation to the somatodendritic region. Flavonoids can inhibit several kinases associated with tau phosphorylation, as well as attenuating the proinflammatory response. Therefore, flavonoids have the potential to reduce tau phosphorylation. (F) Hyperphosphorylated tau can self-aggregate to form toxic oligomers and eventually neurofibrillary tangles (NFT). There is evidence that flavonoids can bind to tau and inhibit its aggregation or promote the formation of non-toxic oligomers. (G) Hyperphosphorylated tau can mislocalise to post-synaptic terminals. Synaptic tau and Aβ can cause synaptic dysfunction (H) and eventual synapse loss (I). Flavonoids have been shown to upregulate BDNF to promote adult hippocampal neurogenesis and synaptogenesis. Upregulation of BDNF may, therefore, prevent the loss of synapses and the consequent loss of neurons (J).
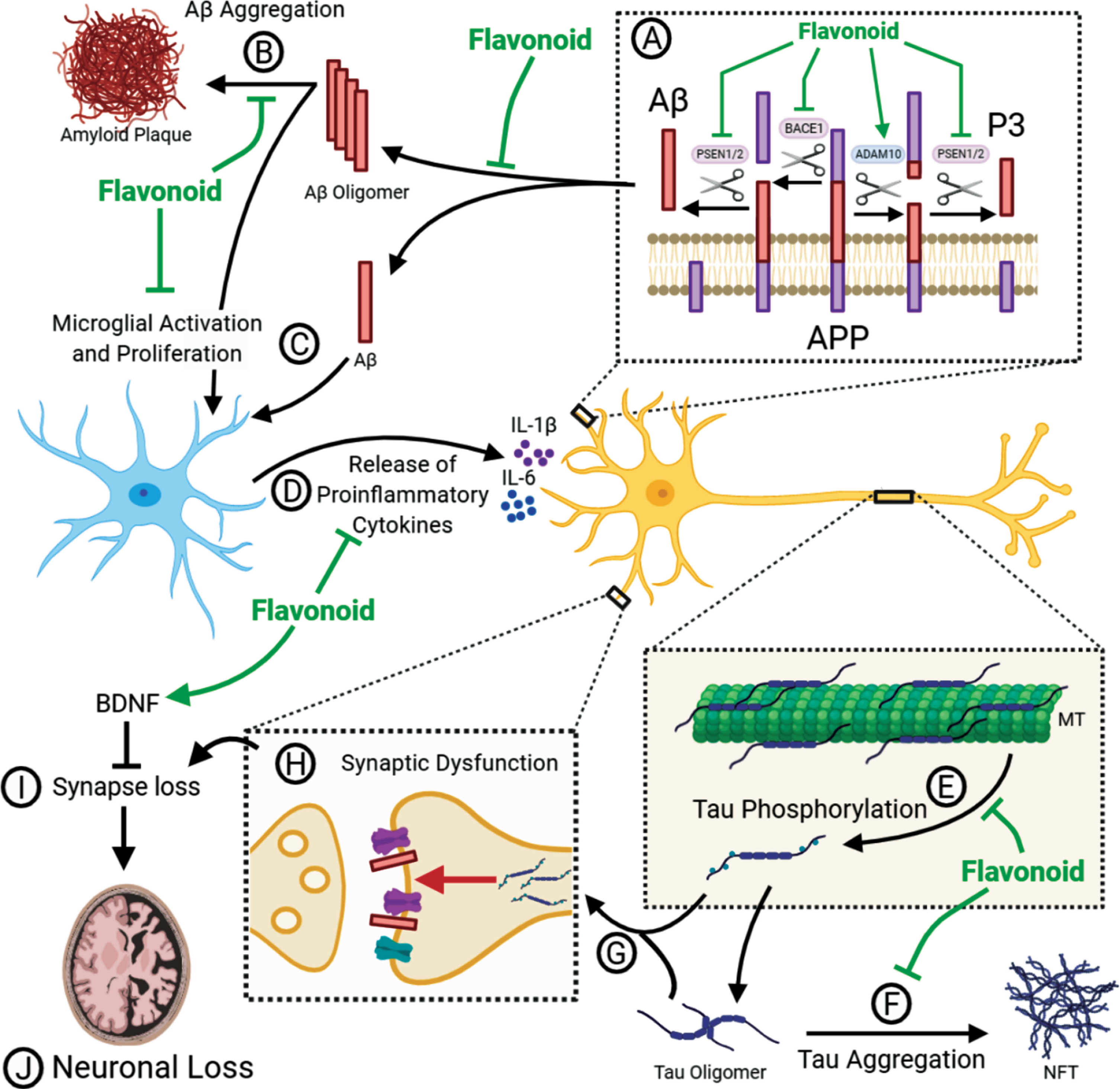
The amyloid cascade hypothesis (ACH) postulates that it is the accumulation of Aβ oligomers that initiates a downstream cascade causing neuroinflammation, tau-induced toxicity, synaptic dysfunction and neuronal loss [2]. Support for this hypothesis comes from the identification that mutations in the amyloid precursor protein (APP), presenilin-1 (PS1) [3, 4] and presenilin-2 (PS2) [5, 6], which either enhance the accumulation of Aβ or promote an increased ratio of the longer, more aggregation prone Aβ1–42 over Aβ1–40 [7–9], are sufficient to cause early onset familial AD (FAD) [10]. APP can be processed in either a non-amyloidogenic pathway, driven by the α-secretase enzyme ADAM10, or in a pro-amyloidogenic pathway involving the β-secretase enzyme BACE1. Following cleavage by either of these enzymes, there is a second cleavage event by γ-secretase which yields either p3 (non-amyloidogenic) or Aβ (pro-amyloidogenic) (Fig. 1A). Presenilin forms the catalytic subunit of the γ-secretase enzyme complex [11]. According to the ACH, the failure to clear Aβ1–42 initiates consequent pathological pathways that lead to the onset of dementia [2]. This hypothesis has been the foundation for the development of many Aβ-targeting therapeutics, with the idea that by targeting this initial Aβ accumulation, the resulting toxic pathways can be inhibited.
Disappointingly, therapeutics targeting APP processing directly, such as BACE1 inhibitors, have failed at Phase III [12], likely due to off target effects [13]. Moreover, methods to reduce soluble and/or aggregated forms of Aβ via antibody strategies have had disappointing outcomes [14, 15], although, it should be noted that recent reports claim that the Aβ-targeting antibody Aducanumab meets its primary endpoint. It is becoming increasingly clear that reducing the levels of Aβ in the brain is unlikely to provide significant clinical benefit when delivered at the stage of mild cognitive impairment (MCI) or later. It is likely that, in order to be maximally effective, the use of Aβ-targeting therapeutics will be required from the start of, and throughout, the long prodromal phase of AD. This limitation necessitates a strategic shift, either switching attention to targets that correlate better with later stage disease progression, such as neuroinflammation or tau, or sticking with the existing targets but putting the focus on biomarker development to enable better patient stratification for earlier intervention. An additional approach is to identify and validate safe and cost-effective lifestyle interventions which could be widely implemented throughout mid-life to reduce AD risk at a population level.
Flavonoid rich diets: Impact on cognitive decline and AD
Epidemiology has long suggested that diets rich in polyphenols, such as the Mediterranean diet, might slow age-related cognitive decline and some studies indicate reduced risk of dementia [16, 17]. Moreover, consumption of flavonoids, a large family of dietary polyphenol compounds, decreases cognitive decline with aging [18, 19]. Although direct evidence of a reduction in AD is lacking, the available epidemiological data does suggest that flavonoid consumption has the potential to ameliorate AD pathology and provide symptomatic benefit.
There are more than 5000 types of flavonoid, and they are found almost ubiquitously in plants and thus are widely available in the human diet. The six main subclasses of flavonoids are: anthocyanidins, flavones, isoflavones, flavonols, flavanones, and flavan-3-ols (flavanols) (Fig. 2). While flavonoids are well known for their ability to act as antioxidants, it is clear that they are also capable of regulating intracellular responses principally through modulation of protein kinase signalling pathways [20]. Furthermore, there is now a substantial and growing body of evidence supporting the ability of flavonoids to interfere in AD-related pathways [21].
Fig. 2
The flavonoid backbone and the six main subgroups with examples from each.
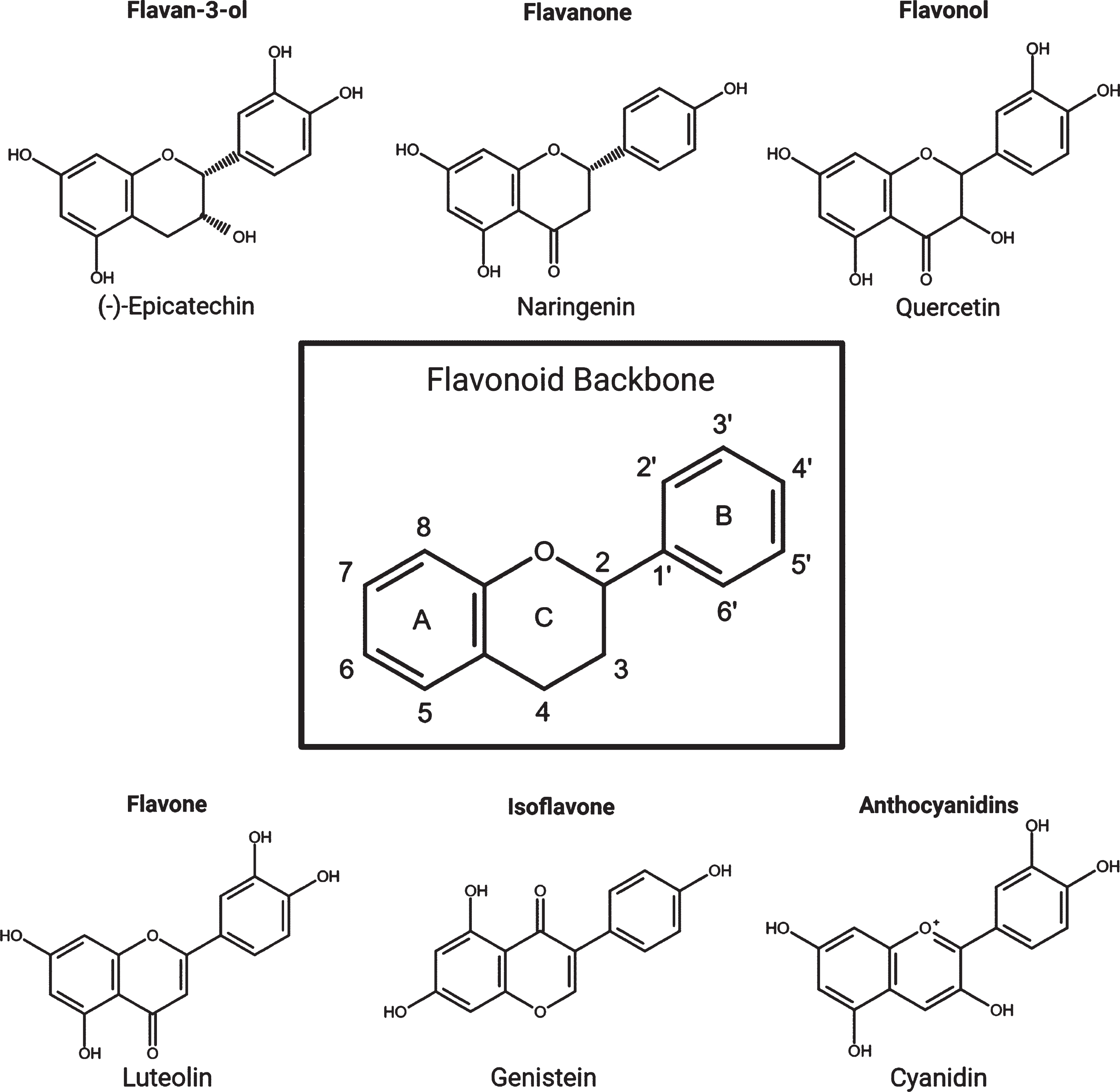
However, the development of flavonoids as therapeutic interventions for AD has been hindered by considerable uncertainty surrounding bioavailability, metabolism and basic pharmacokinetics. In this review emerging mechanistic evidence for flavonoid-mediated modulation of AD pathways is evaluated, with a focus on studies where genuine consideration has been given to the bioavailability of flavonoids. The metabolism and bioavailability of flavonoids in both humans and animal models, including the increasingly evident role of the gut-microbiome to these outcomes will be highlighted. Considering this, approaches to improve the translatability of flavonoids into therapeutics for AD will be discussed. Finally, we will examine whether supplement or more conventional drug design approaches based on flavonoid scaffolds represents the best way forward.
INHIBITING AD PATHOLOGY WITH FLAVONOIDS: MECHANISMS OF ACTION
There are a very large number of studies describing protective effects of various polyphenols against AD-relevant insults following their administration to cell lines but, unfortunately, we are unable to include them all here. Likewise, several studies show that flavonoids can act as acetylcholinesterase (AChE) inhibitors but, as AChE inhibitors are symptomatic interventions and not preventative or disease modifying, they will not be discussed here (for review see [22]). A major advance in the last decade has been the investigation of flavonoids in mouse models of AD. Here, the analysis of these in vivo studies will be mainly limited to those that have employed an oral route of polyphenol administration in drinking water or food, as well as via oral gavage; a summary of the outcomes of those studies that used oral methods of delivery is shown in Table 1. The doses investigated range from 1–500 mg/kg/day which in humans would be equivalent to 0.07–35 g intake for a 70 kg adult. While little is known about the exact binding partners of most flavonoids, common molecular mechanisms have emerged which impact on Aβ and tau pathology, neuroinflammation, oxidative stress and neurogenesis (Fig. 1).
