Simultaneous assessment of blood coagulation and hematocrit levels in dielectric blood coagulometry
Abstract
Background:
In a whole blood coagulation test, the concentration of any in vitro diagnostic agent in plasma is dependent on the hematocrit level but its impact on the test result is unknown.
Objective:
The aim of this work was to clarify the effects of reagent concentration, particularly Ca2+, and to find a method for hematocrit estimation compatible with the coagulation test.
Methods:
Whole blood coagulation tests by dielectric blood coagulometry (DBCM) and rotational thromboelastometry were performed with various concentrations of Ca2+ or on samples with different hematocrit levels. DBCM data from a previous clinical study of patients who underwent total knee arthroplasty were re-analyzed.
Results:
Clear Ca2+ concentration and hematocrit level dependences of the characteristic times of blood coagulation were observed. Rouleau formation made hematocrit estimation difficult in DBCM, but use of permittivity at around 3 MHz made it possible. The re-analyzed clinical data showed a good correlation between permittivity at 3 MHz and hematocrit level (
Conclusions:
Changes in the hematocrit level may affect whole blood coagulation tests. DBCM has the potential to overcome this effect with some automated correction using results from simultaneous evaluations of the hematocrit level and blood coagulability.
1.Introduction
Whole blood coagulation tests such as thromboelastography [1], rotational thromboelastometry [2] and recently developed dielectric blood coagulometry (DBCM) [3] are intended for the evaluation of comprehensive blood coagulability. It has also been reported that DBCM is highly sensitive to hypercoagulability in patients with diabetes [4] or respiratory diseases [5], and it is also promising for risk assessment of stroke [6] and prediction of venous thromboembolism after total knee arthroplasty [7]. In DBCM, as well as thromboelastometry, Ca2+ is commonly used to restart the blood coagulation process on citrated blood. Additionally, tissue factor and ellagic acid, which are activators of the extrinsic and intrinsic coagulation pathways, respectively, and other test reagents can be used according to the design of the assays. One of the unsolved issues in whole blood coagulation tests is uncertainty about reagent concentration in plasma that is possibly affected by the hematocrit level. Using a certain amount of a reagent in a test, a sample with a higher hematocrit level induces a higher concentration of the reagent in plasma due to the excluded volume effect of erythrocytes. However, it has been neither mentioned nor well studied how such an effect impacts on whole blood coagulation tests.
In the case of a diluted plasma sample, it has been reported that the coagulation time measured in the activated recalcification test with Celite (an activator of the intrinsic coagulation pathway) was highly dependent on doped calcium concentration [8]. In fact, the coagulation time decreased with increasing final concentration of Ca2+ up to 8.3 mM, and it increased with further increasing Ca2+ concentrations. As a result, a U- or V-shaped change of the coagulation time was observed [8]. In the case of a whole blood sample, however, little has been studied and is known about the effects of Ca2+ concentration, except the study of thromboelastography with a limiting concentration range of Ca2+ up to 1.8 mM [9].
On the other hand, Spiezia et al. reported that reduction of the hematocrit level induced significant changes of maximum clot firmness (MCF), clotting time (CT), and other parameters in rotational thromboelastometry [10]. They considered that the changes in MCF were mostly just apparent in vitro originating from the method of measurement and did not reflect in vivo coagulability. For the changes in CT, the mechanism was discussed in view of a reduced availability of phospholipids and a change in the total amount of clotting factors. Therefore, the question whether the plasma concentration of the reagent affected by the hematocrit level was responsible for the CT changes remained. In either case, this reminds us that the hematocrit level may affect the estimation of plasma fibrinogen in an extrinsically activated test with reagents containing tissue factor and the platelet inhibitor cytochalasin D (FIBTEM assay) of rotational thromboelastometry [11]. Against this background, simultaneous assessments of blood coagulation and hematocrit level are now the key issue to develop an automated alert or correction method.
