Paired associative stimulation modulates corticomotor excitability in chronic stroke: A preliminary investigation
Abstract
Background:
Paired associative stimulation (PAS) combining repeated pairing of electrical stimulation of a peripheral nerve with transcranial magnetic stimulation (TMS) over the primary motor cortex (M1) can induce neuroplastic adaptations in the human brain and enhance motor learning in neurologically-intact individuals. However, the extent to which PAS is an effective technique for inducing associative plasticity and improving motor function in individuals post-stroke is unclear.
Objective:
The objective of this pilot study was to investigate the effects of a single session of PAS to modulate corticomotor excitability and motor skill performance in individuals post-stroke.
Methods:
Seven individuals with chronic stroke completed two separate visits separated by at least one week. We assessed general corticomotor excitability, intracortical network activity and behavioral outcomes prior to and at three time points following PAS and compared these outcomes to those following a sham PAS condition (PASSHAM).
Results:
Following PAS, we found increased general corticomotor excitability but no significant difference in behavioral measures between PAS conditions. There was a relationship between PAS-induced corticomotor excitability increase and enhanced motor skill performance across post-PAS testing time points.
Conclusion:
These results provide preliminary evidence for the potential of PAS to increase corticomotor excitability that could favorably impact motor skill performance in chronic individuals post-stroke and are an important first step for future studies investigating the clinical application and behavioral relevance of PAS interventions in post stroke patient populations.
1Introduction
Despite intensive rehabilitation efforts, the majority of individuals post-stroke display persistent functional disability of the paretic arm and hand. These motor impairments are, in part, a result of maladaptive changes in the strength and organization of structural and functional connections between brain regions (Takatsuru et al., 2009; Taub et al., 1994). Intracortical network interactions between afferent pathways to the somatosensory cortex and efferent pathways within the motor cortex typically result in a balanced pattern of cortical excitation and inhibition that is necessary for motor control in neurologically-intact individuals (Roy & Gorassini, 2008). Compromised somatosensory cortical function following stroke may disrupt intracortical interactions between the primary somatosensory (S1) and primary motor cortex (M1) leading to impairments in motor function in individuals post-stroke (Borich, Brodie, Gray, Ionta, & Boyd, 2015). Therapeutic interventions that effectively target sensorimotor pathways could potentially enhance motor recovery in individuals living with post-stroke motor disability.
Neuromodulatory techniques using non-invasive brain stimulation (NIBS) have previously targeted somatosensory networks and motor pathways in isolation, but few studies have implemented NIBS strategies to specifically target pathways of sensorimotor integration. Repetitive transcranial magnetic stimulation (rTMS) and transcranial electrical stimulation (TES) are NIBS approaches that can modulate abnormal brain activity after stroke. However, these techniques have shown limited effectiveness in significantly improving motor function in stroke despite substantial scientific inquiry (Elsner B, Kugler J, & Pohl M, 2016; Hao, Wang, Zeng, & Liu, 2013). One important reason may be that rTMS targets a single cortical region rather than targeting associative plasticity mechanisms between cortical regions known to be important for motor function. Modification of synaptic responsiveness at a network level can be induced through associative neuroplasticity mechanisms with paired associative stimulation (PAS). In neurologically-intact individuals, traditional PAS approaches can increase or decrease M1 cortical excitability by repeatedly associating electrical stimulation of a peripheral nerve with a TMS pulse delivered over the contralateral M1 depending on the specific interstimulus interval (Stefan, Kunesch, Cohen, Benecke, & Classen, 2000). Based on the concept of spike-timing dependent plasticity (STDP), PAS is hypothesized to induce long-term potentiation (LTP)-like plasticity represented by changes in corticomotor excitability when stimulus pairs are repeatedly delivered at an interstimulus interval (ISI: ∼20–25 