Table 1
A summary of the results of flavonoid interventions in AD mouse models. Only interventions that used an oral method of delivery such as in water, diet, oral gavage (O.g.) or intragastric administration (I.g.) were included. The method of delivery was classified as ‘orally’ where further detail was not supplied. Treatment times of 28–30 days were rounded to 1 month. A decrease in Aβ pathology was classified as a decrease in the levels of soluble or insoluble Aβ1–40 or Aβ1–42, a reduction in visible Aβ plaques or a shift in APP processing. A decrease in gliosis involved decreased levels of microglia or astroglia. Pro-inflammatory markers included iNOS, COX2, NF-κB, TNF-α, M-CSF ICAM-1, TLR4, NLRP3, IL-16, IL-1β, IL-6, IL-17A, IL-12p70 and the JAK2/STAT3 pathway. Oxidative stress markers included Nrf2, SOD1, GPx, GSH, H2O2, MDA, CAT or HO-1. Synaptic markers included PSD95, SNAP23, SNAP25, Arc, Homer, Synaptotagmin, Synapsin, Spinophilin, Gephyrin, Synaptophysin and glutamatergic receptor subunits. Evidence for pro-survival/neurogenesis included increases in BDNF, activation of the TrkA pathway and inhibition of p75NTR pathway as well as decreased neuronal apoptosis and corresponding markers such as caspase-3. Improvement in the Morris Water Maze was classed as a reduced escape latency, an increased time spent in the target quadrant and an increase in the number of platform crossings. () A significant effect was found; (cross)no significant effect was found; (–) this variable was not investigated. * Oxidative stress was identified as a decrease in anti-oxidative enzymes
Flavonoid | Mouse Model | Concentration (mg/kg/day) | Duration | Method of Delivery | ↓β-Amyloid Pathology | ↓p-Tau | ↓Gliosis | ↓Pro-inflammatory Markers | ↓ Oxidative stress | ↑ Synaptic Markers | ↑Pro-Survival Neurogenesis | Improved performance on MWM | Reference |
7,8-DHF | 5XFAD | 5 | 2–6 months | Orally | ✓ | – | – | – | – | ✓ | ✓ | ✓ | [23] |
Tg2576 (APPswe) | 7 | 9–10 months | Water | ✗ | – | – | – | – | ✓ | ✓ | ✓ | [77] | |
Apigenin | APPswe/PS1dE0 | 40 (5 days a week) | 4–7 months | O.g. | ✓ | – | – | – | ✓ | – | ✓ | ✓ | [24] |
Baicalein | APPswe/PS1dE9 | 40 | 5–7 months | Water | – | – | – | – | – | – | – | ✓ | [25] |
80 | ✓ | ✓ | – | ✓ | – | ✓ | – | ✓ | |||||
Baicalin | APPswe/PS1dE9 | 100 | 8–9 months (sacrificed at 10months) | O.g. | ✗ | – | ✓ | ✓ | – | – | – | – | [163] |
ICR injected with Aβ42 into hippocampus | 30 | 2 weeks after Aβ42 injection | O.g. | – | – | – | – | – | – | – | ✗ | [238] | |
50 | – | – | – | – | – | – | – | ✗ | [78] | ||||
100 | – | – | ✓ | ✓ | – | – | – | ✓ | |||||
Cyanidin 3-O-β-glucopyranoside | APPswe/PS1dE9 | 5 | 8–10 months | O.g. | – | – | – | – | – | – | – | ✓ | [79]) |
Dihydromyricetin | Tg-SwiDi | 2 | 20–23 months | Orally | ✓ | – | – | – | – | ✓ | – | – | [26] |
Diosmin | 3xTg-AD | 1 | 6–10 months | Diet | ✓ | – | – | – | – | – | – | – | [27] |
10 | ✓ | – | – | – | – | – | – | – | |||||
EGCG | TgCRND8 (APP) | 50 | 2–6 months | Orally | ✓ | – | – | – | – | – | – | – | [28] |
SAMP8 | 5 | 60 days | I.g. | ✓ | – | – | – | – | – | ✓ | ✓ | [29] | |
15 | ✓ | – | – | – | – | – | ✓ | ✓ | |||||
APPswe/PS1dE9 | 2 | 12–13 months | O.g. | ✓ | – | – | ✓ | – | ✓ | – | ✓ | [30] | |
6 | ✓ | – | – | ✓ | – | ✓ | – | ✓ | |||||
2 | 9–10 months | Water | ✓ | – | – | – | – | – | ✓ | ✓ | [31] | ||
30 | 12–15 months | O.g. | ✓ | – | – | ✓ | * | ✓ | – | ✓ | [32] | ||
40 | 3–6 months | Water | ✓ | – | ✓ | – | – | – | – | – | [33] | ||
2 | 9–10 months | I.g. | ✓ | – | ✓ | ✓ | – | – | – | – | [34] | ||
50 | 8–14 months | Water | ✓ | – | – | – | – | – | – | – | [35]) | ||
IcrTacSam mice with intracerebroventricular injection of Aβ42 | 1.5 | 3 weeks prior to Aβ | Water | ✓ | – | – | – | – | – | ✓ | ✓ | [36] | |
3 | ✓ | – | – | – | – | – | ✓ | ✓ | |||||
IcrTacSam mice with daily i.p. injection of LPS for 1 week | 1.5 | 3 weeks prior to LPS | Water | – | – | – | – | – | – | – | ✓ | [37] | |
3 | ✓ | – | ✓ | ✓ | – | – | ✓ | ✓ | |||||
Ts65Dn | 30 | 5–6 months | Water | – | – | – | – | – | – | – | ✗ | [239] | |
(–)-Epicatechin | APPswe/PS1dE9 | 40 | 3–12 months | Diet | ✓ | – | ✓ | ✓ | – | – | – | ✗ | [38] |
50 | 8–12 months | Water | ✗ | – | – | – | ✓ | – | ✓ | ✗ | [131] | ||
TASTPM (APPswe/PS1 M146V) | 15mg/day | 21 Days from 7 months | Water | ✓ | – | – | – | – | – | – | – | [39] | |
Eriodictyol | LPS injection for 10 days at day 35 of Eriodictyol treatment | 25 | 45 days from 3 months | I.g. | – | – | – | ✓ | – | – | – | ✓ | [40] |
50 | ✓ | – | – | ✓ | – | – | – | ✓ | |||||
100 | ✓ | – | ✓ | ✓ | – | – | – | ✓ | |||||
Fisetin | huAPPswe/PS1dE9 | 25 | 3–9 months | Diet | ✓ | – | ✓ | – | ✓ | ✓ | – | ✓ | [41] |
SAMP8 | 25 | 3–10 months | Diet | – | – | ✓ | ✓ | ✓ | ✓ | – | – | [117] | |
Formononetin | APPswe/PS1dE9 | 15 | 7–8 months | I.g. | ✓ | – | – | ✓ | – | – | – | ✓ | [42] |
Hesperetin | C57BL.6N mice with LPS injection for 2 weeks by i.p. | 50 | 5 weeks (3 weeks prior to LPS) | O.g. | – | – | ✓ | ✓ | – | ✓ | ✓ | ✓ | [118] |
Hesperidin | APPswe/PS1dE9 | 50 | 3–7 months | Diet | ✗ | – | – | – | ✗ | – | – | ✗ | [43] |
100 | ✓ | – | – | – | ✓ | – | – | ✓ | |||||
20 | 9–12 months | I.g. | – | – | – | – | – | – | – | ✓ | [80] | ||
40 | – | – | – | – | ✓ | – | – | ✓ | |||||
80 | – | – | – | – | – | – | – | ✓ | |||||
APP/PS1–21 | 100 | 10 days from 5 months | O.g. | ✓ | – | ✓ | – | – | – | – | – | [44] | |
Icariin | APPswe/PS1dE9 | 30 | 10–14 months | O.g. | ✓ | – | – | – | – | – | – | – | [45] |
60 | ✓ | – | – | – | – | – | – | – | |||||
APP/PS1 | 60 | 4–12 months | O.g. | ✓ | – | – | ✓ | – | – | – | ✓ | [46] | |
APP/PS1 | 60 | 9–12 months | Orally | ✓ | – | – | – | – | – | ✓ | ✓ | [47] | |
5XFAD | 50μmol/kg/day | 8 days from 7–9 months | Orally | – | – | – | – | – | – | – | ✓ | [81] | |
APP/PS1–21 | 100 | 10 days from 5 months | O.g. | ✓ | – | ✓ | ✓ | – | – | – | – | [48] | |
3xTg-AD | 65 | 6–8 months | Diet | ✓ | – | – | – | – | ✓ | – | ✓ | [49] | |
Tg2576 (APPswe) | 60 | 9–12 months | Orally | ✓ | – | – | – | – | – | ✓ | – | [50] | |
APPV17I Tg | 30μmol/kg/day | 4–10 months | I.g. | ✓ | – | – | – | – | – | – | ✗ | [51] | |
100μmol/kg/day | ✓ | – | – | – | – | – | – | ✓ | |||||
Liquirigenin | Tg2576 (APPswe) | 3 | 10–13 months | I.g. | ✗ | – | – | – | – | – | – | ✗ | [52] |
10 | ✓ | – | – | – | – | – | – | ✓ | |||||
30 | ✓ | – | – | – | – | – | – | ✓ | |||||
Myricetin | Tg2576 (APPswe) | 0.5% diet | 5–14 months | Diet | ✓ | – | – | – | – | – | – | – | [53] |
Naringin | APPswe/PS1dE9 | 50 | 3–7 months | Diet | – | – | – | – | – | – | – | ✗ | [54] |
100 | ✓ | – | – | ✗ | – | – | – | ✓ | |||||
50 | – | – | – | – | – | – | – | ✗ | [82] | ||||
100 | – | – | – | – | – | – | – | ✓ | |||||
Pinocembrin | APPswe/PS1dE9 | 40 (5 days a week) | 4–7 months | O.g. | ✗ | – | ✓ | ✓ | – | – | – | ✓ | [83] |
Puerarin | APPswe/PS1dE9 | 30 | 8–9 months | Orally | ✗ | – | – | – | ✓ | – | – | ✓ | [84] |
Quercetin | 5XFAD | 500 | 2 –4 months | O.g. | ✓ | – | – | – | – | – | – | ✓ | [57] |
Intracerebroventricular injection of aggregated Aβ25–35 at 10 weeks | 50 | 2 weeks (from DPI 5) | Orally | – | – | – | ✓ | ✓ | – | – | – | [58] | |
APP/PS1 | 2mg/g diet | 1–13 months | Diet | ✓ | ✓ | – | – | – | – | – | ✓ | [59] | |
1–9 months | ✓ | ✓ | – | – | – | – | – | ✓ | |||||
6–13 months | ✗ | ✗ | – | – | – | – | – | ✗ | |||||
Rutin | Aβ25–35 injected into third ventricle of ICR mice | 100 | 2 weeks (following Aβ) | Orally | – | – | – | – | ✓ | – | – | ✓ | [85] |
APPswe/PS1dE9 | 100 | 8–9.5 months | Orally | ✓ | – | ✓ | ✓ | ✓ | – | – | ✓ | [55] | |
Scutellarin | APPswe/PS1dE9 | 50 | 9–12 months | Diet | ✓ | – | ✓ | ✓ | – | – | – | ✓ | [56] |
Flavonoid inhibition of APP processing and Aβ deposition
Several flavonoids have shown the capacity to ameliorate Aβ pathology, both in vitro and in vivo (Table 1). This effect may come as a result of a shift towards the non-amyloidogenic processing pathway, enhanced degradation of Aβ and inhibition of aggregation (Fig. 1A, B). The resultant effect is a net reduction in Aβ42. A well-established example of flavonoid-mediated inhibition of APP processing is seen with epigallocatechin gallate (EGCG), a much studied catechin that is abundant in tea. EGCG has been shown in vitro to dose dependently increase the proportion of mature ADAM10 (mADAM10) relative to pro-ADAM10, correlating with a shift towards non-amyloidogenic processing and a decrease in Aβ production [60, 61]. The increase in proteolytically active mADAM10 by EGCG was found to occur through at least two pathways: oestrogen receptor α (ERα)-mediated activation of the PI3K/Akt pathway, and activation of the pro-ADAM10 cleavage enzyme furin through a PI3K-independent mechanism [62]. The finding that EGCG shifts APP processing towards the non-amyloidogenic pathway has been confirmed in vivo in several different mouse models of AD [32, 35–37]. Oral administration of EGCG was found to promote α-secretase activity while down regulating β- and γ-secretase activity, and as such decreased the levels of both soluble and insoluble Aβ.
Another example is that of the flavone baicalein, which increases non-amyloidogenic cleavage of APP while inhibiting pro-amyloidogenic cleavage through a GABAA receptor-dependent pathway [63]. GABAA receptor activation has been shown previously to promote non-amyloidogenic processing of APP [64]. Furthermore, baicalein may regulate GABAA receptor activation [65], potentially through inhibition of GABA transaminase which degrades GABA [66].
Moreover, the modulation of APP processing by flavonoids is known to occur at very low concentrations that could potentially be found in the brain. For example, (–)-epicatechin ((–)-EC) inhibits βγ-secretase dependent APP processing with a low IC50 of 20.5 nM, which correlates with reduced Aβ pathology in TASTPM mice treated with (–)-EC [39]. Furthermore, both quercetin (100 nM) and quercetin-3-O--rutinoside (rutin) (50 nM, 100 nM) significantly increased degradation of BACE1 in SH-SY5Y cells over expressing APPs we [67]. These effects at low concentrations enhance the likelihood that flavonoids could modulate APP processing in vivo.
Another mechanism through which flavonoids could decrease the levels of Aβ in the brain is by enhancing degradation of Aβ. Neprilysin (NEP), a zinc-metalloprotease, is an Aβ-degrading enzyme which is decreased in affected areas of the AD brain [68]. EGCG (1–3μg/ml) increases NEP release from astrocytes in an Akt/PI3K-dependent manner, resulting in degradation of exogenous Aβ [69]. Consequently, intragastric administration of EGCG to SAMP8 mice, a senescence model of AD, increased NEP expression and reduced Aβ level same lio rating spatial learning [29]. Similarly, oral administration of a combination of green tea catechins, including EGCG, to a transgenic human APP over expression mouse caused an increase in NEP levels, as well as decreased Aβand improved spatial learning [70]. Hence, EGCG can promote the degradation of Aβ, as well as inhibiting production.
Alternatively, flavonoids have the potential to target Aβ through direct binding and inhibition of aggregation which has been demonstrated in vitro in aggregation assays [71–76] (Fig. 1B). A limitation of these studies is that they are typically undertaken at high stoichiometric Aβ-flavonoid ratios that would not easily be achieved in vivo. Nevertheless, such in vitro aggregation assays are useful in identifying flavonoid structures with anti-aggregative properties that could be used as molecular scaffolds for the design of therapeutics. Consideration of the physiological stoichiometry, impact of the cellular environment and posttranslational modifications of the target protein will enhance the overall translatability in vivo.
While in vivo experiments have shown that flavonoid treatments reduce the levels of insoluble, aggregated Aβ, it is not yet possible to determine whether this is due to direct flavonoid binding to the target protein or interactions with other proteins such as kinases, which modulate the tendency for amyloidogenic proteins to aggregate. Likewise, it is tempting to relate the changes in spatial learning and memory observed in through flavonoid intervention in APP-based models of AD[23–25,30–32,36,41–43,46,49–53,54–59,77–85]to reductions in Aβ (Table 1). However, the behavioural recovery observed could be due to recruitment of other mechanisms, either downstream of Aβ signalling or involved in physiological cognition, particularly as not all studies with reductions in Aβ-pathology correlated with behavioural improvements [38, 51] and vice versa [77, 83, 84]. Indeed, there is evidence to suggest that flavonoids modulate other disease-associated mechanisms, therefore having the potential to act as multi-modal AD therapeutics.
Flavonoid inhibition of tau phosphorylation and aggregation
Tau is a microtubule-associated protein that, physiologically, binds to microtubules predominantly in axons [86, 87]. The primary function of tau is thought to be the stabilisation and organisation of microtubules, aiding in the transport of cargo to and from synapses. However, in AD, tau is hyperphosphorylated [88, 89], and dissociates from the microtubule (Fig. 1E). Hyperphosphorylation of tau promotes its mislocalization to the somatodendritic region [86] (Fig. 1G) where it has been shown to impair nuclear import [90], and synaptic function [91] (Fig. 1H). Tau accumulation in the somatodendritic region has also been associated with local translation of tau mRNA, which can be induced by Aβ application via a FYN/ERK/S6 pathway [92]. Hyperphosphorylated tau also aggregates into oligomers, eventually forming insoluble straight/paired helical filaments and neurofibrillary tangles (NFT) which are one of the characteristic hallmarks of AD (Fig. 1F). Several kinases are thought to contribute to the hyperphosphorylated state of tau in AD, especially the proline directed kinases; GSK-3β [93, 94], CDK5 [95, 96] and members of the MAPK family [97]. The reported activity of flavonoids within MAPK and other protein kinase signalling pathways raises the potential for flavonoids to modulate tau phosphorylation, thereby impacting on the development of tau pathology. This is highlighted in Fig. 3, which demonstrates the extent to which flavonoids have the potential to influence tau phosphorylation [98–109].
Fig. 3
Flavonoids can modulate kinases involved in the hyperphosphorylation of tau. (A) Tau contains an amino-terminal domain, a proline rich region and microtubule binding repeats (R1–R4). The diagram highlights the identified phosphorylation sites on tau which have been shown to be phosphorylated by kinases that can be modulated by flavonoids: GSK3β, ERK2, CDK5, JNK, p38 and Akt [98–109]. (B) A diagram explaining how flavonoids may attenuate tau phosphorylation by modulation of kinases.
![Flavonoids can modulate kinases involved in the hyperphosphorylation of tau. (A) Tau contains an amino-terminal domain, a proline rich region and microtubule binding repeats (R1–R4). The diagram highlights the identified phosphorylation sites on tau which have been shown to be phosphorylated by kinases that can be modulated by flavonoids: GSK3β, ERK2, CDK5, JNK, p38 and Akt [98–109]. (B) A diagram explaining how flavonoids may attenuate tau phosphorylation by modulation of kinases.](https://content.iospress.com:443/media/bpl/2020/6-2/bpl-6-2-bpl200098/bpl-6-bpl200098-g003.jpg)
A number of studies have demonstrated a role for JNK in tau phosphorylation [110, 111], and inhibiting JNK in primary cortical neurons, a transgenic Aβ mouse model and human fibroblasts derived from AD patients all showed a decrease in phosphorylation of tau at Ser202, Ser205 and Ser422 [108]. Several flavonoids have been shown to inhibit JNK activity in vitro [112–115], although not typically at concentrations found in vivo. Despite this, there is evidence from animal models of AD that oral or intragastric flavonoid administration can inhibit the activity of JNK [30, 40, 116–118].
The MAPK member p38 kinase has also been proposed to contribute to hyperphosphorylation of tau both directly [119] and indirectly, for example through activation of the CDK5 activator p35 [120] (Fig. 3). As such, increased levels of p38 kinase and CDK5 were found to correlate with tau hyperphosphorylation in a htau transgenic mouse model [121]. Furthermore, both p38 kinase and JNK were found associated with NFTs in AD brains [122] and in a patient with P301L-associated FTDP-17 [123]. Moreover, as will be discussed later, several lines of evidence suggest that neuroinflammation induces p38 kinase-dependent phosphorylation of tau [120, 124–126]. However, despite evidence supporting p38 kinase-mediated phosphorylation of tau as well as flavonoid interactions with p38 kinase [127] and flavonoid modulation of p38 kinase activity in a variety of systems [40, 128, 129], there have been few attempts to directly link the two mechanisms. For example, administration of proanthocyanidins by oral gavage reduced lead-induced tau phosphorylation which correlated with levels of activated p38 kinase and JNK, but no direct association was made [130] (Table 1).
An alternative mechanism for the inhibition of tau phosphorylation by flavonoids is through activation of the PI3K/Akt pathway which inhibits the key tau kinase GSK-3β (Fig. 3) by phosphorylation at Ser9. Delivery of naringin [54], hesperidin [43, 80], EGCG [30], (–)-EC [131], and baicalin [25] through oral routes to mouse models of AD have all been found to inhibit GSK-3β phosphorylation at Ser9. Additionally, several in vitro and in silico models have pointed towards direct inhibition of GSK-3β by flavonoids. For example, crystal structure studies of GSK-3β in complex with the flavonol morin revealed direct inhibition of GSK-3β through interactions with the ATP binding pocket [132], which correlates with studies demonstrating reduction in tau phosphorylation in 3×Tg mice carrying mutations in APPswe, PS1 M146V and taup301L[133]. Similarly in silico docking studies with quercetin and other citrus flavonoids suggested direct interaction with the GSK-3β active site and direct inhibition was confirmed in vitro [124, 131]. Furthermore, unlike other serine/threonine protein kinases, GSK-3β phosphorylation prefers pre-phosphorylation 4 amino acids C-terminally to the target phosphorylation site [135–138]. This adds an additional layer of regulation, as inhibition of other tau-kinases can consequently inhibit GSK-3β phosphorylation of tau.