Simultaneous assessments will be easily accomplished if the hematocrit level can be evaluated before blood coagulation occurs. In that case, the effects of hematocrit level and blood coagulation can be separated and estimated. However, thromboelastography and rotational thromboelastometry do not comply with this requirement because they are not sensitive to the hematocrit level without coagulation occurring. In comparison, dielectric spectroscopy (DS), which is the measurement principle of DBCM, is generally very sensitive to the cell volume fraction (ϕ) of a sample [12,13]. In the most simplified system (not for real blood), such as a diluted suspension of spherical cells, ϕ is described by:
Because whole blood is a highly complex system, the above theories are not applicable as they are. The particular problem is rouleau formation of erythrocytes, which is reversible aggregation occurring in a stationary condition of blood without flow [19]. It is known that chain-shape rouleaux are formed over several seconds, afterward progressing to a three-dimensional network in several dozen seconds [20]. These formations then become homogeneous spherical aggregates, and, at that stage, the first sedimentation starts [20]. Through all these processes,
On the other hand, the main DS response of blood is interfacial polarization on the erythrocyte membrane that manifests itself as β-dispersion in the frequency around hundreds of kHz to the MHz region [3,12,21]. This β-dispersion depends on several parameters, including sample temperature [21], cell shape, and aggregational state [12,17–19,22], as well as the hematocrit level [17,21]. Although estimation of the hematocrit level from β-dispersion requires additional ingenuity, it is worth trying.
In the present work, a study using blood from healthy volunteers was performed first to confirm dose dependences of Ca2+ in DBCM and rotational thromboelastometry. Second, dielectric data obtained in a previous study [3] were re-analyzed to identify a parameter that is sensitive to the hematocrit level but insensitive to rouleau formation. Such a parameter would be useful for estimating hematocrit in whole blood. Third, DBCM data obtained in a previous clinical study on patients who underwent total knee arthroplasty [7] were re-analyzed to compare the dielectric permittivity data and the hematocrit level that were recorded as clinical data. Finally, the effects of the hematocrit level on whole blood coagulation tests are discussed.
2.Materials and methods
2.1.Sample blood
This study was approved by the Ethics Committee of Tokyo Medical and Dental University. Blood samples were drawn into collection tubes with 3.13% sodium citrate solution (9:1 in volume) from healthy volunteers who consented to the study. In a previous study [7], patients scheduled to undergo unilateral or bilateral total knee arthroplasty at the university hospital were considered for enrollment, and written, informed consent was obtained from all enrolled patients. Citrated blood samples were obtained in the morning just before the surgery and a day after it, and DBCM measurements and routine blood tests were carried out. Blood collection was done at the same time, with different collection tubes for DBCM measurement and the routine blood tests. Portions of these data were re-analyzed in the present work.
The clinical part of the present study using data corresponding to the patients was performed in Tokyo Medical and Dental University, and the nonclinical part of the study was carried out at Sony Corporation.
2.2.Ca2+ concentration dependences
The principle of DBCM is summarized elsewhere [3]. Briefly, dielectric measurements were done using an automated blood coagulation analyzer prototype (Sony Corporation, Tokyo, Japan). This apparatus consists of an automated blood dispenser, sample cartridge holders with temperature controller, an impedance analyzer board, and a computer. The system was calibrated by open, short, and load (50 ohm) circuits. An operator set disposable sample cartridges and blood collection tube in the apparatus after inversion mixing of blood, and started measurements. A whole blood sample of 180 µl was dispensed into each cartridge with titanium electrode inserts as a parallel plate type capacitor, which is electrically connected to the impedance analyzer board through electric contact pins and coaxial cables. The dispensed sample was mixed and stirred with the reagent previously pipetted into the cartridge. As reagents, 12 µl of calcium chloride solution with various concentrations from 50 to 400 mM (final concentration in blood from 3 to 25 mM) were tested, where blood samples were obtained from three healthy subjects (ages: 30, 34 and 42 years, respectively). The complex impedance data of the sample at 37°C (errors within 0 and −1°C) were recorded over time up to 60 min with an interval of one min and frequency range from 1 kHz to 10 MHz and converted to complex dielectric permittivity (
The effect of Ca2+ concentration was also tested in a limited number of experiments by rotational thromboelastometry ROTEM® delta (TEM group, Basel, Switzerland), where calcium chloride solutions of 200, 250, and 308 mM (final concentration in blood: 12.5, 15.6 and 19.3 mM, respectively) were used as reagents instead of the original one supplied by the manufacturer. The sample temperature was controlled to be 37°C during measurements by ROTEM® delta.