ms) that maximizes coincident timing of stimuli in the targeted cortical regions (Carson & Kennedy, 2013; Muller-Dahlhaus, Orekhov, Liu, & Ziemann, 2008). In neurologically-intact individuals, facilitatory PAS techniques can increase corticomotor excitability (Carson & Kennedy, 2013; Castel-Lacanal, Gerdelat-Mas, Marque, Loubinoux, & Simonetta-Moreau, 2007; Stefan, Kunesch, Benecke, Cohen, & Classen, 2002; Stefan, Wycislo, & Classen, 2004) and improve motor task performance (Player, Taylor, Alonzo, & Loo, 2012). However, there are no studies to date that have investigated the neurophysiologic or behavioral effects of a traditional facilitatory PAS technique in individuals post-stroke. Using a modified PAS paradigm, Castel-Lacanal et al. found increased measures of general corticomotor excitability to wrist extensor muscles in both a group of two (Castel-Lacanal et al., 2007) and six individuals post-stroke (Castel-Lacanal et al., 2009). Another study pairing peripheral nerve stimulation with transcranial direct current stimulation (tDCS) directed towards motor region of the ipsilesional hemisphere observed improvements in performance accuracy on a keyboard-based skilled motor task in individuals post-stroke (Celnik, Paik, Vandermeeren, Dimyan, & Cohen, 2009). Despite the potential importance to clinical translation, there is a paucity of studies investigating associations between PAS-induced changes in corticomotor excitability and motor behavior. Additionally, the extent to which traditional PAS approaches that can effectively enhance corticomotor excitability in young neurologically-intact individuals may also induce similar neuroplastic changes in individuals after stroke is unknown. Understanding the extent to which PAS can induce positive neuroplastic and motor behavioral changes in stroke patient populations is an important prerequisite to evaluate clinical translational potential.
Previous research has attempted to leverage our understanding of the neural manifestations underpinning paretic arm and hand motor impairment into the development of stroke rehabilitation techniques to improve functional outcomes. Following stroke, there is an observed decrease in ipsilesional M1 corticomotor excitability that results from both decreased facilitatory activity and increased inhibitory activity in GABA-mediated circuits (Ward & Cohen, 2004). This abnormal corticomotor excitability has been associated with the level of post-stroke motor dysfunction (Koski, Mernar, & Dobkin, 2004; Manganotti et al., 2002; Traversa, Cicinelli, Pasqualetti, Filippi, & Rossini, 1998) and increases in ipsilesional corticomotor excitability have been shown to parallel improvements in paretic limb motor function over the course of recovery (Rapisarda, Bastings, de Noordhout, Pennisi, & Delwaide, 1996) and following rehabilitation. (Koski et al., 2004). Thus, stroke rehabilitation approaches that effectively enhance corticomotor excitability within the ipsilesional hemisphere could potentially improve paretic motor function in individuals post-stroke. Given that traditional facilitatory PAS techniques can increase corticomotor excitability in the targeted hemisphere and improve motor task performance in neurologically-intact individuals, it is then conceivable that facilitatory PAS could modulate abnormal activity in intracortical circuits contributing to reduced ipsilesional corticomotor excitability in individuals post-stroke (Carson & Kennedy, 2013; Humeau, Shaban, Bissiere, & Luthi, 2003; Kujirai, Kujirai, Sinkjaer, & Rothwell, 2006; Player et al., 2012). Still, the underlying intracortical mechanisms by which PAS induces changes in general corticomotor excitability measures remain speculative even in neurologically-intact individuals amidst inconsistent findings (Carson & Kennedy, 2013). Some studies have shown changes in short interval cortical inhibition (SICI) and intracortical facilitation (ICF) following traditional PAS (Kujirai et al., 2006) while other studies showed no change in measures reflecting the activity of intracortical networks (Russmann, Lamy, Shamim, Meunier, & Hallett, 2009; Sale, Ridding, & Nordstrom, 2007; Stefan et al., 2002). Considering the atypical cortical network activity observed in the brain after stroke, PAS may well have a different mechanistic corticomotor and behavioral effect in a stroke survivor when compared to a neurologically-intact individual. However, the underlying intracortical mechanisms of PAS-induced neuroplastic and behavioral changes in individuals post-stroke have not been previously investigated.