The few studies that have looked to directly link flavonoid treatment with tau phosphorylation have shown promising results. Oral delivery of grape seed polyphenolic extract (GSPE), which contains high concentrations of proanthocyanidins, particularly catechin and (–)-EC oligomers, has been shown to inhibit tau phosphorylation at several key sites, such as: Ser202/Ser205 (AT8), Ser396/Ser404 (PHF-1), Thr212/Ser214 (AT100) [139] and Thr181 [140]. Alongside this, significant reductions in the presence of Sarcosyl-insoluble tau follow GSPE treatment, suggestive of a reduction in aggregation [139, 140]. It has been suggested that the mechanism through which GSPE elicits these effects is primarily through inhibition of ERK1/2, as GSPE treatment did not correlate with inhibition of GSK3β. A role for ERK1/2 in phosphorylation of tau in AD is supported by in vitro studies and immunohistological analysis of post-mortem brains [97, 141, 142].
Therefore, several flavonoids have the capacity to reduce tau phosphorylation, either through inhibition of GSK-3β via activation of the PI3K/Akt pathway or modulation of MAPKs that are capable of phosphorylating tau directly (Fig. 3). However, there is a clear lack of studies exploring the direct link between flavonoid-mediated modulation of tau-phosphorylating kinases and the phosphorylation state of tau itself. As such, more studies are required to determine whether flavonoid-mediated inhibition of tau phosphorylation is achievable at the nanomolar concentrations that might be detected in brain. It will be essential to investigate this in order to determine the full therapeutic potential of flavonoidsin AD prevention.
Similar to Aβ, flavonoids have also been suggested to inhibit tau aggregation (Fig. 1F). Flavanols are the predominant group associated with inhibition of tau aggregation, although the flavone baicalein has been shown to inhibit tau oligomerization, albeit with a relatively high IC50 (∼35μM) [143]. GSPE inhibits tau aggregation of the 306VQIVYK311 hexapeptide [140, 144], which is thought to be essential in aggregation of full length tau [145] and destabilises tauopathy patient-derived fibrils [146]. Interestingly, the oxidation of (–)-EC by H2O2 has been shown to facilitate inhibition of tau aggregation [147]. Studies giving more consideration to stoichiometric ratios have demonstrated that (–)-Epicatechin-3-gallate (ECG) inhibits tau aggregation with an IC50-of 1.8μM [148] and EGCG inhibits the aggregation of a tau peptide at substoichiometric levels (0.1X) [149]. While more studies are needed to determine the impact of this inhibition of tau aggregation in a cellular context, these studies suggest that flavonoid-mediated inhibition of tau aggregation is a possible approach for reducing AD pathology.
Flavonoids, therefore, have the potential to interfere in the pathological phosphorylation of tau, as well as in the formation of tau oligomers and NFTs. Considering that tau phosphorylation and aggregation occurs consequently to Aβ pathology, and therefore, is a more tractable target for impairing AD progression, a reduction in tau pathology could suggest intervention is possible at MCI and later stages of disease.
Flavonoid modulation of neuroinflammatory response in AD
The role of neuroinflammation in the development and progression of AD has long been debated. However, astrogliosis, microgliosis and chronic inflammation are now well accepted pathological hallmarks of AD, alongside plaques and tangles [150]. Genome wide association studies (GWAS) [151] and transcriptomics [152, 153] have highlighted the involvement of the innate immune system in AD. In particular microglia have been linked to Aβ and tau spreading and propagation [154], synaptic phagocytosis [155–157], and the release of pro-inflammatory cytokines that promote AD pathology [150, 158].
An increasing number of studies have implicated microglia as the missing link between Aβ accumulation and tau pathology (Fig. 1C). In transgenic mice expressing the P301S mutation associated with frontotemporal dementia (FTD), microglial activation and synapse loss occurred prior to development of tau tangles [159]. Furthermore, suppression of the immune response with FK506 reduced tau pathology and increased lifespan. Histological analysis of post-mortem brain correlated increased microglial activation with AD pathology, suggesting that microglial activation occurred following Aβ plaque deposition but prior to NFT formation [160]. Analysis of the transcriptional profile of APPswe/PS1dE9 and Tau22 transgenic mouse models, which exhibit amyloid and tau pathology respectively found that while Aβ pathology activated the expression of several AD risk genes associated with microglia, this did not occur in the tau transgenic mouse [161]. Thus, providing further evidence that microglial activation lies upstream of tau phosphorylation and downstream of Aβ. Furthermore, silencing genes associated with the microglial inflammasome (Nlrp3–/–and Asc–/–) in the Tau22 transgenic mouse model of tauopathy led to reduced tau phosphorylation and aggregation [162]. Significantly, this pathway could be stimulated by Aβ-fibrils and was essential to Aβ- associated induction of tau phosphorylation in Tau22 mice following hippocampal injection of APP/PS1 brain homogenate [162]. This work presents a possible timeline of AD pathology, with Aβ accumulation initiating activation of NLRP3 inflammasomes (Fig. 1C) in microglia, which consequently cause release of IL-1β (Fig. 1D) and thus triggers tau phosphorylation (Fig. 1E). As IL-1β release has been shown to cause tau phosphorylation in a p38-MAPK-dependent manner [125, 126], this presents an additional target for flavonoid intervention.
A reduction in gliosis [33, 34, 37, 38, 40, 41, 44, 48, 55, 56, 59, 78, 83, 117, 118, 163] as well as neuroinflammatory markers [25, 30, 32, 34, 37, 38, 40, 42, 46, 48, 55, 56, 58, 78, 83, 117, 118, 163] has been shown in many animal models of AD orally administered with flavonoids (Table 1). Additionally, several flavonoids have been identified that inhibit microglial activation and IL-1β release. The isoflavone genistein reduced mRNA levels of IL-1β and TNF-α through a G-protein coupled oestrogen receptor (GPER)-dependent mechanism in primary microglia and BV2 cells [164]. Pre-treatment with the flavanone pinocembrin inhibited LPS-induced activation of BV2 cells and consequent release of pro-inflammatory mediators including IL-1β [165]. In vivo, intra-gastric administration of procyanidins reduced morphine-induced activation of NLRP3 and IL-1β, likely through inhibition of p38 kinase [166]. Furthermore, a number of in vivo studies of flavonoid intake in AD mouse models have shown a reduction in IL-1β following oral or gastric administration of EGCG [32, 34], formononetin [42] and eriodictyol [40]. Alongside this, many studies have reported decreases in levels of activated microglia following flavonoid treatment [167]
In addition to IL-1β, other pro-inflammatory cytokines have been suggested to play a role in AD. Tumour necrosis factor-α (TNF-α), which can be reduced by flavonoids [167], has also been implicated in AD [168, 169]. TNF-α, and to a lesser extent IL-1β was found to upregulate γ-secretase cleavage of APP through a JNK-dependent pathway, albeit in stably transfected HEK293 cells [170]. Furthermore, IL-6, which has been shown to be down regulated following administration of baicalin [78], formononetin [42] and eriodictyol [40], induces AD-like phosphorylation of tau in primary rat hippocampal neurons [120] (Fig. 1D). This phosphorylation was found to be due to activation of p38 kinase which caused upregulation of the CDK5 activator p35. Therefore, it is possible that flavonoid-induced down regulation of IL-6 in AD mouse models could reduce tau phosphorylation.
Given the accumulating evidence linking microglial activation, pro-inflammatory cytokine release and tau phosphorylation, it would be of interest to determine whether flavonoid-mediated attenuation of the microglial responses correlates with decreased tau pathology.
Flavonoid modulation of antioxidant responses in AD
Flavonoids are generally known for their antioxidant capacity [171], which may have benefit in AD as oxidative stress has been implicated in neurodegeneration [172]. Evidence from post-mortem studies on AD-brain shows that, relative to control brain, there is a net reduction in the ability to decrease H2O2 levels and stimulate anti-oxidative stress pathways [173, 174]. H2O2 can act as a messenger molecule activating signalling pathways, such as MAPK [175, 176], or react with metallic cations, such as Fe2+, to produce HO· [177] which can damage cellular components such as lipids and proteins. Imbalances in brain-iron levels have been reported in AD [178–181] and likely contribute to cognitive decline [182], supporting the suggestion that oxidative stress promotes AD pathology. Indeed, studies in transgenic APP/PS1 mice showed that increasing oxidative stress in the brain through delivery of a pro-oxidant iron rich diet increased the deposition of Aβ [183].
While flavonoids have the capacity to act directly as reactive oxygen scavengers by donating hydrogen, it is unlikely that this is the mechanism by which theyelicit their antioxidant potential due to the low circulating concentration of flavonoids in the brain relative to better characterised ROS scavengers such as ascorbic acid [184, 185]. Instead, it is most likely that the mechanism through which flavonoids inhibit oxidative stress is through modulation of intracellular pathways such as the MAPK pathway. The MAPK pathway responds to changes in the cellular environment, such as oxidative stress, and transduces these stimuli into cellular responses through phosphorylation or activation of transcription factors. Activation of anti-apoptotic ERK1/2 and PI3K/AKT pathways, and downregulation of pro-apoptotic JNK pathways by flavonoids is neuroprotective against multiple stressors including oxidative stress at both high [112, 186] and physiological concentrations [114, 128].
Another mechanism through which flavonoids could act as antioxidants is via PI3K dependent activation of the transcription factor Nuclear factor erythroid 2-related factor 2 (Nrf2). Activation of anti-oxidant response element (ARE) by Nrf2 enhances the expression of antioxidant-related genes, such as those involved in synthesis of several anti-oxidative enzymes including glutathione peroxidase (GPx), catalase (CAT), heme-oxygenase 1 (HO-1) and superoxide dismutase (SOD) [187]. Flavonoids have consistently been shown to activate Nrf2 in non-neuronal cell lines [188–190]. Furthermore, physiological levels of (–)-EC have been shown to upregulate ARE activity in astrocytes in a PI3-K dependent manner [191], which has been shown to protect neurons against oxidative stress [192]. Quercetin [193] and EGCG [194] have also been found to promote Nrf2 signalling in primary neurons, however, the effects at physiologically relevant concentrations were not investigated and there is uncertainty as to the extent to which Nrf2 is expressed in mature neurons.
Oral ingestion, or intragastric administration, of fisetin [41], hesperidin [43, 80], quercetin [58], rutin [55, 85], apigenin [24], puerarin [84] or (–)-EC [131] has been shown to reduce markers of oxidative stress in the brains of mouse models of AD (Table 1). This was demonstrated through increased activity of anti-oxidative enzymes such as GPx, CAT and SOD and a reduction in measurable H2O2, ROS and malondialdehyde (MDA). Collectively this supports a PI3K/Akt/Nrf2/ARE mechanism for conferring neuroprotection against oxidative stress by flavonoids which may be beneficial in ameliorating AD pathology.
Flavonoids promote BDNF induced neurogenesis in AD
Brain-derived neurotrophic factor (BDNF) is known to play an important role in neural development, differentiation and synaptic plasticity through binding to its receptor TrkB. BDNF levels are also known to be decreased in the AD brain relative to healthy controls [195–197]. Furthermore, decreased levels of BDNF have been linked to delta-secretase mediated cleavage of both Aβ and tau and subsequent neurodegeneration [198, 199]. BDNF also plays an important role in neurogenesis. The existence of adult hippocampal neurogenesis (AHN) in humans has been highly contested through the years, yet new advances in tissue processing techniques have now confirmed AHN [200, 201]. Moreover, it has been shown that, while neurogenesis decreases with aging, this decline is greatly exaggerated in AD [200]. Targeting BDNF/TrkB signalling to promote synaptic plasticity and neurogenesis may in turn slow the deterioration of the learning and memory abilities of AD patients.
There is evidence that flavonoids can promote BDNF/TrkB signalling and related downstream effects. Physiological concentrations of (–)-EC (100 nM) activate the cAMP response element binding protein (CREB) and consequently upregulate levels of BDNF in primary cortical neurons [202]. Both quercetin and (–)-EC have been shown to upregulate the expression levels of BDNF following oral administration to several rodent models of AD [131, 203–205]. Furthermore, oral delivery of 7,8-dihydroxyflavone (7,8-DHF), a TrkB agonist, has been shown to enhance long term potentiation (LTP), reduce synaptic loss, and improve performance in the Morris water maze (MWM) in vivo [23, 77]. Alongside the increased levels of BDNF seen with quercetin treatment, increases in neural progenitor cells (NPCs) and neural stem cells (NSC) associated with neurogenesishave also been shown [205].
Given this evidence, flavonoid-induced upregulation of the BDNF/TrkB pathway to increase neurogenesis and reduce synaptic loss is an additional mechanism through which flavonoids may be able to reduce AD pathology (Fig. 1I).
EMERGING CONSIDERATIONS FOR FLAVONOID INTERVENTIONS IN AD
Given the low success rate for translating therapeutics from in vivo animal studies to effective human interventions for AD, it is becoming increasingly important to ensure that the ‘translatability gap’ is as small as possible. In this section we consider emerging evidence as to the extent of metabolism and bioavailability of flavonoids, and how this could influence the interpretation of existing data and experimental design moving forward.
Flavonoid metabolome and brain bioavailability
While the ‘key’ metabolites of several notable flavonoids have been known for years, there is only limited knowledge of the full metabolite profiles and, more importantly for AD intervention, which metabolites reach the brain.
In this respect, important advances in the field have been the use of radio labelling to determine complete flavonoid metabolomes. Such examples include identification of the anthocyanidincyanidin-3-glucoside (C3G) metabolome in humans [206] as well as the (–)-EC metabolome in both humans and rodents [207, 208]. As a result, 24 C3G and >20 (–)-EC metabolites have now been identified in humans. In comparing these metabolomes, there are similarities in the metabolites produced, such as the presence of structurally related molecules which have been glucuronidated, methylated or sulphated, as well as structurally unrelated metabolites such as hippuric acid. Both of these human studies found that the flavonoid metabolites were much more abundant than the parent flavonoid molecule, to the extent that 14C-(–)-EC itself was undetectable in the plasma or urine. Therefore, it is likely that the bioactivity in vivo resides in one or more flavonoid-derived metabolites rather than the parent flavonoid. This is important as many in vitro experiments use only parent flavonoids to determine mechanisms of action and itis unlikely that these unmetabolized flavonoids will be available in the brain at active concentrations. In relation to (–)-EC, structurally related epicatechin metabolites (SREM) are likely to be the predominant bioactive constituents in the human brain, given the absence of the parent (–)-EC molecule in human plasma [207]. This corresponds with pharmacokinetic studies in rats which showed that chronic oral administration of (–)-EC and catechin (C) resulted in a combined concentration of ∼484 nM (–)-EC/C-glucuronides and methyl-(–)-EC/C-glucuronides, while unmetabolized (–)-EC could only be found in trace amounts [209]. Despite this many in vitro studies focus on concentrations >10μM. Flavonoids often exhibit biphasic responses, activating protein kinase signalling pathways at low concentrations (nM) and inhibiting at high concentrations (μM), or vice versa [39, 114, 193]. Therefore, it is possible that where flavonoids are shown to inhibit pro-AD pathways in vitro at high concentrations, in vivo at nanomolar concentrations they may elicit different effects.
Considering this, metabolic profiles for other flavonoids considered as potential therapeutic interventions for dementia should be established to aid mechanistic investigations. In order to determine bioavailability in the brain analysis of cerebral spinal fluid (CSF) could potentially be undertaken to confirm the appearance of flavonoid-derived metabolites.
Species differences in flavonoid metabolism limit translatability of in vitro and in vivo studies
The metabolism of flavonoids is an important consideration when designing and interpreting animal studies. While there are plenty of studies on flavonoid metabolism in rodents, the information about human metabolism is lacking. As more information becomes available, it is becoming clear that there are key differences in flavanol metabolism between species, which may further limit the translatability of in vivo studies.
An example of this is the absence of (–)-EC-3’-O-glucuronide and (–)-EC-3’-sulfate, the main metabolites of (–)-EC found in plasma and urine of humans, in rats [185, 208]. It is, therefore, not possible to determine the effect of these metabolites on the rodent brain through delivery of (–)-EC alone. Such investigations would require direct i.p. injection of these metabolites or administration by osmotic mini-pump.
Furthermore, as mentioned previously, (–)-EC was not identified in plasma or urine of humans [208], although it was in rats [207]. Likewise, C3G metabolites were found to be 42 fold more abundant than the parent C3G molecule in humans [206], whereas in mice the difference was only 6 fold [210]. Therefore, highlighting doubts about the contribution of unmetabolized flavonoids in the human brain relative to the rodent models used in pre-clinical trials.
Studies of key quercetin metabolites in gerbils, mice and rats showed that there are species dependent differences in the metabolism of quercetin [211]. Given what we now know about (–)-EC metabolism, it is likely that this would correlate with differences in quercetin metabolism between humans and rodents as well. Without knowing what flavonoid metabolites are found in humans, and particularly in the brain, it is hard to pre-determine if flavonoids would elicit therapeutic benefit in humans, despite positive findings in animal models (Table 1).