2.3.Analysis of rouleau formation
Previously observed DS data for citrated whole blood from a healthy subject (age: 44 years) were re-analyzed to investigate the rouleau formation process [3]. In the cited work [3], a change in dielectric permittivity accompanying rouleau formation was observed at 37°C without recalcification, so that coagulation did not occur. Specimen blood dispensed into the sample cartridge was agitated by manual pipetting movements. Measurements started within 5 s after agitation ended, and the dielectric spectrum was recorded over time with a time interval of 3 s up to approximately 10 min. After that, agitation and dielectric measurements were repeated to confirm both repeatability and reversibility.
2.4.Dielectric permittivity and hematocrit level in clinical study
DBCM data at 37°C obtained in the recent clinical study on patients who underwent total knee arthroplasty [7] were re-analyzed to compare dielectric permittivity and the hematocrit level obtained by a routine blood count test. The mean age of the patients was 73.7 years (SD = 7.6, n (preoperative) = 21 and n (postoperative) = 24).
2.5.In vitro hematocrit handling
Blood samples with different hematocrit levels were prepared as follows. Whole blood in collection tubes from a healthy subject (age: 34 years) was centrifuged with a mild condition (300g, 10 min), platelet rich autologous plasma was added or removed, and then it was mixed again. Blood coagulation tests at 37°C were performed by DBCM and ROTEM®, where 200 mM calcium chloride solution was used as a reagent to achieve a final concentration of Ca2+ in blood of 12.5 mM. The hematocrit level and platelet number of the samples were measured by a blood cell counting device, PoCH100i (Sysmex Corp., Kobe, Japan). In general, ethylenediaminetetraacetic acid (EDTA) is used as an anticoagulant for evaluation of hematocrit level using a blood cell counting device, where a blood sample is not diluted by EDTA because it is dry reagent. In the present experiments, however, we measured the hematocrit levels of citrated blood samples prepared for DBCM and ROTEM® tests. Therefore, we corrected the hematocrit levels to take into account the 10% dilution by sodium citrate.
2.6.Repeatability test
The blood sample from a healthy subject (age: 45 years) was measured at 37°C by the prototype system of DBCM seven times to estimate repeatability of the method, where calcium chloride solutions of 200 mM (final concentration in blood: 12.5 mM) was used as a reagent. The hematocrit level was measured by PoCH100i and corrected in the same way described in the previous Section 2.5.
3.Results and discussion
3.1.Effects of reagent concentration in whole blood coagulation tests
A typical dielectric spectrum of human whole blood is shown in Fig. 1(a), where dielectric permittivity
Fig. 1.
A typical dielectric spectrum (dielectric dispersion curve) of whole blood from a healthy volunteer before the blood coagulation process proceeds (a), and the change in normalized dielectric spectra during the progression of blood coagulation (b). The normalization was done with the dielectric dispersion curve at the first time point.