Currently, there is a limited understanding of PAS-induced neuroplastic and motor behavioral changes in individuals post-stroke. The primary purpose of this study was to determine the capacity for PAS to induce short-term changes in ipsilesional corticomotor excitability in individuals post-stroke. Secondarily, we aimed to investigate the intracortical neural networks underlying PAS-induced changes in corticomotor excitability and explore the behavioral effects of PAS on motor skill performance.
2Methods
A single-group repeated measures crossover experimental study design was used (Fig. 1). Seven individuals with chronic stroke (>6 mo.) (age: 67±11 years, 3 males, Upper Extremity Fugl-Meyer Assessment score: 53±12) were recruited (Table 1). Participants were included if they were between the ages of 21 to 85, had a single subcortical ischemic stroke and a Fugl-Meyer score of >30. Participants were excluded if they had a hemorrhagic stroke due to increased risk for seizure (Kilpatrick et al., 1990; Sung & Chu, 1989), history of multiple clinically-significant strokes, a neurodegenerative disorder or psychiatric diagnosis, or had any contraindications to TMS (Rossini et al., 2015). All participants gave written informed consent and the experimental protocol was approved by the Emory University Institutional Review Board.
Fig.1
Experimental paradigm. During the PAS protocol 180 pulse pairs using an interstimulus interval of N20+5 ms at 0.25 Hz were delivered over the median nerve and primary motor cortex. Measures of corticomotor excitability (1 mV, SICI, ICF, SAI) and motor skill performance (SRTT) were assessed at baseline, immediately following PAS (POST 0) and at 30 and 60 minutes following PAS (POST30 an POST60). (SICI: short interval cortical inhibition, ICF: intracortical facilitation, SAI: short afferent inhibition, SRTT: serial reaction time task).
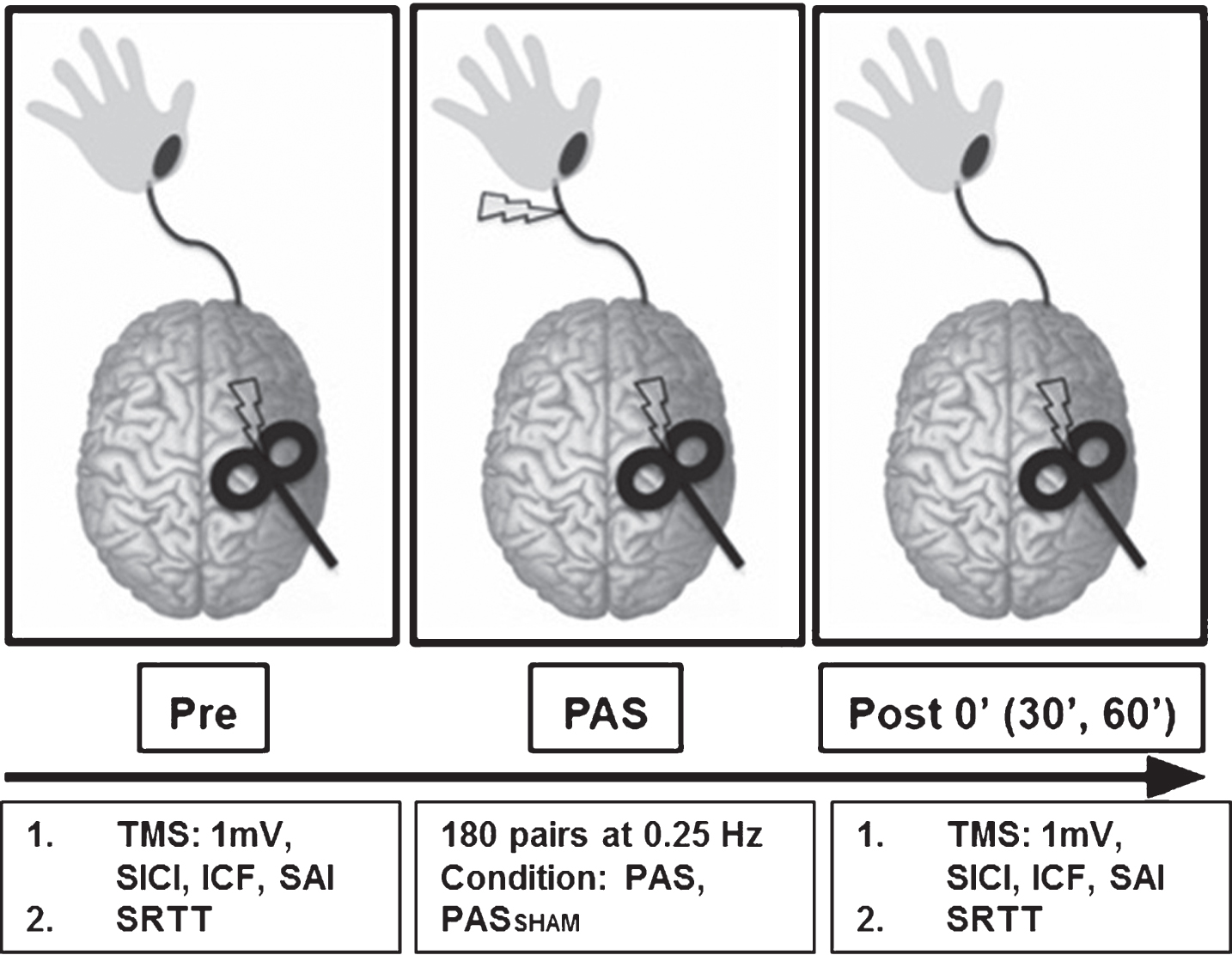
Table 1