Harnessing metabolites for flavonoid intervention studies for AD
Utilising the information now available about the pharmacokinetics and bioavailability of (–)-EC, Ottaviani et al. have identified a collection of SREMs that have the potential to be utilised as biomarkers for (–)-EC intake [212]. The levels of (–)-EC-3’-glucuronide, (–)-EC-3’-sulfate and 3’-O-methyl-(–)-EC-5-sulfate, collectively termed SREMB, in the urine can be used to estimate the amount of (–)-EC ingested 24 hours previously. The standard practice for epidemiological studies interested in implications of dietary compounds on health and disease is through self-reporting of subjects to estimate consumption, which is inherently unreliable. With biomarkers to assess the actual amounts of (–)-EC in the diet, future epidemiological studies will be able to investigate the effect of (–)-EC on AD with greater accuracy and certainty.
The impact of the microbiome on flavonoid metabolism and AD
It is now known that the gut-microbiome is responsible for the generation of several flavonoid metabolites [185, 206, 208, 213–216]. The microbiome can partake in the deglycosylation, ring fission and reduction of flavonoids [215], with deglycosylation being of particular importance, as this is thought to be essential for flavonoid absorption [217], with the exception of C3G [218]. Despite making up a large proportion of circulating flavonoid-derived metabolites, few studies to date have explored the mechanistic function of microbiome-derived metabolites in relation to AD. An example of such biologically relevant metabolites are phenyl-γ-valerolactones and phenyl-γ-valeric acids, formed from microbiome metabolism of flavanols. In vitro assays have suggested that these metabolites can permeate the blood brain barrier (BBB) [219] and reduce Aβ-toxicity [220].There is further suggestion that 3-HBA and 3-HPP, derived from GSPE metabolism by the rat microbiome, can impair the aggregation of Aβ peptides, nonetheless at high molar ratios [213]. Other studies in SHSY-5Y cells show some ability of microbiome-derived metabolites of flavanols to act in a neuroprotective manner against H2O2 treatment and SIN-1-induced stress [221, 222]. There is recent evidence that flavonoid intake can alter the composition of the human gut microbiome [223]. However, it is yet to be determined what effect, if any, this may have on health and disease. An increasing number of studies have suggested a role for the gut microbiome in AD, most likely through modulation of microglial activation. One study in cognitively impaired elderly found a significant increase in abundance of pro-inflammatory bacteria as well as decreased abundance of anti-inflammatory bacteria in the gut of Aβ positive relative to Aβ-negative participants [224]. Similarly, it was also found that AD patients have a distinct gut-microbiome composition relative to age and sex-matched controls [225]. Likewise, 5XFAD mice exhibited a different microbiome composition relative to non-transgenic controls and this dysbiosis was suggested to promote microglial activation [226]. By treating these mice with GV-971, which promotes normal gut-microbiome composition, Aβ, tau and behavioural pathology was significantly reduced. Interestingly, the treatment of APPPS1-21 mice with antibiotics led to a reduction in Aβ and microglial pathology in male, but not female, mice, suggesting a sex-specific role for the microbiome in AD [227]. Therefore, modulation of the microbiome composition by flavonoids should be considered carefully as this has potential to promote or hinder AD progression.
Furthermore, there are important inter-individual differences in the composition of the gut microbiome as a result of various factors including age, sex, drug use and dietary intake [228]. This is significant as the composition of the human gut microbiome has so far been shown to alter the metabolism of flavonols [229, 230] and isoflavones [215]. Therefore, it may become beneficial to assess the microbiome of an individual before recommending flavonoid interventions in order to personalise the amount and type of flavonoids for maximum efficacy. Furthermore, combined treatment with pro-biotics might enhance the effects of flavonoid intake by ensuring the most efficient microbiota are present in the gut. Moreover, AD risk factors such as diabetes and obesity also influence polyphenol uptake and metabolism [231, 232]. Although this might not result from direct changes to the microbiome, they could impair an individual’s responsiveness to flavonoid intervention.
Moving forward therefore, the potential contribution of the microbiome should be taken into consideration when looking to assess the bioavailability and efficacy of flavonoids or flavonoid-derived drugs particularly when the method of flavonoid delivery bypasses the gut and other key sites of metabolism.
Alterations to Promote the Stability, Bioavailability, Specificity and Activity of Flavonoids
Like many small molecule and antibody therapies, several flavonoids are likely to exhibit low brain bioavailability, a potential limitation of their use as therapeutic interventions in humans[233]. One method to overcome this is to encapsulate the flavonoids in nanoparticles. This increases their stability and brain bioavailability as well as promoting sustained release [234]. Furthermore, nanoparticles can include targeting peptides in their coating, allowing for more specific activity. As a result, nano-encapsulation could reduce the quantity of flavonoid that would need to be administered.
Nano-encapsulation of EGCG improved bio availability in rats as well as its α-secretase promoting activity in SHSY-5Y cells [235]. Furthermore, nanoparticles loaded with EGCG and ascorbic acid were found to decrease Aβ deposition and increase synaptic expression relative to EGCG alone in APP/PS1 mice, which correlated with improved spatial learning [33]. However, it is unclear whether this is a result of the nanoparticles or the added ascorbic acid, which is a powerful antioxidant. It should also be noted that this study found that the nanoparticles may disrupt the BBB, which needs to be investigated further in vivo [33]. Nano-encapsulation of quercetin also showed significant improvements to cognition and memory in SAMP8 mice as well as lower levels of markers of inflammation relative to free quercetin [236]. These results were in-line with increased quercetin levels in the brain (∼200 ng/g free quercetin: 371 ng/g nano-encapsulated quercetin). Therefore, nano-encapsulating flavonoids may improve their efficacy in vivo, thus making them more appealing as therapeutic interventions for AD.
Their capacity to act at multiple targets associated with AD pathology makes flavonoids attractive molecular scaffolds for development of more potent inhibitors of AD pathology. Alteration of the flavonoid structure has been found to enhance inhibition of BACE-1, Aβ aggregation and acetylcholinesterase (AChE) activity, as reviewed by Jalili-Baleh (2018) [237]. However, it is possible that these changes will impact the ability of the flavonoid-based drug to reach the brain.
While the development of more potent and specific flavonoid-derived therapeutics has the potential to elicit stronger effects, the switch from nutraceutical to pharmaceutical brings its own hurdles. The increased expense and potency could mean that these compounds are no longer suitable for early and sustained delivery throughout midlife. In contrast, supplementation with naturally available flavonoids is likely to be more widely accessible, with potentially fewer side effects. As such, even if direct modulation of AD pathways is weaker, capacity for long term delivery could contribute to an overall benefit.
Incorporating the Metabolome to Promote Translatability in Identifying a Therapeutic Intervention for AD
The identification of the (–)-EC metabolome yielded a library of potential bioactive molecules, retrospective biomarkers of (–)-EC intake, and a greater understanding of the limitations of the animal models used. In the future, it would be of great value to elucidate the full human metabolomes of more flavonoids in order to limit the risk of failure at clinical trials. Utilising techniques such as the radiolabelling used by Czank et al. (2013) and Ottaviani et al. (2016) to identify the full human metabolome of these clinically relevant flavonoids may help to bridge the translatability gap between pre-clinical investigations and human interventions.
An important point to consider is that despite considerable uncertainty that parent flavonoids are present at sufficient concentrations in the brain, the metabolites themselves likely have poor bioavailability following oral delivery. To overcome this, one route would be to nano-encapsulate the metabolite of interest, thus improving its bioavailability. Alternatively, it may be more efficient to use the parent-flavonoid as a pro-drug, which with appropriate dosing will facilitate availability of metabolites in the brain. In this scenario, it will be necessary to investigate the mechanistic effects of combinations of metabolites that derive from the same parent flavonoid, to ensure a consistent mechanism of action.
While the incorporation of metabolism and brain bioavailability creates additional hurdles in experimental design, it also increases the likelihood of accurately identifying bioactive flavonoids or flavonoid-derived metabolites that can be used as therapeutic interventions for AD in humans.
CONCLUSIONS
As this review has highlighted, flavonoids have the potential to act as multimodal therapeutics to prevent the onset and/or progression of AD. Much of the research effort to date has focused on the reduction of Aβ by flavonoids. The advantage of flavonoids over conventional Aβ-targeting drugs such as antibodies and small molecule inhibitors is that it is possible for them to be administered as dietary supplements. Due to their relative affordability and low toxicity, supplementation with flavonoids could enable early protection at a young age and throughout life, without the need for pre-clinical diagnosis. However, determining if early and consistent flavonoid intake is capable of preventing AD onset would require an enormous amount of resource and most importantly, time. Until it is possible to accurately identify AD pre-symptomatically, before Aβ accumulation has initiated downstream toxic cascades, it may be necessary to shift the focus to flavonoid induced changes to later-onset pathologies such as neuroinflammation or tau phosphorylation. To date, tau pathology is clearly underrepresented in flavonoid-intervention studies carried out in mouse models of AD despite the strong basis for flavonoid-modulation of tau phosphorylating kinases.
Moving forward, it will be important to incorporate the bioavailability and metabolism into experimental planning throughout all stages of pre-clinical research. To reduce the current translatability gap, it will be essential to:
1. Focus on physiologically relevant concentrations when determining the mechanistic functions of flavonoids in vitro, to ensure that said mechanisms are possible in vivo.
2. Identify the complete human metabolomes of flavonoids of interest to determine functional metabolites.
3. Compare the mechanisms of action of parent flavonoids with their human metabolites, both alone and in combination, to ensure that the same mechanisms will be engaged following in vivo administration.
Taking this into consideration should result in more efficient and effective identification of flavonoid-based molecules that could succeed as therapeutic interventions for AD.
CONFLICTS OF INTEREST
The authors declare no conflicts of interest.
ACKNOWLEDGMENTS
KH is supported by the MRC GW4 BioMed DTP, RW acknowledges support from Alzheimer’s Society (481 AS-PG-18b-009), Alzheimer’s Research UK (EG2018A-008), and an open grant from Mars Edge. All figures were created with BioRender.
REFERENCES
[1] | Prince MJ , Wimo A , Guerchet MM , Ali GC , Wu Y-T , Prina M . World Alzheimer Report 2015 – The Global Impact of Dementia: An analysis of prevalence, incidence, cost and trends. Alzheimer’s Disease International; 2015. |
[2] | Hardy J , Selkoe DJ . The amyloid hypothesis of Alzheimer’s disease: progress and problems on the road to therapeutics. Science. (2002) ;297: (5580):353–6. doi:10.1126/science.1072994 |
[3] | Clark RF , Hutton M , Fuldner M , Froelich S , Karran E , Talbot C , et al. The structure of the presenilin 1 (S182) gene and identification of six novel mutations in early onset AD families. Nat Genet. (1995) ;11: (2):219–22. doi:10.1038/ng1095-219 |
[4] | Sherrington R , Rogaev EI , Liang Y , Rogaeva EA , Levesque G , Ikeda M , et al. Cloning of a gene bearing missense mutations in early-onset familial Alzheimer’s disease. Nature. (1995) ;375: (6534):754–60. doi:10.1038/375754a0 |
[5] | Levy-Lahad E , Wasco W , Poorkaj P , Romano DM , Oshima J , Pettingell WH , et al. Candidate gene for the chromosome 1 familial Alzheimer’s disease locus. Science (80-). (1995) ;269: (5226):973–7. doi:10.1126/science.7638622 |
[6] | Rogaev EI , Sherrington R , Rogaeva EA , Levesque G , Ikeda M , Liang Y , et al. Familial Alzheimer’s disease in kindreds with missense mutations in a gene on chromosome 1 related to the Alzheimer’s disease type 3 gene. Nature. (1995) ;376: (6543):775–8. doi:10.1038/376775a0 |
[7] | Scheuner D , Eckman C , Jensen M , Song X , Citron M , Suzuki N , et al. Secreted amyloid β-protein similar to that in the senile plaques of Alzheimer’s disease is increased in vivo by the presenilin 1 and 2 and APP mutations linked to familial Alzheimer’s disease. Nat Med. (1996) ;2: (8):864–70. doi:10.1038/nm0896-864 |
[8] | Citron M , Westaway D , Xia W , Carlson G , Diehl T , Levesque G , et al. Mutant presenilins of Alzheimer’s disease increase production of 42-residue amyloid β-protein in both transfected cells and transgenic mice. Nat Med. (1997) ;3: (1):67–72. doi:10.1038/nm0197-67 |
[9] | Borchelt DR , Thinakaran G , Eckman CB , Lee MK , Davenport F , Ratovitsky T , et al. Familial Alzheimer’s disease-linked presenilin I variants elevate aβ1-42/1-40 ratio in vitro and in vivo . Neuron. (1996) ;17: (5):1005–13. doi:10.1016/s0896-6273(00)80230-5 |
[10] | Wu L , Rosa-Neto P , Hsiung G-YR , Sadovnick AD , Masellis M , Black SE , et al. Early-Onset Familial Alzheimer’s Disease (EOFAD). Can J Neurol Sci. (2012) ;39: :436–45. doi:10.1017/s0317167100013949 |
[11] | Gertsik N , Chiu D , Li YM . Complex regulation of γ- secretase: From obligatory to modulatory subunits. Vol. 7, Frontiers in Aging Neuroscience. Frontiers Media S.A.; 2015. p. 342. doi:10.3389/fnagi.2014.00342 |