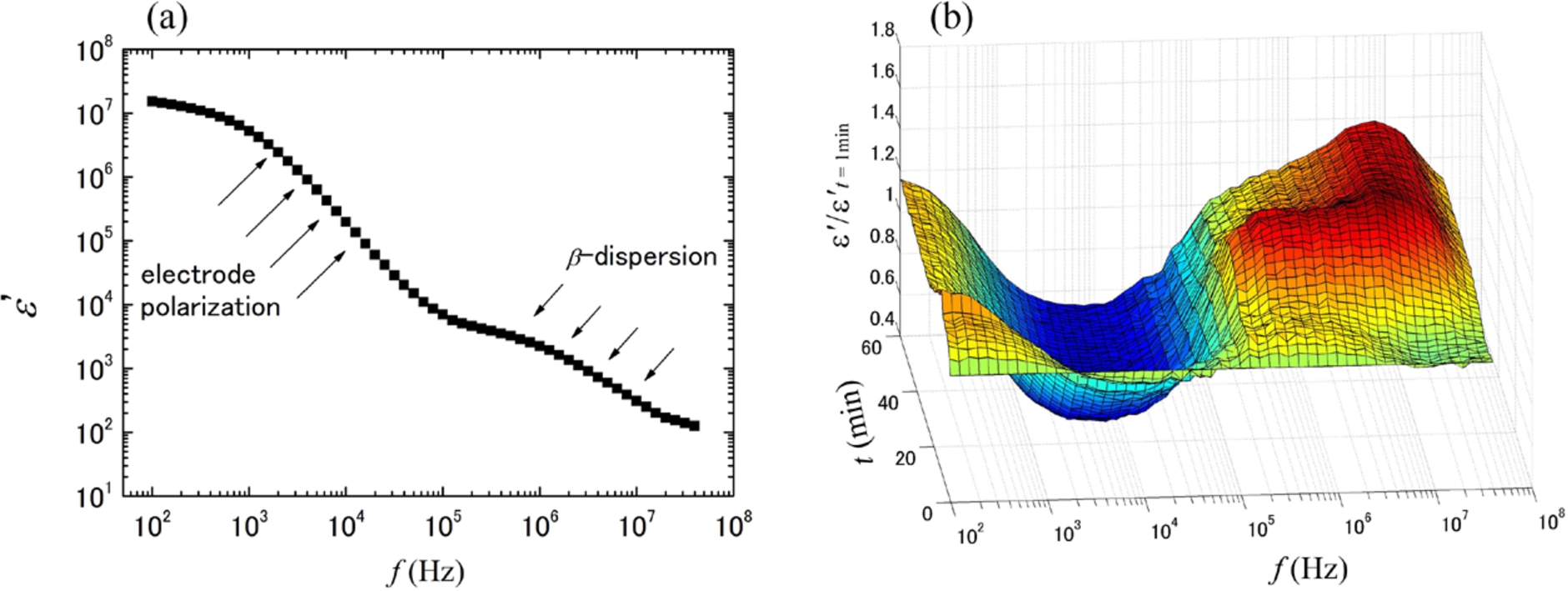
Figure 2 shows permittivity change at 10 MHz through the blood coagulation process that proceeds with arrows to indicate the characteristic time of coagulation (
Fig. 2.
Dielectric permittivity changes at 10 MHz with blood coagulation for the same blood sample with different final concentrations of reagent (Ca2+) at 6.3 mM (black circles), 12.5 mM (blue squares), and 21.9 mM (red triangles). The permittivity data are normalized by the minimum and maximum values of the permittivity, and
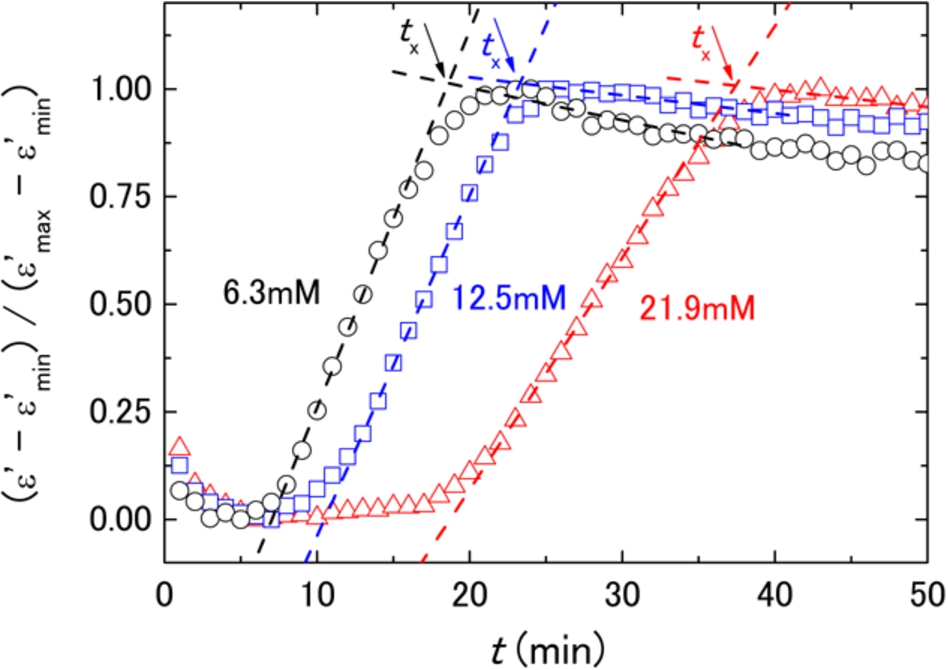
Fig. 3.