Participant characteristics. Mean values±1SD are shown. FM: Fugl-Meyer Upper Extremity Assessment; PSD: Post-stroke Duration (in months); RMT: Resting motor threshold
ID | Age (y)/ gender | CVA location | PSD (mo) | RMT (iM1) PAS | RMT (iM1) sham | FM score |
S02 | 46/F | L BG/IC/TL | 30 | 50 | 49 | 62 |
S03 | 64/F | R ACA | 42 | 63 | 58 | 58 |
S04 | 62/F | L IC | 145 | 68 | 73 | 59 |
S06 | 80/M | R MCA | 35 | 45 | 46 | 35 |
S08 | 75/M | R Thalamus, Cerebral peduncle, BG/IC | 12 | 64 | 63 | 54 |
S09 | 74/F | R Corona radiata, caudate, putamen | 13 | 35 | 38 | 40 |
S10 | 66/M | L BG | 133 | 53 | 55 | 66 |
2.1Assessment of motor behavior
A modified version of the serial reaction time task (SRTT) (Cohen, Pascual-Leone, Press, & Robertson, 2005) was used to evaluate motor skill performance. Briefly, participants were seated in front of a keyboard and a computer screen that displayed a row of white rectangular targets corresponding to each of four sequential keys on the keyboard. Participants were asked to press the key corresponding to the stimulus target as quickly and accurately as possible. The next target would be presented 400 ms following each correct key press. Participants completed a total of 280 key presses during each assessment. During the first block of 50 key presses, the key stimulus sequence was displayed in random order. During the next 15 blocks, a 12-element repeated sequence was presented in each block without participant awareness to evaluate implicit sequence skill performance. During the last block, 50 key presses were presented again in a random order. The duration of each assessment was dependent on participant response time (RT) and each SRTT assessment lasted approximately 3–5 minutes. To evaluate basal motor performance, only the mean RT across the first block of 50 key presses was analyzed in this study. Participants performed the SRTT for the paretic and nonparetic limb in random order at baseline (PRE), POST0, POST30, and POST60 minute time points relative to the PAS protocol delivery.