[12] | Moussa-Pacha NM , Abdin SM , Omar HA , Alniss H , Al-Tel TH . BACE1 inhibitors: Current status and future directions in treating Alzheimer’s disease. Med Res Rev. (2020) ;40: (1):339–84. doi:10.1002/med.21622 |
[13] | Ou-Yang MH , Kurz JE , Nomura T , Popovic J , Rajapaksha TW , Dong H , et al. Axonal organization defects in the hippocampus of adult conditional BACE1 knockout mice. Sci Transl Med. (2018) ;10: (459). doi:10.1126/scitranslmed.aao5620 |
[14] | Honig LS , Vellas B , Woodward M , Boada M , Bullock R , Borrie M , et al. Trial of solanezumab for mild dementia due to Alzheimer’s disease. N Engl J Med. (2018) ;378: (4):321–30. doi:10.1056/nejmoa1705971 |
[15] | Vandenberghe R , Rinne JO , Boada M , Katayama S , Scheltens P , Vellas B , et al. Bapineuzumab for mild to moderate Alzheimer’s disease in two global, randomized, phase 3 trials. Alzheimer’s Res Ther. (2016) ;8: (1). doi:10.1186/s13195-016-0189-7 |
[16] | Morris MC , Tangney CC , Wang Y , Sacks FM , Bennett DA , Aggarwal NT . MIND diet associated with reduced incidence of Alzheimer’s disease. Alzheimer’s Dement. (2015) ;11: (9):1007–14. doi:10.1016/j.jalz.2014.11.009 |
[17] | Hill E , Goodwill AM , Gorelik A , Szoeke C . Diet and biomarkers of Alzheimer’s disease: a systematic review and meta-analysis. Vol. 76, Neurobiology of Aging. Elsevier Inc.; 2019. p. 45-52. doi:10.1016/j.neurobiolaging.2018.12.008 |
[18] | Letenneur L , Proust-Lima C , Le Gouge A , Dartigues J , Barberger-Gateau P . Flavonoid Intake and Cognitive Decline over a 10-Year Period. Am J Epidemiol. (2007) ;165: (12):1364–71. doi:10.1093/aje/kwm036 |
[19] | Root M , Ravine E , Harper A . Flavonol Intake and Cognitive Decline in Middle-Aged Adults. J Med Food. (2015) ;18: (12):1327–32. doi:10.1089/jmf.2015.0010 |
[20] | Williams RJ , Spencer JPE , Rice-Evans C . Flavonoids: Antioxidants or signalling molecules? Free Radic Biol Med. (2004) ;36: (7):838–49. doi:10.1016/j.freeradbiomed.2004.01.001 |
[21] | Williams RJ , Spencer JPE . Flavonoids, cognition, and dementia: Actions, mechanisms, and potential therapeutic utility for Alzheimer disease. Free Radic Biol Med. (2012) ;52: :35–45. doi:10.1016/j.freeradbiomed.2011.09.010 |
[22] | Khan H , Marya , Amin S , Kamal MA , Patel S . Flavonoids as acetylcholinesterase inhibitors: Current therapeutic standing and future prospects. Vol. 101, Biomedicine and Pharmacotherapy. Elsevier Masson SAS; 2018. p. 860-70. doi:10.1016/j.biopha.2018.03.007 |
[23] | Zhang Z , Liu X , Schroeder JP , Chan C-B , Song M , Yu SP , et al. 7,8-Dihydroxyflavone Prevents Synaptic Loss and Memory Deficits in a Mouse Model of Alzheimer’s Disease. Neuropsychopharmacology. (2014) ;39: (3):638–50. doi:10.1038/npp.2013.243 |
[24] | Zhao L , Wang JL , Liu R , Li XX , Li JF , Zhang L . Neuroprotective, anti-amyloidogenic and neurotrophic effects of apigenin in an Alzheimer’s disease mouse model. Molecules. (2013) ;18: (8):9949–65. doi:10.3390/molecules18089949 |
[25] | Gu X-H , Xu L-J , Liu Z-Q , Wei B , Yang Y-J , Xu G-G , et al. The flavonoid baicalein rescues synaptic plasticity and memory deficits in a mouse model of Alzheimer’s disease. Behav Brain Res. (2016) ;311: :309–21. doi:10.1016/j.bbr.2016.05.052 |
[26] | Liang J , Lindemeyer • , Kerstin A , Shen Y , Héctor • , López-Valdés E , Martínez-Coria H , et al. Dihydromyricetin Ameliorates Behavioral Deficits and Reverses Neuropathology of Transgenic Mouse Models of Alzheimer’s Disease ((2014) ;39: (6):1171–81. doi:10.1007/s11064-014-1304-4 |
[27] | Sawmiller D , Habib A , Li S , Darlington D , Hou H , Tian J , et al. Diosmin reduces cerebral Aβ levels, tau hyperphosphorylation, neuroinflammation, and cognitive impairment in the 3xTg-AD mice. J Neuroimmunol. (2016) ;299: :98–106. doi:10.1016/j.jneuroim.2016.08.018 |
[28] | Walker JM , Klakotskaia D , Ajit D , Weisman GA , Wood WG , Sun GY , et al. Beneficial Effects of Dietary EGCG and Voluntary Exercise on Behavior in an Alzheimer’s Disease Mouse Model. J Alzheimer’s Dis. (2015) ;44: (2):561–72. doi:10.3233/jad-140981 |
[29] | Chang X , Rong C , Chen Y , Yang C , Hu Q , Mo Y , et al. (–)-Epigallocatechin-3-gallate attenuates cognitive deterioration in Alzheimer’s disease model mice by upregulating neprilysin expression. Exp Cell Res. (2015) ;334: (1):136–45. doi:10.1016/j.yexcr.2015.04.004 |
[30] | Jia N , Han K , Kong J-J , Zhang X-M , Sha S , Ren G-R , et al. (–)-Epigallocatechin-3-gallate alleviates spatial memory impairment in APP/PS1 mice by restoring IRS-1 signaling defects in the hippocampus. Mol Cell Biochem. (2013) ;380: (1-2):211–8. doi:10.1007/s11010-013-1675-x |
[31] | Liu M , Chen F , Sha L , Wang S , Tao L , Yao L , et al. (-)-Epigallocatechin-3-gallate ameliorates learning and memory deficits by adjusting the balance of TrkA/p75NTR signaling in APP/PS1 transgenic mice. Mol Neurobiol. (2014) ;49: (3):1350–63. doi:10.1007/s12035-013-8608-2 |
[32] | Mori T , Koyama N , Tan J , Segawa T , Maeda M , Town T . Combined treatment with the phenolics ()-epigallocatechin-3-gallate and ferulic acid improves cognition and reduces Alzheimer-like pathology in mice. J Biol Chem. (2019) ;294: (8):2714–31. doi:10.1074/jbc.ra118.004280 |
[33] | Cano A , Ettcheto M , Chang J-H , Barroso E , Espina M , Kühne BA , et al. Dual-drug loaded nanoparticles of Epigallocatechin-3-gallate (EGCG)/Ascorbic acid enhance therapeutic efficacy of EGCG in a APPswe/PS1dE9 Alzheimer’s disease mice model. J Control Release. (2019) ;301: :62–75. doi:10.1016/j.jconrel.2019.03.010 |
[34] | Zhong X , Liu M , Yao W , Du K , He M , Jin X , et al. Epigallocatechin-3-Gallate Attenuates Microglial Inflammation and Neurotoxicity by Suppressing the Activation of Canonical and Noncanonical Inflammasome via TLR4/NF-κB Pathway. Mol Nutr Food Res. 2019;1801230. doi:10.1002/mnfr.201801230 |
[35] | Rezai-Zadeh K , Arendash GW , Hou H , Fernandez F , Jensen M , Runfeldt M , et al. Green tea epigallocatechin-3-gallate (EGCG) reduces β-amyloid mediated cognitive impairment and modulates tau pathology in Alzheimer transgenic mice. Brain Res. (2008) ;1214: :177–87. doi:10.1016/j.brainres.2008.02.107 |
[36] | Lee JW , Lee YK , Ban JO , Ha TY , Yun YP , Han SB , et al. Green Tea (-)-Epigallocatechin-3-Gallate Inhibits β-Amyloid-Induced Cognitive Dysfunction through Modification of Secretase Activity via Inhibition of ERK and NF-κB Pathways in Mice. J Nutr. (2009) ;139: (10):1987–93. doi:10.3945/jn.109.109785 |
[37] | Lee Y-J , Choi D-Y , Yun Y-P , Han SB , Oh K-W , Hong JT . Epigallocatechin-3-gallate prevents systemic inflammation-induced memory deficiency and amyloidogenesis via its anti-neuroinflammatory properties. J Nutr Biochem. (2013) ;24: (1):298–310. doi:10.1016/j.jnutbio.2012.06.011 |
[38] | Zeng Y-Q , Wang Y-J , Zhou X-F . Effects of (-)Epicatechin on the Pathology of APP/PS1 Transgenic Mice. Front Neurol. (2014) ;5: :69. doi:10.3389/fneur.2014.00069 |
[39] | Cox CJ , Choudhry F , Peacey E , Perkinton MS , Richardson JC , Howlett DR , et al. Dietary (–)-epicatechin as a potent inhibitor of βγ-secretase amyloid precursor protein processing. Neurobiol Aging. (2015) ;36: (1):178–87. doi:10.1016/j.neurobiolaging.2014.07.032 |
[40] | He P , Yan S , Zheng J , Gao Y , Zhang S , Liu Z , et al. Eriodictyol Attenuates LPS-Induced Neuroinflammation, Amyloidogenesis, and Cognitive Impairments via the Inhibition of NF-κB in Male C57BL/6J Mice and BV2 Microglial Cells. J Agric Food Chem. (2018) ;66: (39):10205–14. doi:10.1021/acs.jafc.8b03731 |
[41] | Currais A , Prior M , Dargusch R , Armando A , Ehren J , Schubert D , et al. Modulation of p25 and inflammatory pathways by fisetin maintains cognitive function in Alzheimer’s disease transgenic mice. Aging Cell. (2014) ;13: (2):379–90. doi:10.1111/acel.12185 |
[42] | Fei H-X , Zhang Y-B , Liu T , Zhang X-J , Wu S-L . Neuroprotective effect of formononetin in ameliorating learning and memory impairment in mouse model of Alzheimer’s disease. Biosci Biotechnol Biochem. (2018) ;82: (1):57–64. doi:10.1080/09168451.2017.1399788 |
[43] | Wang D , Liu L , Zhu X , Wu W , Wang Y . Hesperidin Alleviates Cognitive Impairment, Mitochondrial Dysfunction and Oxidative Stress in a Mouse Model of Alzheimer’s Disease. Cell Mol Neurobiol. (2014) ;34: (8):1209–21. doi:10.1007/s10571-014-0098-x |
[44] | Li C , Zug C , Qu H , Schluesener H , Zhang Z . Hesperidin ameliorates behavioral impairments and neuropathology of transgenic APP/PS1 mice. Behav Brain Res. (2015) ;281: :32–42. doi:10.1016/j.bbr.2014.12.012 |
[45] | Jin F , Gong QH , Xu YS , Wang LN , Jin H , Li F , et al. Icariin, a phoshphodiesterase-5 inhibitor, improves learning and memory in APP/PS1 transgenic mice by stimulation of NO/cGMP signalling. Int J Neuropsychopharmacol. (2014) ;17: (6):871–81. doi:10.1017/s1461145713001533 |
[46] | Zhu T , Zhang F , Li H , He Y , Zhang G , Huang N , et al. Long-term icariin treatment ameliorates cognitive deficits via CD4+ T cell-mediated immuno-inflammatory responses in APP/PS1 mice. Clin Interv Aging. (2019) ;14: :817–26. doi:10.2147/cia.s208068 |
[47] | Li F , Zhang Y , Lu X , Shi J , Gong Q . Icariin improves the cognitive function of APP/PS1 mice via suppressing endoplasmic reticulum stress. Life Sci. 2019;234. doi:10.1016/j.lfs.2019.116739 |
[48] | Zhang ZY , Li C , Zug C , Schluesener HJ . Icariin ameliorates neuropathological changes, TGF-β1 accumulation and behavioral deficits in a mouse model of cerebral amyloidosis. PLoS One. (2014) ;9: (8). doi:10.1371/journal.pone.0104616 |
[49] | Chen Y , Han S , Huang X , Ni J , He X . The protective effect of icariin on mitochondrial transport and distribution in primary hippocampal neurons from 3×Tg-AD mice. Int J Mol Sci. (2016) ;17: (2). doi:10.3390/ijms17020163 |
[50] | Li F , Dong HX , Gong QH , Wu Q , Jin F , Shi JS . Icariin decreases both APP and Aβ levels and increases neurogenesis in the brain of Tgmice. Neuroscience. (2015) ;304: :29–35. doi:10.1016/j.neuroscience.2015.06.010 |
[51] | Zhang L , Shen C , Chu J , Zhang R , Li Y , Li L . Icariin decreases the expression of APP and BACE-1 and reduces the β-amyloid burden in an APP transgenic mouse model of Alzheimer’s disease. Int J Biol Sci. (2014) ;10: (2):181–91. doi:10.7150/ijbs.6232 |
[52] | Liu RT , Tang JT , Zou LB , Fu JY , Lu QJ . Liquiritigenin attenuates the learning and memory deficits in an amyloid protein precursor transgenic mouse model and the underlying mechanisms. Eur J Pharmacol. (2011) ;669: (1-3):76–83. doi:10.1016/j.ejphar.2011.07.051 |
[53] | Hamaguchi T , Ono K , Murase A , Yamada M . Phenolic compounds prevent Alzheimer’s pathology through different effects on the amyloid-β aggregation pathway. Am J Pathol. (2009) ;175: (6):2557–65. doi:10.2353/ajpath.2009.090417 |
[54] | Wang D , Gao K , Li X , Shen X , Zhang X , Ma C , et al. Long-term naringin consumption reverses a glucose uptake defect and improves cognitive deficits in a mouse model of Alzheimer’s disease. Pharmacol Biochem Behav. (2012) ;102: (1):13–20. doi:10.1016/j.pbb.2012.03.013 |
[55] | Xu P xin , Wang S wei , Yu X lin , Su Y jing , Wang T , Zhou W wei , et al. Rutin improves spatial memory in Alzheimer’s disease transgenic mice by reducing Aβ oligomer level and attenuating oxidative stress and neuroinflammation. Behav Brain Res. (2014) ;264: :173–80. doi:10.1016/j.bbr.2014.02.002 |
[56] | Zeng YQ , Cui YB , Gu JH , Liang C , Zhou XF . Scutellarin mitigates Aβ-induced neurotoxicity and improves behavior impairments in AD mice. Molecules. (2018) ;23: (4). doi:10.3390/molecules23040869 |
[57] | Zhang X , Hu J , Zhong L , Wang N , Yang L , Liu C-C , et al. Quercetin stabilizes apolipoprotein E and reduces brain Aβ levels in amyloid model mice. Neuropharmacology. (2016) ;108: :179–92. doi:10.1016/j.neuropharm.2016.04.032 |
[58] | Woo M , Kim MJ , Song YO . Bioactive Compounds in Kimchi Improve the Cognitive and Memory Functions Impaired by Amyloid Beta. Nutrients. (2018) ;10: (10). doi:10.3390/nu10101554 |
[59] | Lu Y , Liu Q , Yu Q . Quercetin enrich diet during the early-middle not middle-late stage of alzheimer’s disease ameliorates cognitive dysfunction. Am J Transl Res. (2018) ;10: (4):1237–46. |
[60] | Obregon DF , Rezai-Zadeh K , Bai Y , Sun N , Hou H , Ehrhart J , et al. ADAM10 Activation Is Required for Green Tea (–)-Epigallocatechin-3-gallate-induced α-Secretase Cleavage of Amyloid Precursor Protein. J Biol Chem. (2006) ;281: (24):16419–27. doi:10.1074/jbc.m600617200 |
[61] | Rezai-Zadeh K , Shytle D , Sun N , Mori T , Hou H , Jeanniton D , et al. Green tea epigallocatechin-3-gallate (EGCG) modulates amyloid precursor protein cleavage and reduces cerebral amyloidosis in Alzheimer transgenic mice. J Neurosci. (2005) ;25: (38):8807–14. doi:10.1523/jneurosci.1521-05.2005 |
[62] | Fernandez JW , Rezai-Zadeh K , Obregon D , Tan J . EGCG functions through estrogen receptor-mediated activation of ADAM10 in the promotion of non-amyloidogenic processing of APP. FEBS Lett. (2010) ;584: (19):4259–67. doi:10.1016/j.febslet.2010.09.022 |
[63] | Zhang S-Q , Obregon D , Ehrhart J , Deng J , Tian J , Hou H , et al. Baicalein reduces β-amyloid and promotes nonamyloidogenic amyloid precursor protein processing in an Alzheimer’s disease transgenic mouse model. J Neurosci Res. (2013) ;91: (9):1239–46. doi:10.1002/jnr.23244 |
[64] | Marcade M , Bourdin J , Loiseau N , Peillon H , Rayer A , Drouin D , et al. Etazolate, a neuroprotective drug linking GABAA receptor pharmacology to amyloid precursor protein processing. J Neurochem. (2008) ;106: (1):392–404. doi:10.1111/j.1471-4159.2008.05396.x |
[65] | De Carvalho RSM , Duarte FS , De Lima TCM . Involvement of GABAergic non-benzodiazepine sites in the anxiolytic-like and sedative effects of the flavonoid baicalein in mice. Behav Brain Res. (2011) ;221: (1):75–82. doi:10.1016/j.bbr.2011.02.038 |
[66] | Tao YH , Jiang DY , Xu HB , Yang XL . Inhibitory effect of Erigeron breviscapus extract and its flavonoid components on GABA shunt enzymes. Phytomedicine. (2008) ;15: (1-2):92–7. doi:10.1016/j.phymed.2007.06.009 |
[67] | Martín-Aragón S , Jiménez-Aliaga KL , Benedí J , Bermejo-Bescós P . Neurohormetic responses of quercetin and rutin in a cell line over-expressing the amyloid precursor protein (APPswe cells). Phytomedicine. (2016) ;23: (12):1285–94. doi:10.1016/j.phymed.2016.07.007 |
[68] | Grimm MOW , Mett J , Stahlmann CP , Haupenthal VJ , Zimmer VC , Hartmann T . Neprilysin and Aβ clearance: Impact of the APP intracellular domain in NEP regulation and implications in Alzheimer’s disease. Vol. 5, Frontiers in Aging Neuroscience. Frontiers Media SA; 2013. p. 98. doi:10.3389/fnagi.2013.00098 |