Reagent (Ca2+) concentration dependences of
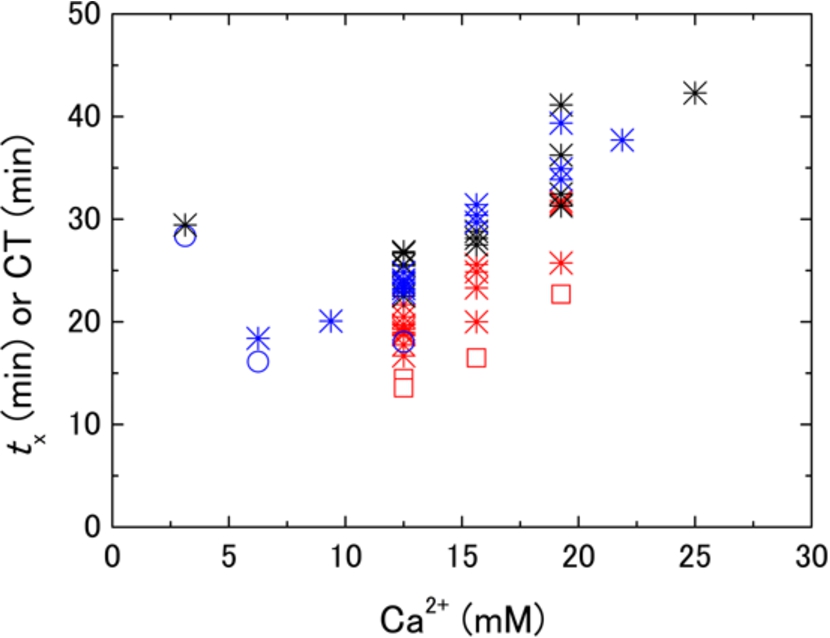
3.2.Rouleau formation and permittivity changes in view of hematocrit estimation
It is known that rouleau formation markedly affects β-dispersion [12,19]. In particular, the relaxation strength increases and the relaxation frequency shifts toward low frequencies, as shown in Fig. 4(a). At the same time, one can see that the permittivity at around 2 to 5 MHz did not change very much with rouleau formation. The effects from the increase of the relaxation strength and the shift of the relaxation frequency canceled out in this frequency region. In fact, the permittivity change by rouleau formation at 3 MHz (Fig. 4(c)) was unnoticeable in comparisons with the cases at 10 MHz (Fig. 4(b)) and 1 MHz (Fig. 4(d)). In contrast, the permittivity at around 2 to 5 MHz did increase with increased hematocrit level in a previously reported study [21]. Therefore, it was expected that the permittivity at a few MHz would be a good indicator of the hematocrit level for human whole blood without the disturbing effects of rouleau formation.
Fig. 4.
Rouleau formation and dielectric response observed in a previous study [3]. Panel (a) shows the dielectric dispersion curves just after pipet mixing and 5 min after. Panels (b), (c), and (d) show changes of the normalized permittivity at 10, 3, and 1 MHz, respectively. The normalization was done with permittivity at the first time point. The measurements of these changes were started just after mixing was stopped and started again after resuspension, three times in a row. The arrows indicate the points at which mixing was stopped. (Rearranged from [3] under the open access license granted by American Chemical Society.)