2.2Assessment of median nerve somatosensory evoked potentials (SEPs)
To evaluate SEPs, a standard bar electrode was placed over the median nerve of the paretic hand with the cathode proximal and the distal end of the electrode aligned with the wrist crease. Localization of stimulation was based on participant report of tingling sensation in the hand territory of innervation. Once the site was determined, stimuli (200μs square wave, monophasic pulse) were delivered a constant current stimulator (DS7A, Digitimer Ltd.) at increasing intensities until an M-wave of 1 mV in peak-to-peak amplitude was evoked in the paretic APB muscle.
During SEP assessment, two hundred stimuli (∼1 mV threshold intensity) were delivered at 3 Hz while the paretic APB was maintained at rest (confirmed by online monitoring of continuous EMG signals by one of the study staff). Participants were asked to report any discomfort or abnormal sensations during the assessment. Electroencephalography (EEG) data were continuously recorded (sampling frequency: 5000 Hz, impedance:<5kΩ, frequency range: 0–1000 Hz, 0.5μV/bit resolution) using a 32-channel TMS-compatible electrode cap (Easy Cap) and amplifier (BrainAmp DC, Brain Products Ltd). The latency of the mean peak of the N20 component of the SEP was extracted from the electrode overlying the primary somatosensory cortex (S1) in the ipsilesional hemisphere (CP3 or CP4) using custom Matlab functions and EEGLAB (Delorme & Makeig, 2004). The individual N20 latency was determined for each participant and used to determine the interstimulus interval for PAS delivery and the assessment of short afferent inhibition (SAI) described below.
2.3Assessments of corticomotor excitability
Corticomotor excitability was assessed at PRE, POST0, POST30, and POST60 minute time points relative to the PAS protocol measuring the peak-to-peak amplitude of the motor evoked potential (MEP) response. During baseline TMS thresholding procedures and cortical excitability assessments, participants were seated upright comfortably in an arm chair while monophasic magnetic pulses with a 100μs approximate rise time and a 1.0 ms total pulse duration were delivered through a 70 mm hand-held figure-of-eight coil connected to two Magstim 2002 stimulators through a Bistim module (MagStim Ltd., Wales, UK). The coil was oriented perpendicular to the central sulcus to induce a posterior-anterior current in the primary motor cortex (M1) of the ipsilesional hemisphere (Devanne, Lavoie, & Capaday, 1997). Cortical excitability was evaluated by measuring the peak-to-peak amplitude of the motor evoked potential (MEP) response using surface electromyography (EMG). EMG activity was recorded using surface electrodes (9 mm diameter, 4–5 mm inter-electrode distance) that were carefully positioned and affixed to the skin overlying the paretic APB muscle belly, with the electrodes aligned parallel to the muscle fiber orientation. EMG data were sampled using a 16 channel EMG system (BrainAmp ExG amplifier, Brain Products GmbH) at a rate of 5000 Hz and band-pass filtered at 10–1000 Hz. Stereotactic neuronavigation software (BrainSight®, Rogue Research Inc.) utilizing each participant’s high-resolution T1 anatomical MRI image (TR = 7.4 ms, TE = 3.7 ms, flip angle θ= 6°, FOV = 256 mm, 160 slices, 1 mm thickness) that was collected prior to the first TMS session. This MRI image was used to map the location of the target paretic abductor pollicis brevis (APB) muscle representation within the lesioned M1. The optimal site for TMS was determined for each participant using standard procedures (Devanne et al., 1997). The selected hotspot and coil orientation were registered with Brainsight® for each participant and used in real-time to ensure coil placement and orientation remained consistent for all TMS assessment measures and the PAS intervention.