[69] | Yamamoto N , Shibata M , Ishikuro R , Tanida M , Taniguchi Y , Ikeda-Matsuo Y , et al. Epigallocatechin gallate induces extracellular degradation of amyloid β-protein by increasing neprilysin secretion from astrocytes through activation of ERK and PI3K pathways. Neuroscience. (2017) ;362: :70–8. doi:10.1016/j.neuroscience.2017.08.030 |
[70] | Lim HJ , Shim SB , Jee SW , Lee SH , Lim CJ , Hong JT , et al. Green tea catechin leads to global improvement among Alzheimer’s disease-related phenotypes in NSE/hAPP-C105 Tg mice. J Nutr Biochem. (2013) ;24: (7):1302–13. doi:10.1016/j.jnutbio.2012.10.005 |
[71] | Lemkul JA , Bevan DR . Morin Inhibits the Early Stages of Amyloid β-Peptide Aggregation by Altering Tertiary and Quaternary Interactions to Produce “Off-Pathway” Structures. Biochemistry. (2012) ;51: (30):5990–6009. doi:10.1021/bi300113x |
[72] | Lemkul JA , Bevan DR . Destabilizing alzheimer s Aβ42 protofibrils with morin: Mechanistic insights from molecular dynamics simulations. Biochemistry. (2010) ;49: (18):3935–46. doi:10.1021/bi1000855 |
[73] | Ono K , Yoshiike Y , Takashima A , Hasegawa K , Naiki H , Yamada M . Potent anti-amyloidogenic and fibril-destabilizing effects of polyphenols in vitro: implications for the prevention and therapeutics of Alzheimer’s disease. J Neurochem. (2003) ;87: (1):172–81. doi:10.1046/j.1471-4159.2003.01976.x |
[74] | Ladiwala ARA , Dordick JS , Tessier PM . Aromatic Small Molecules Remodel Toxic Soluble Oligomers of Amyloid through Three Independent Pathways. 2010; doi:10.1074/jbc.m110.173856 |
[75] | Hanaki M , Murakami K , Akagi KI , Irie K . Structural insights into mechanisms for inhibiting amyloid β42 aggregation by non-catechol-type flavonoids. Bioorganic Med Chem. (2016) ;24: (2):304–13. doi:10.1016/j.bmc.2015.12.021 |
[76] | Guzzi C , Colombo L , Luigi A De , Salmona M , Nicotra F , Airoldi C . Flavonoids and Their Glycosides as Anti-amyloidogenic Compounds: Aβ1-42 Interaction Studies to Gain New Insights into Their Potential for Alzheimer’s Disease Prevention and Therapy. Chem –An Asian J. (2017) ;12: (1):67–75. doi:10.1002/asia.201601291 |
[77] | Gao L , Tian M , Zhao H-Y , Xu Q-Q , Huang Y-M , Si Q-C , et al. TrkB activation by 7, 8-dihydroxyflavone increases synapse AMPA subunits and ameliorates spatial memory deficits in a mouse model of Alzheimer’s disease. J Neurochem. (2016) ;136: (3):620–36. doi:10.1111/jnc.13432 |
[78] | Chen C , Li X , Gao P , Tu Y , Zhao M , Li J , et al. Baicalin attenuates Alzheimer-like pathological changes and memory deficits induced by amyloid β1-42 protein. Metab Brain Dis. (2015) ;30: (2):537–44. doi:10.1007/s11011-014-9601-9 |
[79] | Song N , Zhang L , Chen W , Zhu H , Deng W , Han Y , et al. Cyanidin 3-O-β-glucopyranoside activates peroxisome proliferator-activated receptor-γ and alleviates cognitive impairment in the APPswe/PS1ΔE9 mouse model. Biochim Biophys Acta – Mol Basis Dis. (2016) ;1862: (9):1786–800. doi:10.1016/j.bbadis.2016.05.016 |
[80] | Hong Y , An Z . Hesperidin attenuates learning and memory deficits in APP/PS1 mice through activation of Akt/Nrf2 signaling and inhibition of RAGE/NF-κB signaling. Arch Pharm Res. (2018) ;41: (6):655–63. doi:10.1007/s12272-015-0662-z |
[81] | Urano T , Tohda C . Icariin improves memory impairment in Alzheimer’s disease model mice (5xFAD) and attenuates amyloid β-induced neurite atrophy. Phyther Res. (2010) ;24: (11):1658–63. doi:10.1002/ptr.3183 |
[82] | Wang D-M , Yang Y-J , Zhang L , Zhang X , Guan F-F , Zhang L-F . Naringin Enhances CaMKII Activity and Improves Long-Term Memory in a Mouse Model of Alzheimer’s Disease. Int J Mol Sci. (2013) ;14: (3):5576–86. doi:10.3390/ijms14035576 |
[83] | Liu R , Li J ze , Song J ke , Zhou D , Huang C , Bai X yu , et al. Pinocembrin improves cognition and protects the neurovascular unit in Alzheimer related deficits. Neurobiol Aging. (2014) ;35: (6):1275–85. doi:10.1016/j.neurobiolaging.2013.12.031 |
[84] | Zhou Y , Xie N , Li L , Zou Y , Zhang X , Dong M . Puerarin alleviates cognitive impairment and oxidative stress in APP/PS1 transgenic mice. Int J Neuropsychopharmacol. (2014) ;17: (04):635–44. doi:10.1017/s146114571300148x |
[85] | Choi JY , Lee JM , Lee DG , Cho S , Yoon Y-H , Cho EJ , et al. The n -Butanol Fraction and Rutin from Tartary Buckwheat Improve Cognition and Memory in an In Vivo Model of Amyloid- β -Induced Alzheimer’s Disease. J Med Food. (2015) ;18: (6):631–41. doi:10.1089/jmf.2014.3292 |
[86] | Kanai Y , Hirokawa N . Sorting mechanisms of Tau and MAP2 in neurons: Suppressed axonal transit of MAP2 and locally regulated microtubule binding. Neuron. (1995) ;14: (2):421–32. doi:10.1016/0896-6273(95)90298-8 |
[87] | Hirokawa N , Funakoshi T , Sato-Harada R , Kanai Y . Selective stabilization of tau in axons and microtubule-associated protein 2C in cell bodies and dendrites contributes to polarized localization of cytoskeletal proteins in mature neurons. J Cell Biol. (1996) ;132: (4):667–79. doi:10.1083/jcb.132.4.667 |
[88] | Grundke-Iqbal I , Iqbal K , Tung YC . Abnormal phosphorylation of the microtubule-associated protein τ (tau) in Alzheimer cytoskeletal pathology. Proc Natl Acad Sci U S A. (1986) ;83: (13):44913–7. doi:10.1073/pnas.83.13.4913 |
[89] | Ihara Y , Nukina N , Miura R , Ogawara M . Phosphorylated tau protein is integrated into paired helical filaments in alzheimer’s disease. J Biochem. (1986) ;99: (6):1807–10. doi:10.1093/oxfordjournals.jbchem.a135662 |
[90] | Eftekharzadeh B , Daigle JG , Kapinos LE , Coyne A , Schiantarelli J , Carlomagno Y , et al. Tau Protein Disrupts Nucleocytoplasmic Transport in Alzheimer’s Disease. Neuron. (2018) ;99: (5):925–940e7. doi:10.1016/j.neuron.2018.07.039 |
[91] | Ittner A , Ittner LM . Dendritic Tau in Alzheimer’s Disease. Neuron. (2018) ;99: (1):13–27. doi:10.1016/j.neuron.2018.06.003 |
[92] | Li C , Götz J . Somatodendritic accumulation of Tau in Alzheimer’s disease is promoted by Fyn-mediated local protein translation. EMBO J. (2017) ;36: (21):3120–38. doi:10.15252/embj.201797724 |
[93] | Hanger DP , Hughes K , Woodgett JR , Brion JP , Anderton BH . Glycogen synthase kinase-3 induces Alzheimer’s disease-like phosphorylation of tau: Generation of paired helical filament epitopes and neuronal localisation of the kinase. Neurosci Lett. (1992) ;147: (1):58–62. doi:10.1016/0304-3940(92)90774-2 |
[94] | Mandelkow E-M , Drewes G , Biernat J , Gustke N , Van Lint J , Vandenheede JR , et al. Glycogen synthase kinase-3 and the Alzheimer-like state of microtubule-associated protein tau. FEBS Lett. (1992) ;314: (3):315–21. doi:10.1016/0014-5793(92)81496-9 |
[95] | Baumann K , Mandelkow EM , Biernat J , Piwnica-Worms H , Mandelkow E . Abnormal Alzheimer-like phosphorylation of tau-protein by cyclin-dependent kinases cdk2 and cdk5. FEBS Lett. (1993) ;336: (3):417–24. doi:10.1016/0014-5793(93)80849-p |
[96] | Noble W , Olm V , Takata K , Casey E , Mary O , Meyerson J , et al. Cdk5 Is a Key Factor in Tau Aggregation and Tangle Formation In Vivo. Neuron. (2003) ;38: (4):555–65. doi:10.1016/s0896-6273(03)00259-9 |
[97] | Drewes G , Lichtenberg-Kraag B , Döring F , Mandelkow EM , Biernat J , Goris J , et al. Mitogen activated protein (MAP) kinase transforms tau protein into an Alzheimer-like state. EMBO J. (1992) ;11: (6):2131–8. doi:10.1002/j.1460-2075.1992.tb05272.x |
[98] | Reynolds CH , Betts JC , Blackstock WP , Nebreda AR , Anderton BH . Phosphorylation sites on tau identified by nanoelectrospray mass spectrometry: Differences in vitro between the mitogen-activated protein kinases ERK2, c-Jun N-terminal kinase and P38, and glycogen synthase kinase- 3β. J Neurochem. (2000) ;74: (4):1587–95. doi:10.1046/j.1471-4159.2000.0741587.x |
[99] | Sperbera BR , Leight S , Goedert M , Lee VMY . Glycogen synthase kinase-3β phosphorylates tau protein at multiple sites in intact cells. Neurosci Lett. (1995) ;197: (2):149–53. doi:10.1016/0304-3940(95)11902-9 |
[100] | Wang J-Z , Xia Y-Y , Grundke-Iqbal I , Iqbal K . Abnormal Hyperphosphorylation of Tau: Sites, Regulation, and Molecular Mechanism of Neurofibrillary Degeneration. Perry G, Zhu X, Smith MA, Sorensen A, Avila J, editors. J Alzheimer’s Dis. (2013) ;33: (s1):S123–39. doi:10.3233/jad-2012-129031 |
[101] | Wang JZ , Wu Q , Smith A , Grundke-Iqbal I , Iqbal K . Tau is phosphorylated by GSK-3 at several sites found in Alzheimer disease and its biological activity markedly inhibited only after it is prephosphorylated by A-kinase. FEBS Lett. (1998) ;436: (1):28–34. doi:10.1016/s0014-5793(98)01090-4 |
[102] | Yoshida H , Hastie CJ , McLauchlan H , Cohen P , Goedert M . Phosphorylation of microtubule-associated protein tau by isoforms of c-Jun N-terminal kinase (JNK). J Neurochem. (2004) ;90: (2):352–8. doi:10.1111/j.1471-4159.2004.02479.x |
[103] | Liu F , Iqbal K , Grundke-Iqbal I , Gong C-X . Involvement of aberrant glycosylation in phosphorylation of tau by cdk5 and GSK-3β. FEBS Lett. (2002) ;530: (1-3):209–14. doi:10.1016/s0014-5793(02)03487-7 |
[104] | Lovestone S , Reynolds CH , Latimer D , Davis DR , Anderton BH , Gallo JM , et al. Alzheimer’s disease-like phosphorylation of the microtubule-associated protein tau by glycogen synthase kinase-3 in transfected mammalian cells. Curr Biol. (1994) ;4: (12):1077–86. doi:10.1016/s0960-9822(00)00246-3 |
[105] | Wang J-Z , Grundke-Iqbal I , Iqbal K . Kinases and phosphatases and tau sites involved in Alzheimer neurofibrillary degeneration. Eur J Neurosci. (2007) ;25: (1):59–68. doi:10.1111/j.1460-9568.2006.05226.x |
[106] | Qi H , Prabakaran S , Cantrelle FX , Chambraud B , Gunawardena J , Lippens XG , et al. Characterization of neuronal tau protein as a target of extracellular signal-regulated kinase. J Biol Chem. (2016) ;291: (14):7742–53. doi:10.1074/jbc.m115.700914 |
[107] | Kimura T , Ishiguro K , Hisanaga SI . Physiological and pathological phosphorylation of tau by Cdk5. Vol. 7, Frontiers in Molecular Neuroscience. Frontiers Research Foundation; 2014. doi:10.3389/fnmol.2014.00065 |
[108] | Ploia C , Antoniou X , Sclip A , Grande V , Cardinetti D , Colombo A , et al. JNK Plays a Key Role in Tau Hyperphosphorylation in Alzheimer’s Disease Models. J Alzheimer’s Dis. (2011) ;26: (2):315–29. doi:10.3233/jad-2011-110320 |
[109] | Johnson GVW , Hartigan JA . Tau protein in normal and Alzheimer’s disease brain: An update. Vol. 1, Journal of Alzheimer’s Disease. IOS Press; 1999. p. 329-51. doi:10.3233/jad-1999-14-512 |
[110] | Reynolds CH , Utton MA , Gibb GM , Yates A , Anderton BH . Stress-Activated Protein Kinase/c-Jun N-Terminal Kinase Phosphorylates τ Protein. J Neurochem. (1997) ;68: (4):1736–44. doi:10.1046/j.1471-4159.1997.68041736.x |
[111] | Reynolds CH , Betts JC , Blackstock WP , Nebreda AR , Anderton BH . Phosphorylation Sites on Tau Identified by Nanoelectrospray Mass Spectrometry. J Neurochem. (2002) ;74: (4):1587–95. doi:10.1046/j.1471-4159.2000.0741587.x |
[112] | Schroeter H , Spencer JP , Rice-Evans C , Williams RJ . Flavonoids protect neurons from oxidized low-density-lipoprotein-induced apoptosis involving c-Jun N-terminal kinase (JNK), c-Jun and caspase-3. Biochem J. (2001) ;358: (Pt 3):547–57. doi:10.1046/j.1471-4159.2000.0741587.x |
[113] | Jang S , Kelley KW , Johnson RW . Luteolin reduces IL-6 production in microglia by inhibiting JNK phosphorylation and activation of AP-1. Proc Natl Acad Sci U S A. (2008) ;105: (21):7534–9. doi:10.1073/pnas.0802865105 |
[114] | Vauzour D , Vafeiadou K , Rice-Evans C , Williams RJ , Spencer JPE . Activation of pro-survival Akt and ERK1/2 signalling pathways underlie the anti-apoptotic effects of flavanones in cortical neurons. J Neurochem. (2007) ;103: (4):1355–67. doi:10.1111/j.1471-4159.2007.04841.x |
[115] | Park SE , Sapkota K , Kim S , Kim H , Kim SJ . Kaempferol acts through mitogen-activated protein kinases and protein kinase B/AKT to elicit protection in a model of neuroinflammation in BV2 microglial cells. Br J Pharmacol. (2011) ;164: (3):1008–25. doi:10.1111/j.1476-5381.2011.01389.x |
[116] | Liu R , Wu CX , Zhou D , Yang F , Tian S , Zhang L , et al. Pinocembrin protects against β-amyloid-induced toxicity in neurons through inhibiting receptor for advanced glycation end products (RAGE)-independent signaling pathways and regulating mitochondrion-mediated apoptosis. BMC Med. 2012;10. doi:10.1186/1741-7015-10-105 |
[117] | Currais A , Farrokhi C , Dargusch R , Armando A , Quehenberger O , Schubert D , et al. Fisetin Reduces the Impact of Aging on Behavior and Physiology in the Rapidly Aging SAMP8 Mouse. Journals Gerontol Ser A. (2018) ;73: (3):299–307. doi:10.1093/gerona/glx104 |
[118] | Muhammad T , Ikram M , Ullah R , Rehman S , Kim M . Hesperetin, a Citrus Flavonoid, Attenuates LPS-Induced Neuroinflammation, Apoptosis and Memory Impairments by Modulating TLR4/NF-κB Signaling. Nutrients. (2019) ;11: (3):648. doi:10.3390/nu11030648 |
[119] | Anderton BH , Betts J , Blackstock WP , Brion JP , Chapman S , Connell J , et al. Sites of phosphorylation in tau and factors affecting their regulation. Biochem Soc Symp. (2001) ;67: :73–80. doi:10.1042/bss0670073 |
[120] | Quintanilla RA , Orellana DI , González-Billault C , Maccioni RB . Interleukin-6 induces Alzheimer-type phosphorylation of tau protein by deregulating the cdk5/p35 pathway. Exp Cell Res. (2004) ;295: (1):245–57. doi:10.1016/j.yexcr.2004.01.002 |
[121] | Kelleher I , Garwood C , Hanger DP , Anderton BH , Noble W . Kinase activities increase during the development of tauopathy in htau mice. J Neurochem. (2007) ;103: (6):2256–67. doi:10.1111/j.1471-4159.2007.04930.x |
[122] | Atzori C , Ghetti B , Piva R , Srinivasan AN , Zolo P , Delisle MB , et al. Activation of the JNK/p38 Pathway Occurs in Diseases Characterized by Tau Protein Pathology and Is Related to Tau Phosphorylation But Not to Apoptosis. J Neuropathol Exp Neurol. (2001) ;60: (12):1190–7. doi:10.1093/jnen/60.12.1190 |
[123] | Ferrer I , Hernández I , Puig B , Jesú s Rey M , Ezquerra M , Tolosa E , et al. Ubiquitin-negative mini-pick-like bodies in the dentate gyrus in p301l tauopathy. J Alzheimer’s Dis. (2004) ;5: (6):445–54. doi:10.3233/jad-2003-5604 |
[124] | Bhaskar K , Konerth M , Kokiko-Cochran ON , Cardona A , Ransohoff RM , Lamb BT . Regulation of tau pathology by the microglial fractalkine receptor. Neuron. (2010) ;68: (1):19–31. doi:10.1016/j.neuron.2010.08.023 |
[125] | Li Y , Liu L , Barger SW , Griffin WST . Interleukin-1 mediates pathological effects of microglia on tau phosphorylation and on synaptophysin synthesis in cortical neurons through a p38-MAPK pathway. J Neurosci. (2003) ;23: (5):1605–11. doi:10.1523/jneurosci.23-05-01605.2003 |
[126] | Ghosh S , Wu MD , Shaftel SS , Kyrkanides S , LaFerla FM , Olschowka JA , et al. Sustained interleukin-1β overexpression exacerbates tau pathology despite reduced amyloid burden in an Alzheimer’s mouse model. J Neurosci. (2013) ;33: (11):5053–64. doi:10.1523/jneurosci.4361-12.2013 |