![Rouleau formation and dielectric response observed in a previous study [3]. Panel (a) shows the dielectric dispersion curves just after pipet mixing and 5 min after. Panels (b), (c), and (d) show changes of the normalized permittivity at 10, 3, and 1 MHz, respectively. The normalization was done with permittivity at the first time point. The measurements of these changes were started just after mixing was stopped and started again after resuspension, three times in a row. The arrows indicate the points at which mixing was stopped. (Rearranged from [3] under the open access license granted by American Chemical Society.)](https://content.iospress.com:443/media/bir/2017/54-1/bir-54-1-bir16118/bir-54-bir16118-g004.jpg)
3.3.Hematocrit level and dielectric response in clinical studies
It was shown in the last Section 3.2 that permittivity at 3 MHz was apparently less influenced by rouleau formation. Therefore, previously obtained data for patients who underwent total knee arthroplasty [7] were re-analyzed to discuss the relevance of permittivity at 3 MHz for hematocrit estimation by comparison with the traditional conductance method.
The relationship between conductivity of a blood sample and the hematocrit level is shown in Fig. 5. As expected, the coefficient of correlation was not high (
Fig. 5.
Conductivity of blood samples (
![Conductivity of blood samples (σs) at 50 kHz observed 1 minute from the start of DBCM measurement against the hematocrit level for patients who underwent total knee arthroplasty [7] (full square). The solid line shows the result of linear regression where the square of the coefficient of correlation (R2) is 0.76. The dashed curve shows the relationship of conductivity and cell volume fraction in Hanai’s theory (Eq. (2)) assuming constant conductivity of the supernatant as σb=1.6 S/m. A plot for a healthy subject is also presented (full diamond) with error bar (SD = 0.065 S/m, CV = 9.8%, n=7) to show the repeatability.](https://content.iospress.com:443/media/bir/2017/54-1/bir-54-1-bir16118/bir-54-bir16118-g005.jpg)
In good contrast with Fig. 5, Fig. 6 shows a better correlation between permittivity at 3 MHz and the hematocrit level (
Fig. 6.
Dielectric permittivity of blood samples at 3 MHz observed 1 minute from the start of DBCM measurement against hematocrit level for patients who underwent total knee arthroplasty [7] (full square). The solid line shows the result of linear regression where the square of the coefficient of correlation (
![Dielectric permittivity of blood samples at 3 MHz observed 1 minute from the start of DBCM measurement against hematocrit level for patients who underwent total knee arthroplasty [7] (full square). The solid line shows the result of linear regression where the square of the coefficient of correlation (R2) is 0.83. A plot for a healthy subject is also presented (full diamond) with error bar (SD = 64.0, CV = 6.8%, n=7) to show the repeatability. Note that there can be systematic errors in permittivity values mainly due to uncertainty of the correction for stray capacitance. In the worst case, for example, the presented permittivity may be underestimated by 5%. Nevertheless, this effect was not critical to the correlation, because always the same systematic errors appeared even in this case.](https://content.iospress.com:443/media/bir/2017/54-1/bir-54-1-bir16118/bir-54-bir16118-g006.jpg)
3.4.Effects of hematocrit level on whole blood coagulation tests
Blood samples with different hematocrit levels prepared by in vitro handling of identical blood were measured by DBCM and ROTEM®. In these experiments, the concentration of the reagent (Ca2+) was constant, and it was 12.5 mM in blood. However, the concentration of the reagent in plasma was dependent on the hematocrit level, for example, it was 25 mM in blood with hematocrit of 50% if the effects of Ca2+ adsorption and uptake by erythrocytes are insignificant. Figure 7 shows prolonging behavior of the characteristic coagulation time,
Fig. 7.
The characteristic coagulation time, such as
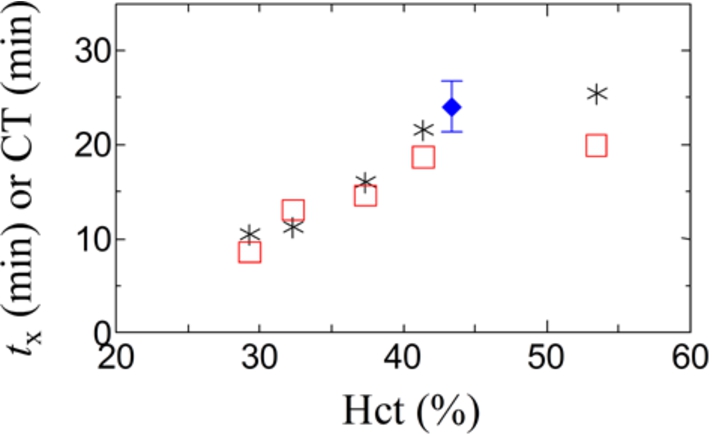
Anderson et al. reported that introduction of thromboelastometry significantly decreased the use of red cells and blood products in cardiac surgery [2]. At the same time, the hematocrit level can be drastically changed during a major surgery. Therefore, the test results may be affected by changes of reagent concentrations in plasma, as shown in the present work.