Prior to assessment or intervention, the resting motor threshold (RMT) needed to produce a > 50μV peak-to-peak amplitude MEP response in at least 3 out of 5 trials was determined for each participant. Additionally, we determined the TMS intensity needed to produce a ∼1 mV MEP response in the same proportion of trials (Castel-Lacanal et al., 2009; Nitsche et al., 2007; Player et al., 2012). If a 1 mV MEP could not be elicited, the maximal MEP amplitude was determined and stimulator intensity was set to elicit a MEP 50% of the maximum amplitude to minimize potential floor or ceiling effects during excitability assessments. At each assessment, TMS was delivered over the ipsilesional M1 APB hotspot at a rate of 0.1 to 0.25 Hz as 20 MEPs were obtained for each TMS condition. Given attention levels can affect the corticomotor response (Stefan et al., 2004), a visual stimulus was present on the computer screen positioned directly in front of the participant at randomly timed intervals. The participant counted the number of visual stimuli and reported the number after each assessment. The following TMS assessments were performed:
SINGLE-PULSE 1 mV MEP RESPONSE: The test stimulus was set to the ∼1 mV threshold identified before the PAS intervention. Thresholds were not re-evaluated at post-PAS assessments.
SHORT AFFERENT INHIBITION (SAI): The influence of afferent input to primary somatosensory cortex on corticomotor output and the subsequent MEP response constituted a measure of SAI. Single electrical stimuli applied over the paretic median nerve preceded single TMS pulses over the lesioned M1. During the SAI assessment, an interstimulus interval of individual N20 latency plus 3 ms (PASN20 +3) between the median nerve (intensity: ∼1 mV M wave) and M1 stimulation (intensity: ∼1 mV MEP) was used.
SHORT INTERVAL INTRACORTICAL INHIBITION (SICI) AND INTRACORTICAL FACILITATION (ICF): Inhibitory and facilitatory intracortical components of general corticomotor excitability assessed using SICI and ICF, respectively. The interstimulus intervals between the conditioning and test TMS pulse were set at 2 ms (SICI) and 12 ms (ICF) (Di Lazzaro et al., 2006). The conditioning stimulus was set at an intensity of 80% RMT while the test stimulus was set at the same ∼1 mV MEP intensity used for the single-pulse MEP measures (Ziemann, Rothwell, & Ridding, 1996).
2.4Paired associative stimulation (PAS) paradigm
Participants completed two PAS conditions (PAS and PASSHAM) performed approximately one week apart. The order of each session was randomized. During both sessions, participants were seated comfortably in a chair with arms resting in a pronated position on a pillow on a table set just below the level of the chest. The paretic median nerve was stimulated at the same intensity used for SAI assessment procedures and was held constant for the duration of the PAS session. The site of stimulation was over the ipsilesional M1 motor hotspot and the intensity was set to the ∼1 mV threshold for the paretic APB muscle, as described above.
During the PAS session, a single electrical pulse applied over the paretic median nerve was combined with single-pulse TMS over the lesioned M1. For the PAS protocol, the interstimulus interval between the median nerve and M1 stimulation was the individual N20 latency plus 5 ms to account for conduction time between S1-M1 cortico-cortical connections (Conde et al., 2013; Goldring, Aras, & Weber, 1970) (PASN20 +5). Each PAS session consisted of 180 pairs of stimuli (median nerve and TMS) delivered at 0.25 Hz. During the PAS session, participants were instructed to keep APB muscles relaxed bilaterally (verified by monitoring of continuous EMG activity). Due to the effects of attention on PAS-induced plasticity, participants were also asked to count the number of visual stimuli that appeared directly over the paretic APB muscle location (Stefan et al., 2004). The PASSHAM session followed identical procedures to the PAS session, except that TMS pulses were delivered with the orientation of the coil perpendicular to the scalp to minimize biological effects of stimulation.