[127] | Carvalho D , Paulino M , Polticelli F , Arredondo F , Williams RJ , Abin-Carriquiry JA . Structural evidence of quercetin multi-target bioactivity: A reverse virtual screening strategy. Eur J Pharm Sci. (2017) ;106: :393–403. doi:10.1016/j.ejps.2017.06.028 |
[128] | Hwang SL , Yen GC . Modulation of Akt, JNK, and p38 activation is involved in citrus flavonoid-mediated cytoprotection of PC12 cells challenged by hydrogen peroxide. J Agric Food Chem. (2009) ;57: (6):2576–82. doi:10.1021/jf8033607 |
[129] | Vafeiadou K , Vauzour D , Lee HY , Rodriguez-Mateos A , Williams RJ , Spencer JPE . The citrus flavanone naringenin inhibits inflammatory signalling in glial cells and protects against neuroinflammatory injury. Arch Biochem Biophys. (2009) ;484: (1):100–9. doi:10.1016/j.abb.2009.01.016 |
[130] | Liu CM , Ma JQ , Liu SS , Zheng GH , Feng ZJ , Sun JM . Proanthocyanidins improves lead-induced cognitive impairments by blocking endoplasmic reticulum stress and nuclear factor-κB-mediated inflammatory pathways in rats. Food Chem Toxicol. (2014) ;72: :295–302. doi:10.1016/j.fct.2014.07.033 |
[131] | Zhang Z , Wu H , Huang H . Epicatechin Plus Treadmill Exercise are Neuroprotective Against Moderate-stage Amyloid Precursor Protein/Presenilin 1 Mice. Pharmacogn Mag. (2016) ;12: (Suppl 2):S139–46. doi:10.4103/0973-1296.182174 |
[132] | Kim K , Cha JS , Kim JS , Ahn J , Ha NC , Cho HS . Crystal structure of GSK3β in complex with the flavonoid, morin. Biochem Biophys Res Commun. (2018) ;504: (2):519–24. doi:10.1016/j.bbrc.2018.08.182 |
[133] | Gong EJ , Park HR , Kim ME , Piao S , Lee E , Jo DG , et al. Morin attenuates tau hyperphosphorylation by inhibiting GSK3β. Neurobiol Dis. (2011) ;44: (2):223–30. doi:10.1016/j.nbd.2011.07.005 |
[134] | Johnson JL , Rupasinghe SG , Stefani F , Schuler MA , Gonzalez De Mejia E . Citrus flavonoids luteolin, apigenin, and quercetin inhibit glycogen synthase kinase-3β enzymatic activity by lowering the interaction energy within the binding cavity. J Med Food. (2011) ;14: (4):325–33. doi:10.1089/jmf.2010.0310 |
[135] | Ter Haar E , Coll JT , Austen DA , Hsiao HM , Swenson L , Jain J . Structure of GSK3β reveals a primed phosphorylation mechanism. Nat Struct Biol. (2001) ;8: (7):593–6. doi:10.1038/89624 |
[136] | Cho JH , Johnson GVW . Glycogen synthase kinase 3β phosphorylates tau at both primed and unprimed sites: Differential impact on microtubule binding. J Biol Chem. (2003) ;278: (1):187–93. doi:10.1074/jbc.m206236200 |
[137] | Dajani R , Fraser E , Roe SM , Young N , Good V , Dale TC , et al. Crystal structure of glycogen synthase kinase 3β: Structural basis for phosphate-primed substrate specificity and autoinhibition. Cell. (2001) ;105: (6):721–32. doi:10.1016/s0092-8674(01)00374-9 |
[138] | Bax B , Carter PS , Lewis C , Guy AR , Bridges A , Tanner R , et al. The structure of phosphorylated GSK-3β complexed with a peptide, FRATtide, that inhibits β-catenin phosphorylation. Structure. (2001) ;9: (12):1143–52. doi:10.1016/s0969-2126(01)00679-7 |
[139] | Santa-Maria I , Diaz-Ruiz C , Ksiezak-Reding H , Chen A , Ho L , Wang J , et al. GSPE interferes with tau aggregation in vivo: implication for treating tauopathy. Neurobiol Aging. (2012) ;33: (9):2072–81. doi:10.1016/j.neurobiolaging.2011.09.027 |
[140] | Wang J , Santa-Maria I , Ho L , Ksiezak-Reding H , Ono K , Teplow DB , et al. Grape Derived Polyphenols Attenuate Tau Neuropathology in a Mouse Model of Alzheimer’s Disease. J Alzheimer’s Dis. (2010) ;22: :653–61. doi:10.3233/jad-2010-101074 |
[141] | Ferrer I , Blanco R , Carmona M , Puig B . Phosphorylated mitogen-activated protein kinase (MAPK/ERK-P), protein kinase of 38kDa (p38-P), stress-activated protein kinase (SAPK/JNK-P), and calcium/calmodulin-dependent kinase II (CaM kinase II) are differentially expressed in tau deposits in neurons and glial cells in tauopathies. J Neural Transm. (2001) ;108: (12):1397–415. doi:10.1007/s007020100016 |
[142] | Pei J-J , Braak H , An W-L , Winblad B , Cowburn RF , Iqbal K , et al. Up-regulation of mitogen-activated protein kinases ERK1/2 and MEK1/2 is associated with the progression of neurofibrillary degeneration in Alzheimer’s disease. Brain Res Mol Brain Res. (2002) ;109: (1-2):45–55. doi:10.1016/s0169-328x(02)00488-6 |
[143] | Sonawane SK , Balmik AA , Boral D , Ramasamy S , Chinnathambi S . Baicalein suppresses Repeat Tau fibrillization by sequestering oligomers. Arch Biochem Biophys. (2019) ;675: :108119. doi:10.1016/j.abb.2019.108119 |
[144] | Ho L , Yemul S , Wang J , Pasinetti GM . Grape seed polyphenolic extract as a potential novel therapeutic agent in tauopathies. J Alzheimers Dis. (2009) ;16: (2):433–9. doi:10.3233/jad-2009-0969 |
[145] | von Bergen M , Friedhoff P , Biernat J , Heberle J , Mandelkow EM , Mandelkow E . Assembly of tau protein into Alzheimer paired helical filaments depends on a local sequence motif ((306)VQIVYK(311)) forming beta structure. Proc Natl Acad Sci U S A. (2000) ;97: (10):5129–34. doi:10.1073/pnas.97.10.5129 |
[146] | Pasinetti GM , Ksiezak-Reding H , Santa-Maria I , Wang J , Ho L . Development of a grape seed polyphenolic extract with anti-oligomeric activity as a novel treatment in progressive supranuclear palsy and other tauopathies. J Neurochem. (2010) ;114: (6):1557–68. doi:10.1111/j.1471-4159.2010.06875.x |
[147] | George RC , Lew J , Graves DJ . Interaction of Cinnamaldehyde and Epicatechin with Tau: Implications of Beneficial Effects in Modulating Alzheimer’s Disease Pathogenesis. J Alzheimer’s Dis. (2013) ;36: (1):21–40. doi:10.3233/jad-122113 |
[148] | Taniguchi S , Suzuki N , Masuda M , Hisanaga S , Iwatsubo T , Goedert M , et al. Inhibition of heparin-induced tau filament formation by phenothiazines, polyphenols, and porphyrins. J Biol Chem. (2005) ;280: (9):7614–23. doi:10.1074/jbc.m408714200 |
[149] | Wobst HJ , Sharma A , Diamond MI , Wanker EE , Bieschke J . The green tea polyphenol (–)-epigallocatechin gallate prevents the aggregation of tau protein into toxic oligomers at substoichiometric ratios. FEBS Lett. (2015) ;589: (1):77–83. doi:10.1016/j.febslet.2014.11.026 |
[150] | Heneka MT , Carson MJ , Khoury J El , Landreth GE , Brosseron F , Feinstein DL , et al. Neuroinflammation in Alzheimer’s disease. Vol. 14, The Lancet Neurology. Lancet Publishing Group; 2015. p. 388-405. doi:10.1016/s1474-4422(15)70016-5 |
[151] | Efthymiou AG , Goate AM . Late onset Alzheimer’s disease genetics implicates microglial pathways in disease risk. Vol. 12, Molecular Neurodegeneration. BioMed Central Ltd.; 2017. doi:10.1186/s13024-017-0184-x |
[152] | Srinivasan K , Friedman BA , Etxeberria A , Huntley MA , Brug MP van der , Foreman O , et al. Alzheimer’s patient brain myeloid cells exhibit enhanced aging and unique transcriptional activation. bioRxiv. 2019;610345. doi:10.1101/610345 |
[153] | Mathys H , Davila-Velderrain J , Peng Z , Gao F , Mohammadi S , Young JZ , et al. Single-cell transcriptomic analysis of Alzheimer’s disease. Nature. (2019) ;570: (7761):332–7. doi:10.1038/s41586-019-1195-2 |
[154] | Asai H , Ikezu S , Tsunoda S , Medalla M , Luebke J , Haydar T , et al. Depletion of microglia and inhibition of exosome synthesis halt tau propagation. Nat Neurosci. (2015) ;18: (11):1584–93. doi:10.1038/nn.4132 |
[155] | Tzioras M , Daniels MJ , King D , Popovic K , Holloway RK , Stevenson AJ , et al. Altered synaptic ingestion by human microglia in Alzheimer’s disease. bioRxiv. 2019;795930. doi:10.1101/795930 |
[156] | Dejanovic B , Huntley MA , De Mazière A , Meilandt WJ , Wu T , Srinivasan K , et al. Changes in the Synaptic Proteome in Tauopathy and Rescue of Tau-Induced Synapse Loss by C1q Antibodies. Neuron. (2018) ;100: (6):1322–1336. doi:10.1016/j.neuron.2018.10.014 |
[157] | Hong S , Beja-Glasser VF , Nfonoyim BM , Frouin A , Li S , Ramakrishnan S , et al. Complement and microglia mediate early synapse loss in Alzheimer mouse models. Science (80-). (2016) ;352: (6286):712–6. doi:10.1126/science.aad8373 |
[158] | Leyns CEG , Holtzman DM . Glial contributions to neurodegeneration in tauopathies. Vol. 12, Molecular Neurodegeneration. BioMed Central Ltd.; 2017. doi:10.1186/s13024-017-0192-x |
[159] | Yoshiyama Y , Higuchi M , Zhang B , Huang S-M , Iwata N , Saido TC , et al. Synapse loss and microglial activation precede tangles in a P301S tauopathy mouse model. Neuron. (2007) ;53: (3):337–51. doi:10.1016/j.neuron.2007.01.010 |
[160] | Felsky D , Roostaei T , Nho K , Risacher SL , Bradshaw EM , Petyuk V , et al. Neuropathological correlates and genetic architecture of microglial activation in elderly human brain. Nat Commun. (2019) ;10: (1). doi:10.1038/s41467-018-08279-3 |
[161] | Sierksma A , Lu A , Salta E , Mancuso R , Zoco J , Blum D , et al. Novel Alzheimer risk genes determine the microglia response to amyloid-β but not to TAU pathology. bioRxiv. 2019;491902. doi:10.1101/491902 |
[162] | Ising C , Venegas C , Zhang S , Scheiblich H , Schmidt S V , Vieira-Saecker A . et al NLRP3 inflammasome activation drives tau pathology. Nature. 2019; doi:10.1038/s41586-019-1769-z |
[163] | Xiong J , Wang C , Chen H , Hu Y , Tian L , Pan J , et al. Aβ-induced microglial cell activation is inhibited by baicalin through the JAK2/STAT3 signaling pathway. Int J Neurosci. (2014) ;124: (8):609–20. doi:10.3109/00207454.2013.865027 |
[164] | Du ZR , Feng XQ , Li N , Qu JX , Feng L , Chen L , et al. G protein-coupled estrogen receptor is involved in the anti-inflammatory effects of genistein in microglia. Phytomedicine. (2018) ;43: :11–20. doi:10.1016/j.phymed.2018.03.039 |
[165] | Zhou LT , Wang KJ , Li L , Li H , Geng M . Pinocembrin inhibits lipopolysaccharide-induced inflammatory mediators production in BV2 microglial cells through suppression of PI3K/Akt/NF-κB pathway. Eur J Pharmacol. (2015) ;761: :211–6. doi:10.1016/j.ejphar.2015.06.003 |
[166] | Cai Y , Kong H , Pan YB , Jiang L , Pan XX , Hu L , et al. Procyanidins alleviates morphine tolerance by inhibiting activation of NLRP3 inflammasome in microglia. J Neuroinflammation. (2016) ;13: (1). doi:10.1186/s12974-016-0520-z |
[167] | Spagnuolo C , Moccia S , Russo GL . Anti-inflammatory effects of flavonoids in neurodegenerative disorders. Eur J Med Chem. (2018) ;153: :105–15. doi:10.1016/j.ejmech.2017.09.001 |
[168] | Cheng X , Yang L , He P , Li R , Shen Y . Differential activation of tumor necrosis factor receptors distinguishes between brains from Alzheimer’s disease and non-demented patients. J Alzheimer’s Dis. (2010) ;19: (2):621–30. doi:10.3233/jad-2010-1253 |
[169] | McAlpine FE , Lee JK , Harms AS , Ruhn KA , Blurton-Jones M , Hong J , et al. Inhibition of soluble TNF signaling in a mouse model of Alzheimer’s disease prevents pre-plaque amyloid-associated neuropathology. Neurobiol Dis. (2009) ;34: (1):163–77. doi:10.1016/j.nbd.2009.01.006 |
[170] | Liaoi YF , Wang BJ , Cheng HT , Kuo LH , Wolfe MS . Tumor necrosis factor-α, interleukin-1β, and interferon-γ stimulate γ-secretase-mediated cleavage of amyloid precursor protein through a JNK-dependent MAPK pathway. J Biol Chem. (2004) ;279: (47):49523–32. doi:10.1074/jbc.m402034200 |
[171] | Rice-Evans CA , Miller NJ , Paganga G . Structure-antioxidant activity relationships of flavonoids and phenolic acids. Free Radic Biol Med. (1996) ;20: (7):933–56. doi:10.1016/0891-5849(95)02227-9 |
[172] | Barone E . Editorial (Thematic Issue: Oxidative Stress and Alzheimer Disease: Where Do We Stand?). Curr Alzheimer Res. (2016) ;13: (2):108–11. doi:10.2174/156720501302160101123849 |
[173] | Youssef P , Chami B , Lim J , Middleton T , Sutherland GT , Witting PK . Evidence supporting oxidative stress in a moderately affected area of the brain in Alzheimer’s disease. Sci Rep. (2018) ;8: (1):11553. doi:10.1038/s41598-018-29770-3 |
[174] | Gsell W , Conrad R , Hickethier M , Sofic E , Frölich L , Wichart I , et al. Decreased Catalase Activity but Unchanged Superoxide Dismutase Activity in Brains of Patients with Dementia of Alzheimer Type. J Neurochem. (2002) ;64: (3):1216–23. doi:10.1046/j.1471-4159.1995.64031216.x |
[175] | Crossthwaite AJ , Hasan S , Williams RJ . Hydrogen peroxide-mediated phosphorylation of ERK1/2, Akt/PKB and JNK in cortical neurones: dependence on Ca2+ and PI3-kinase. J Neurochem. (2002) ;80: (1):24–35. doi:10.1046/j.0022-3042.2001.00637.x |
[176] | Samanta S , Perkinton MS , Morgan M , Williams RJ . Hydrogen Peroxide Enhances Signal-Responsive Arachidonic Acid Release from Neurons: Role of Mitogen-Activated Protein Kinase. J Neurochem. (1998) ;70: (5):2082–90. doi:10.1046/j.1471-4159.1998.70052082.x |
[177] | Halliwell B . Oxidative stress and neurodegeneration: where are we now? J Neurochem. (2006) ;97: (6):1634–58. doi:10.1111/j.1471-4159.2006.03907.x |
[178] | GOODMAN L . Alzheimer’s disease; a clinico-pathologic analysis of twenty-three cases with a theory on pathogenesis. J Nerv Ment Dis. (1953) ;118: (2):97–130. |
[179] | Acosta-Cabronero J , Williams GB , Cardenas-Blanco A , Arnold RJ , Lupson V , Nestor PJ . In vivo quantitative susceptibility mapping (QSM) in Alzheimer’s disease. PLoS One. (2013) ;8: (11). doi:10.1371/journal.pone.0081093 |
[180] | Wang D , Li YY , Luo JH , Li YH . Age-related iron deposition in the basal ganglia of controls and Alzheimer disease patients quantified using susceptibility weighted imaging. Arch Gerontol Geriatr. (2014) ;59: (2):439–49. doi:10.1016/j.archger.2014.04.002 |
[181] | Du L , Zhao Z , Cui A , Zhu Y , Zhang L , Liu J , et al. Increased Iron Deposition on Brain Quantitative Susceptibility Mapping Correlates with Decreased Cognitive Function in Alzheimer’s Disease. ACS Chem Neurosci. (2018) ;9: (7):1849–57. doi:10.1021/acschemneuro.8b00194 |
[182] | Ayton S , Wang Y , Diouf I , Schneider JA , Brockman J , Morris MC , et al. Brain iron is associated with accelerated cognitive decline in people with Alzheimer pathology. Mol Psychiatry. 2019; doi:10.1038/s41380-019-0375-7 |
[183] | Choudhry F , Howlett DR , Richardson JC , Francis PT , Williams RJ . Pro-oxidant diet enhances β/γ secretase-mediated APP processing in APP/PS1 transgenic mice. Neurobiol Aging. (2012) ;33: (5):960–8. doi:10.1016/j.neurobiolaging.2010.07.008 |
[184] | Reiber H , Ruff M , Uhr M . Ascorbate concentration in human cerebrospinal fluid (CSF) and serum. Intrathecal accumulation and CSF flow rate. Clin Chim Acta. (1993) ;217: (2):163–73. doi:10.1016/0009-8981(93)90162-w |
[185] | Borges G , Ottaviani JI , van der Hooft JJJ , Schroeter H , Crozier A . Absorption, metabolism, distribution and excretion of (–)-epicatechin: A review of recent findings. Mol Aspects Med. (2018) ;61: :18–30. doi:10.1016/j.mam.2017.11.002 |