4.Conclusions
The excluded volume of erythrocytes (i.e., hematocrit level) directly influences plasma concentration of reagents in whole blood coagulation tests. The present work shows that a change in concentration of reagent Ca2+ affected the characteristic coagulation time significantly. Therefore, uncertainty in the Ca2+ concentration may yield an incorrect assessment of blood coagulability. Simultaneous assessments of blood coagulation and hematocrit levels in DBCM will be helpful to solve this problem, because it makes possible a self-contained and automated correction. It is feasible because the dielectric permittivity at around 3 MHz highly correlated to hematocrit level as shown in Fig. 6. To apply this technique to medical applications, however, the accumulation of large-scale data for a wide range of abnormal and healthy subjects is required to define correction coefficients. These will be considered in future studies with a new DBCM system with improved repeatability.
Acknowledgements
This work was partially supported by the Medical Research and Development Programs Focused on Technology Transfer: Development of Advanced Measurement and Analysis Systems (SENTAN) from the Japan Agency for Medical Research and Development, AMED (Y.H).
Conflict of interest
The authors declare the following competing financial interests: Y.H., M.-A.B., K.M., S.L., A.M. and S.O. are employees of Sony Corp. The other authors declare no competing financial interests.
References
[1] | Chitlur M, Sorensen B, Rivard GE, Young G, Ingerslev J, Othman M, et al. Standardization of thromboelastography: A report from the TEG-ROTEM working group. Haemophilia. (2011) ;17: :532–537. doi:10.1111/j.1365-2516.2010.02451.x. |
[2] | Anderson L, Quasim I, Soutar R, Steven M, Macfie A, Korte W. An audit of red cell and blood product use after the institution of thromboelastometry in a cardiac intensive care unit. Transfus Med. (2006) ;16: :31–39. doi:10.1111/j.1365-3148.2006.00645.x. |
[3] | Hayashi Y, Brun M-A, Machida K, Nagasawa M. Principles of dielectric blood coagulometry as a comprehensive coagulation test. Anal Chem. (2015) ;87: :10072–10079. doi:10.1021/acs.analchem.5b02723. |
[4] | Uchimura I, Kaibara M, Nagasawa M, Hayashi Y. Effect of circulating tissue factor on the hypercoagulability in type 2 diabetes mellitus studied by rheometry and dielectric blood coagulometry. Biorheology. (2016) ;53: :209–219. doi:10.3233/BIR-16107. |
[5] | Chiba S, Uchibori K, Fujiwara T, Ogata T, Yamauchi S, Shirai T, et al. Dielectric blood coagulometry as a novel coagulation test. J Sci Res Rep. (2015) ;4: :180–186. |
[6] | Hasegawa Y, Hamada S, Nishimura T, Sasaki T, Ebana Y, Kawabata M, et al. Novel dielectric coagulometer identifies hypercoagulability in patients with a high CHADS2 score without atrial fibrillation. PLoS ONE. (2016) ;11: :e0156557. |
[7] | Uchiyama H, Inoue Y, Uchimura I, Nakamura T, Kudo T, Muneta T, et al. Prediction of venous thromboembolism after total knee arthroplasty using dielectric blood coagulometry. Ann Vasc Surg. (2017) ;38: :286–292. doi:10.1016/j.avsg.2016.05.099. |
[8] | Hunter DT, Allensworth JL. Improved coagulation screening by an activated recalcification test. J Clin Pathol. (1967) ;20: :244–248. doi:10.1136/jcp.20.3.244. |