2.5Data reduction and analyses
2.5.1Corticomotor excitability
At each assessment, the peak-to-peak MEP amplitudes were averaged for each participant. Mean amplitudes of SAI, SICI and ICF were expressed as a ratio of conditioned over unconditioned (1 mV) MEP responses. Percent change relative to the PRE testing time point was calculated for each MEP variable as [(PRE – POST)/PRE]*100 (Nitsche et al., 2007; Player et al., 2012).
2.5.2Motor skill performance
The average RT of the 50 key presses completed in the first random block of the modified SRTT was calculated for each assessment for each hand. The RT (reaction time + movement time) was defined as the time between the visual stimulus presentations to the time of the correct key press.
All MEP data for each condition were tested for normality and homogeneity of variance using Kolmogorov-Smirnov and Levene’s tests respectively. If these tests met assumptions of normality and homogeneity of variance as indicated by a non-significant test result, then statistical testing was performed using parametric procedures. Separate repeated measures analysis of variance (ANOVA) tests with within-subject factors of time (PRE, POST0, POST30, POST60) and condition (PAS and PASSHAM) were used to test the impact of PAS on corticomotor excitability for all MEP amplitude change measures (1 mV, SAI, SICI, and ICF) and response time. In an exploratory analysis, the relationship between changes in MEP amplitude and changes in response time for measures showing within-subjects effects using Pearson product moment correlation coefficients. All analyses were performed using SPSS version 24 with a critical α level set to 0.05.
3Results
Complete data sets for cortical excitability assessments were obtained for 6 participants in the PAS condition and 5 participants in the PASSHAM condition. During the PAS condition, S08 was unable to complete POST30 and POST60 testing time points because of technical issues with data recording hardware. During the PASSHAM condition, S04 and S08 could not complete the POST60 TMS testing due to time constraints. Complete behavioral datasets were obtained for 5 participants. During both conditions, S06 and S08 could not complete the SRTT keyboard task with their paretic hand due to the magnitude of their motor impairment. Therefore, their behavioral data were not analyzed. The MEP data from each testing condition met assumptions of normality and homogeneity of variance.
3.1Corticomotor excitability
A significant increase in MEP amplitude occurred following the PAS but not the PASSHAM condition (Fig. 2) during the 1 mV condition. Analyses revealed a significant main effect of condition (F1,5 =14.59, p = 0.02), with greater MEP amplitude increase during the PAS (85.39±78.57%) than the PASSHAM (12.88±39.85%) condition. We did not observe a significant condition-by-time interaction (F3,5 = 1.95, p = 0.18) or a main effect of time (F3,5 = 2.53, p = 0.11) during the 1 mV testing. During the SAI condition, there was a trend towards a change in MEP amplitude over time (F1,5 = 4.23, p = 0.06) but no observable difference between PAS or PASSHAM conditions (F1,5 = 0.15, p = 0.74, Fig. 3A). For the SICI and ICF conditions, there was neither an effect of time (SICI: F1,5 = 0.49, p = 0.7; ICF: F1,5 = 0.63, p = 0.62) nor condition (SICI: F1,5 = 4.66, p = 0.16; ICF: F1,5 = 0.45, p = 0.57) (Fig. 3B, C).
Fig.2
Group (left) and individual (right) MEP amplitude (mean±SE) during the 1 mV TMS testing condition. A) Average MEP amplitudes value during PAS and PASSHAM conditions. B) Average MEP amplitude expressed as a percent change from the PRE test value. *indicates main effect of condition. C) Individual % change in MEP responses following the PAS and D) PASSHAM conditions.