[186] | Schroeter H , Williams RJ , Matin R , Iversen L , Rice-Evans CA . Phenolic antioxidants attenuate neuronal cell death following uptake of oxidized low-density lipoprotein. Free Radic Biol Med. (2000) ;29: (12):1222–33. doi:10.1016/s0891-5849(00)00415-9 |
[187] | Thimmulappa RK , Mai KH , Srisuma S , Kensler TW , Yamamoto M , Biswal S . Identification of Nrf2-regulated genes induced by the chemopreventive agent sulforaphane by oligonucleotide microarray. Cancer Res. (2002) ;62: (18):5196–203. |
[188] | Pallauf K , Duckstein N , Hasler M , Klotz LO , Rimbach G . Flavonoids as Putative Inducers of the Transcription Factors Nrf2, FoxO, and PPAR γ. Oxid Med Cell Longev. 2017;2017. doi:10.1155/2017/4397340 |
[189] | Paredes-Gonzalez X , Fuentes F , Jeffery S , Saw CLL , Shu L , Su ZY , et al. Induction of NRF2-mediated gene expression by dietary phytochemical flavones apigenin and luteolin. Biopharm Drug Dispos. (2015) ;36: (7):440–51. doi:10.1002/bdd.1956 |
[190] | Saw CLL , Guo Y , Yang AY , Paredes-Gonzalez X , Ramirez C , Pung D , et al. The berry constituents quercetin, kaempferol, and pterostilbene synergistically attenuate reactive oxygen species: Involvement of the Nrf2-ARE signaling pathway. Food Chem Toxicol. (2014) ;72: :303–11. doi:10.1016/j.fct.2014.07.038 |
[191] | Bahia PK , Rattray M , Williams RJ . The dietary flavonoid (-)epicatechin stimulates phosphatidylinositol 3-kinase dependent antioxidant response element activity and upregulates glutathione in cortical astrocytes. J Neurochem. (2008) ;106: (5):2194–204. doi:10.1111/j.1471-4159.2008.05542.x |
[192] | Shih AY , Johnson DA , Wong G , Kraft AD , Jiang L , Erb H , et al. Coordinate regulation of glutathione biosynthesis and release by Nrf2-expressing glia potently protects neurons from oxidative stress. J Neurosci. (2003) ;23: (8):3394–406. |
[193] | Arredondo F , Echeverry C , Abin-Carriquiry JA , Blasina F , Antúnez K , Jones DP , et al. After cellular internalization, quercetin causes Nrf2 nuclear translocation, increases glutathione levels, and prevents neuronal death against an oxidative insult. Free Radic Biol Med. (2010) ;49: (5):738–47. doi:10.1016/j.freeradbiomed.2010.05.020 |
[194] | Romeo L , Intrieri M , D’Agata V , Ontario ML , Oriani G , Mangano NG , et al. The major green tea polyphenol, (-)-epigallocatechin-3-gallate, induces heme oxygenase in rat neurons and acts as an effective neuroprotective agent against oxidative stress. J Am Coll Nutr. (2009) ;28: :492S–499S. doi:10.1080/07315724.2009.10718116 |
[195] | Phillips HS , Hains JM , Armanini M , Laramee GR , Johnson SA , Winslow JW . BDNF mRNA Is Decreased in the Hippocampus of Individuals with Alzheimer’s Disease. Vol. 7, Neuron. 1991. |
[196] | Murray KD , Gall CM , Jones EG , Isackson PJ . Differential regulation of brain-derived neurotrophic factor and type II calcium/calmodulin-dependent protein kinase messenger RNA expression in Alzheimer’s disease. Neuroscience. (1994) ;60: (1):37–48. doi:10.1016/0306-4522(94)90202-x |
[197] | Connor B , Young D , Yan Q , Faull RLM , Synek B , Dragunow M . Brain-derived neurotrophic factor is reduced in Alzheimer’s disease. Mol Brain Res. (1997) ;49: (1-2):71–81. doi:10.1016/s0169-328x(97)00125-3 |
[198] | Wang Z-H , Xiang J , Liu X , Yu SP , Manfredsson FP , Sandoval IM , et al. Deficiency in BDNF/TrkB Neurotrophic Activity Stimulates δ-Secretase by Upregulating C/EBPβ in Alzheimer’s Disease. Cell Rep. (2019) ;28: (3):655–669e5. doi:10.1016/j.celrep.2019.06.054 |
[199] | Xiang J , Wang Z-H , Ahn EH , Liu X , Yu S-P , Manfredsson FP , et al. Delta-secretase-cleaved Tau antagonizes TrkB neurotrophic signalings, mediating Alzheimer’s disease pathologies. Proc Natl Acad Sci U S A. (2019) ;116: (18):9094–102. doi:10.1073/pnas.1901348116 |
[200] | Moreno-Jiménez EP , Flor-García M , Terreros-Roncal J , Rábano A , Cafini F , Pallas-Bazarra N , et al. Adult hippocampal neurogenesis is abundant in neurologically healthy subjects and drops sharply in patients with Alzheimer’s disease. Nat Med. (2019) ;25: (4):554–60. doi:10.1038/s41591-019-0375-9 |
[201] | Tobin MK , Musaraca K , Disouky A , Bennett DA , Arfanakis K , Lazarov Correspondence O . Human Hippocampal Neurogenesis Persists in Aged Adults and Alzheimer’s Disease Patients Higher numbers of DCX + PCNA + cells correlate with higher cognitive scores d Increased DCX + PCNA + cells correlate with levels of interaction of presynaptic SNAREs. Stem Cell. 2019; doi:10.1016/j.stem.2019.05.003 |
[202] | Schroeter H , Bahia P , Spencer JPE , Sheppard O , Rattray M , Cadenas E , et al. (-)Epicatechin stimulates ERK-dependent cyclic AMP response element activity and up-regulates GluR2 in cortical neurons. J Neurochem. (2007) ;101: (6):1596–606. doi:10.1111/j.1471-4159.2006.04434.x |
[203] | Lv M , Yang S , Cai L , Qin L , Li B , Wan Z . Effects of Quercetin Intervention on Cognition Function in APP/PS1 Mice was Affected by Vitamin D Status. Mol Nutr Food Res. (2018) ;62: (24):1800621. doi:10.1002/mnfr.201800621 |
[204] | Wang D , Li S , Chen J , Liu L , Zhu X . The Effects of Astilbin on Cognitive Impairments in a Transgenic Mouse Model of Alzheimer’s Disease. Cell Mol Neurobiol. (2017) ;37: (4):695–706. doi:10.1007/s10571-016-0405-9 |
[205] | Karimipour M , Rahbarghazi R , Tayefi H , Shimia M , Ghanadian M , Mahmoudi J , et al. Quercetin promotes learning and memory performance concomitantly with neural stem/progenitor cell proliferation and neurogenesis in the adult rat dentate gyrus. Int J Dev Neurosci. (2019) ;74: :18–26. doi:10.1016/j.ijdevneu.2019.02.005 |
[206] | Czank C , Cassidy A , Zhang Q , Morrison DJ , Preston T , Kroon PA , et al. Human metabolism and elimination of the anthocyanin, cyanidin-3-glucoside: a 13C-tracer study. Am J Clin Nutr. (2013) ;97: (5):995–1003. doi:10.3945/ajcn.112.049247 |
[207] | Borges G , van der Hooft JJJ , Crozier A . A comprehensive evaluation of the [2-14C](–)-epicatechin metabolome in rats. Free Radic Biol Med. (2016) ;99: :128–38. doi:10.1016/j.freeradbiomed.2016.08.001 |
[208] | Ottaviani JI , Borges G , Momma TY , Spencer JPE , Keen CL , Crozier A , et al. The metabolome of [2-(14)C](-)-epicatechin in humans: implications for the assessment of efficacy, safety, and mechanisms of action of polyphenolic bioactives. Sci Rep. (2016) ;6: :29034. doi:10.1038/srep29034 |
[209] | Wang J , Ferruzzi MG , Ho L , Blount J , Janle EM , Gong B , et al. Brain-Targeted Proanthocyanidin Metabolites for Alzheimer’s Disease Treatment. J Neurosci. (2012) ;32: (15):5144–50. doi:10.1523/jneurosci.6437-11.2012 |
[210] | Felgines C , Krisa S , Mauray A , Besson C , Lamaison JL , Scalbert A , et al. Radiolabelled cyanidin 3-O-glucoside is poorly absorbed in the mouse. Br J Nutr. (2010) ;103: (12):1738–45. doi:10.1017/s0007114510000061 |
[211] | Yeh SL , Lin YC , Lin YL , Li CC , Chuang CH . Comparing the metabolism of quercetin in rats, mice and gerbils. Eur J Nutr. (2016) ;55: (1):413–22. doi:10.1007/s00394-015-0862-9 |
[212] | Ottaviani JI , Fong R , Kimball J , Ensunsa JL , Gray N , Vogiatzoglou A , et al. Evaluation of (–)-epicatechin metabolites as recovery biomarker of dietary flavan-3-ol intake. Sci Rep. (2019) ;9: (1). doi:10.1038/s41598-019-49702-z |
[213] | Wang D , Ho L , Faith J , Ono K , Janle EM , Lachcik PJ , et al. Role of intestinal microbiota in the generation of polyphenol-derived phenolic acid mediated attenuation of Alzheimer’s disease β-amyloid oligomerization. Mol Nutr Food Res. (2015) ;59: (6):1025–40. doi:10.1002/mnfr.201400544 |
[214] | Ho L , Zhao D , Ono K , Ruan K , Mogno I , Tsuji M , et al. Heterogeneity in gut microbiota drive polyphenol metabolism that influences α-synuclein misfolding and toxicity. J Nutr Biochem. (2019) ;64: :170–81. doi:10.1016/j.jnutbio.2018.10.019 |
[215] | Murota K , Nakamura Y , Uehara M . Flavonoid metabolism: The interaction of metabolites and gut microbiota. Vol. 82, Bioscience, Biotechnology and Biochemistry. Japan Society for Bioscience Biotechnology and Agrochemistry; 2018. p. 600-10. doi:10.1080/09168451.2018.1444467 |
[216] | Hidalgo M , Jose Oruna-Concha M , Kolida S , Walton GE , Kallithraka S , Spencer JPE , et al. Metabolism of Anthocyanins by Human Gut Microflora and Their Influence on Gut Bacterial Growth. 2012; doi:10.1021/jf3002153 |
[217] | Erlund I , Kosonen T , Alfthan G , Mäenpää J , Perttunen K , Kenraali J , et al. Pharmacokinetics of quercetin from quercetin aglycone and rutin in healthy volunteers. Eur J Clin Pharmacol. (2000) ;56: (8):545–53. doi:10.1007/s002280000197 |
[218] | Cao G , Muccitelli HU , Sánchez-Moreno C , Prior RL . Anthocyanins are absorbed in glycated forms in elderly women: A pharmacokinetic study. Am J Clin Nutr. (2001) ;73: (5):920–6. doi:10.1093/ajcn/73.5.920 |
[219] | Unno K , Pervin M , Nakagawa A , Iguchi K , Hara A , Takagaki A , et al. Blood–Brain Barrier Permeability of Green Tea Catechin Metabolites and their Neuritogenic Activity in Human Neuroblastoma SH-SY5Y Cells. Mol Nutr Food Res. (2017) ;61: (12):1700294. doi:10.1002/mnfr.201700294 |
[220] | Ruotolo R , Minato I , La Vitola P , Artioli L , Curti C , Franceschi V , et al. Flavonoid-Derived Human Phenyl-γ-Valerolactone Metabolites Selectively Detoxify Amyloid-β Oligomers and Prevent Memory Impairment in a Mouse Model of Alzheimer’s Disease. Mol Nutr Food Res. (2020) ;64: (5):1900890. doi:10.1002/mnfr.201900890 |
[221] | Esteban-Fernández A , Rendeiro C , Spencer JPE , Del Coso DG , de Llano MDG , Bartolomé B , et al. Neuroprotective Effects of Selected Microbial-Derived Phenolic Metabolites and Aroma Compounds from Wine in Human SH-SY5Y Neuroblastoma Cells and Their Putative Mechanisms of Action. Front Nutr. (2017) ;4: :3. doi:10.3389/fnut.2017.00003 |
[222] | González-Sarrías A , Núñ ez-Sánchez MÁ , Tomás-Barberán FA , Espín JC . Neuroprotective Effects of Bioavailable Polyphenol-Derived Metabolites against Oxidative Stress-Induced Cytotoxicity in Human Neuroblastoma SH-SY5Y Cells. J Agric Food Chem. (2017) ;65: (4):752–8. doi:10.1021/acs.jafc.6b04538 |
[223] | Ivey KL , Chan AT , Izard J , Cassidy A , Rogers GB , Rimm EB . Role of Dietary Flavonoid Compounds in Driving Patterns of Microbial Community Assembly. MBio. (2019) ;10: (5). doi:10.1128/mbio.01205-19 |
[224] | Cattaneo A , Cattane N , Galluzzi S , Provasi S , Lopizzo N , Festari C , et al. Association of brain amyloidosis with pro-inflammatory gut bacterial taxa and peripheral inflammation markers in cognitively impaired elderly. Neurobiol Aging. (2017) ;49: :60–8. doi:10.1016/j.neurobiolaging.2016.08.019 |
[225] | Vogt NM , Kerby RL , Dill-McFarland KA , Harding SJ , Merluzzi AP , Johnson SC , et al. Gut microbiome alterations in Alzheimer’s disease. Sci Rep. (2017) ;7: (1). doi:10.1038/s41598-017-13601-y |
[226] | Wang X , Sun G , Feng T , Zhang J , Huang X , Wang T , et al. Sodium oligomannate therapeutically remodels gut microbiota and suppresses gut bacterial amino acids-shaped neuroinflammation to inhibit Alzheimer’s disease progression. Cell Res. (2019) ;29: (10):787–803. doi:10.1038/s41422-019-0216-x |
[227] | Dodiya HB , Kuntz T , Shaik SM , Baufeld C , Leibowitz J , Zhang X , et al. Sex-specific effects of microbiome perturbations on cerebral Ab amyloidosis and microglia phenotypes. J Exp Med. (2019) ;216: (7):1542–60. doi:10.1084/jem.20182386 |
[228] | Kim YS , Unno T , Kim BY , Park MS . Sex differences in gut microbiota. Vol. 38, World Journal of Men?s Health. Korean Society for Sexual Medicine and Andrology; 2020. p. 48-60. doi:10.5534/wjmh.190009 |
[229] | Carry E , Zhao D , Mogno I , Faith J , Ho L , Villani T , et al. Targeted analysis of microbial-generated phenolic acid metabolites derived from grape flavanols by gas chromatography-triple quadrupole mass spectrometry. J Pharm Biomed Anal. (2018) ;159: :374–83. doi:10.1016/j.jpba.2018.06.034 |
[230] | Tamura M , Hoshi C , Kobori M , Takahashi S , Tomita J , Nishimura M , et al. Quercetin metabolism by fecal microbiota from healthy elderly human subjects. PLoS One. (2017) ;12: (11). doi:10.1371/journal.pone.0188271 |
[231] | Novotny JA , Chen TY , Terekhov AI , Gebauer SK , Baer DJ , Ho L , et al. The effect of obesity and repeated exposure on pharmacokinetic response to grape polyphenols in humans. Mol Nutr Food Res. (2017) ;61: (11). doi:10.1002/mnfr.201700043 |
[232] | Chen TY , Ferruzzi MG , Wu QL , Simon JE , Talcott ST , Wang J , et al. Influence of diabetes on plasma pharmacokinetics and brain bioavailability of grape polyphenols and their phase II metabolites in the Zucker diabetic fatty rat. Mol Nutr Food Res. (2017) ;61: (10). doi:10.1002/mnfr.201700111 |
[233] | Faria A , Mateus N , Calhau C . Flavonoid transport across blood-brain barrier: Implication for their direct neuroprotective actions. Nutr Aging. (2012) ;1: (2):89–97. doi:10.3233/nua-2012-0005 |
[234] | Patra JK , Das G , Fraceto LF , Campos EVR , Rodriguez-Torres M del P , Acosta-Torres LS , et al. Nano based drug delivery systems: recent developments and future prospects. J Nanobiotechnology. (2018) ;16: (1):71. doi:10.1186/s12951-018-0392-8 |
[235] | Smith A , Giunta B , Bickford PC , Fountain M , Tan J , Shytle RD . Nanolipidic particles improve the bioavailability and α-secretase inducing ability of epigallocatechin-3-gallate (EGCG) for the treatment of Alzheimer’s disease. Int J Pharm. (2010) ;389: (1-2):207–12. doi:10.1016/j.ijpharm.2010.01.012 |
[236] | Moreno LCG e I , Puerta E , Suárez-Santiago JE , Santos-Magalhães NS , Ramirez MJ , Irache JM . Effect of the oral administration of nanoencapsulated quercetin on a mouse model of Alzheimer’s disease. Int J Pharm. (2017) ;517: (1-2):50–7. doi:10.1016/j.ijpharm.2016.11.061 |
[237] | Jalili-Baleh L , Babaei E , Abdpour S , Nasir Abbas Bukhari S , Foroumadi A , Ramazani A , et al. A review on flavonoid-based scaffolds as multi-target-directed ligands (MTDLs) for Alzheimer’s disease. Vol. 152, European Journal of Medicinal Chemistry. Elsevier Masson SAS; 2018. p. 570-89. doi:10.1016/j.ejmech.2018.05.004 |
[238] | Jin X , Liu M , Zhang D , Zhong X , Du K , Qian P , et al. Baicalin mitigates cognitive impairment and protects neurons from microglia-mediated neuroinflammation via suppressing NLRP 3 inflammasomes and TLR 4/ NF -κB signaling pathway. CNS Neurosci Ther. (2019) ;25: (5):575–90. doi:10.1111/cns.13086 |
[239] | Catuara-Solarz S , Espinosa-Carrasco J , Erb I , Langohr K , Notredame C , Gonzalez JR , et al. Principal Component Analysis of the Effects of Environmental Enrichment and (-)-epigallocatechin-3-gallate on Age-Associated Learning Deficits in a Mouse Model of Down Syndrome. Front Behav Neurosci. (2015) ;9: :330. doi:10.3389/fnbeh.2015.00330 |