[9] | James MF, Roche AM. Dose-response relationship between plasma ionized calcium concentration and thrombelastography. J Cardiothorac Vasc Anesth. (2004) ;18: :581–586. doi:10.1053/j.jvca.2004.07.016. |
[10] | Spiezia L, Radu C, Marchioro P, Bertini D, Rossetto V, Castelli M, et al. Peculiar whole blood rotation thromboelastometry (Rotem) profile in 40 sideropenic anaemia patients. Thromb Haemost. (2008) ;100: :1106–1110. |
[11] | Ogawa S, Szlam F, Bolliger D, Nishimura T, Chen EP, Tanaka KA. The impact of hematocrit on fibrin clot formation assessed by rotational thromboelastometry. Anesth Analg. (2012) ;115: :16–21. doi:10.1213/ANE.0b013e31824d523b. |
[12] | Raicu V, Feldman Y. Dielectric relaxation in biological systems: Physical principles, methods, and applications. Oxford: Oxford University Press; (2015) . |
[13] | Hayashi Y, Katsumoto Y, Oshige I, Omori S, Yasuda A, Asami K. The effects of erythrocyte deformability upon hematocrit assessed by the conductance method. Phys Med Biol. (2009) ;54: :2395–2405. doi:10.1088/0031-9155/54/8/009. |
[14] | Takashima S, Asami K, Takahashi Y. Frequency domain studies of impedance characteristics of biological cells using micropipet technique. I. Erythrocyte. Biophys J. (1988) ;54: :995–1000. doi:10.1016/S0006-3495(88)83037-6. |
[15] | Asami K, Hanai T, Koizumi N. Dielectric approach to suspensions of ellipsoidal particles covered with a shell in particular reference to biological cells. Japanese J Appl Phys. (1980) ;19: :359–365. doi:10.1143/JJAP.19.359. |
[16] | Asami K, Yonezawa T. Dielectric behavior of non-spherical cells in culture. Biochim Biophys Acta. (1995) ;1245: :317–324. doi:10.1016/0304-4165(95)00116-6. |
[17] | Hayashi Y, Oshige I, Katsumoto Y, Omori S, Yasuda A, Asami K. Dielectric inspection of erythrocyte morphology. Phys Med Biol. (2008) ;53: :2553–2564. doi:10.1088/0031-9155/53/10/007. |
[18] | Asami K. Characterization of heterogeneous systems by dielectric spectroscopy. Prog Polym Sci. (2002) ;27: :1617–1659. doi:10.1016/S0079-6700(02)00015-1. |
[19] | Irimajiri A, Ando M, Matsuoka R, Ichinowatari T, Takeuchi S. Dielectric monitoring of rouleaux formation in human whole blood: A feasibility study. Biochim Biophys Acta. (1996) ;1290: :207–209. doi:10.1016/0304-4165(96)00048-7. |
[20] | Fabry TL. Mechanism of erythrocyte aggregation and sedimentation. Blood. (1987) ;70: :1572–1576. |
[21] | Wolf M, Gulich R, Lunkenheimer P, Loidl A. Broadband dielectric spectroscopy on human blood. Biochim Biophys Acta. (2011) ;1810: :727–740. doi:10.1016/j.bbagen.2011.05.012. |
[22] | Hayashi Y, Katsumoto Y, Omori S, Yasuda A, Asami K, Kaibara M, et al. Dielectric coagulometry: A new approach to estimate venous thrombosis risk. Anal Chem. (2010) ;82: :9769–9774. doi:10.1021/ac101927n. |
[23] | Asami K, Irimajiri A, Hanai T, Shiraishi N, Utsumi K. Dielectric analysis of mitochondria isolated from rat liver 1. swollen mitoplasts as simulated by a single-shell model. Biochim Biophys Acta. (1984) ;778: :559–569. doi:10.1016/0005-2736(84)90407-3. |
[24] | Feldman Y, Ermolina I, Hayashi Y. Time domain dielectric spectroscopy study of biological systems. IEEE Trns Dielectr Electr Insul. (2003) ;10: :728–753. doi:10.1109/TDEI.2003.1237324. |
[25] | Peratta C, Peratta A. Modelling the human body exposure to elf electric fields. vol. 47: . Southampton: WIT Press; (2010) . |