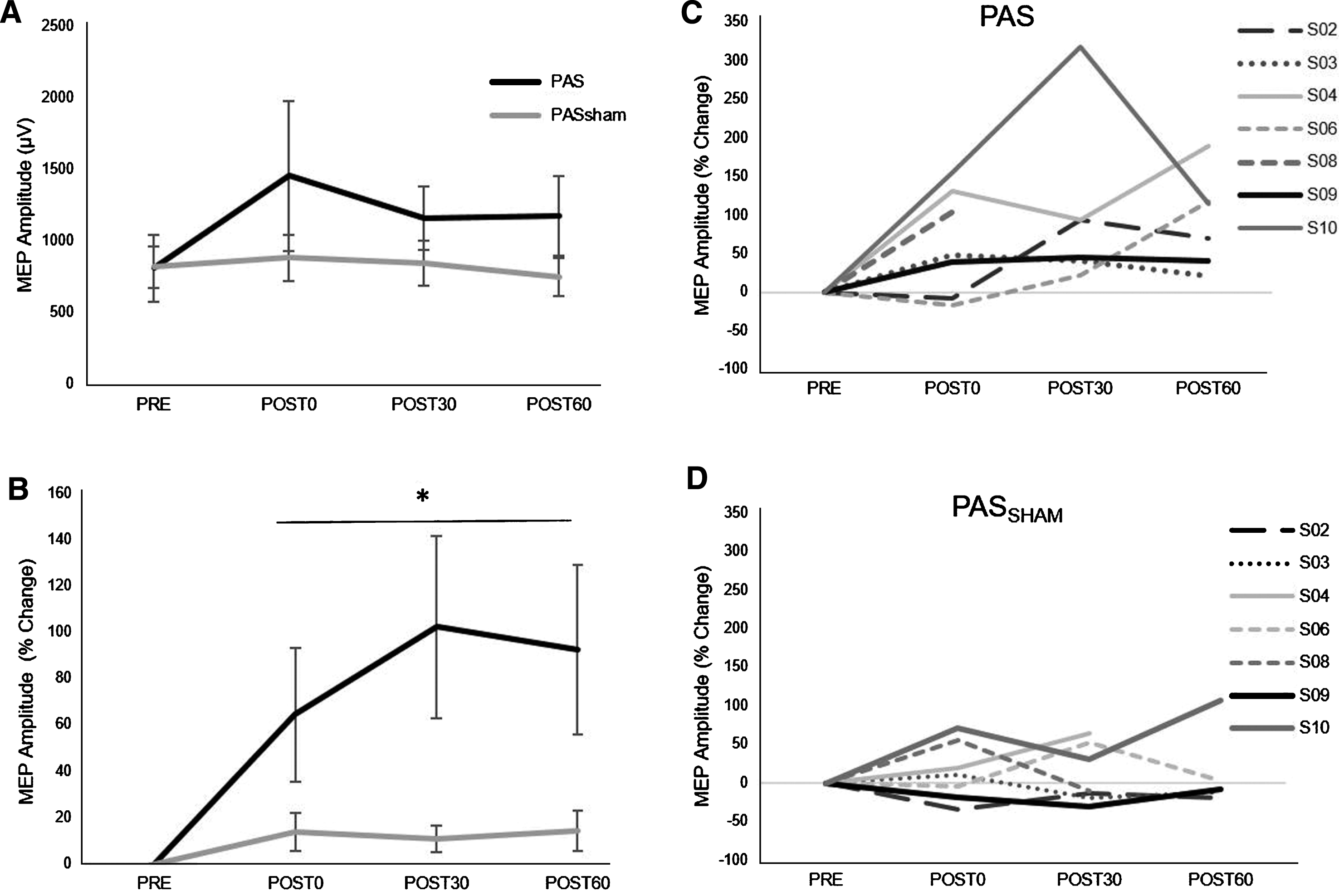
Fig.3
Group normalized MEP amplitude (mean±SE) during the PAS and PASSHAM conditions for A) SAI, B) SICI, and C) ICF TMS tests. No significant interaction or main effects of condition or time were observed. (SAI: short afferent inhibition, SICI: short interval intracortical inhibition, ICF: intracortical facilitation).
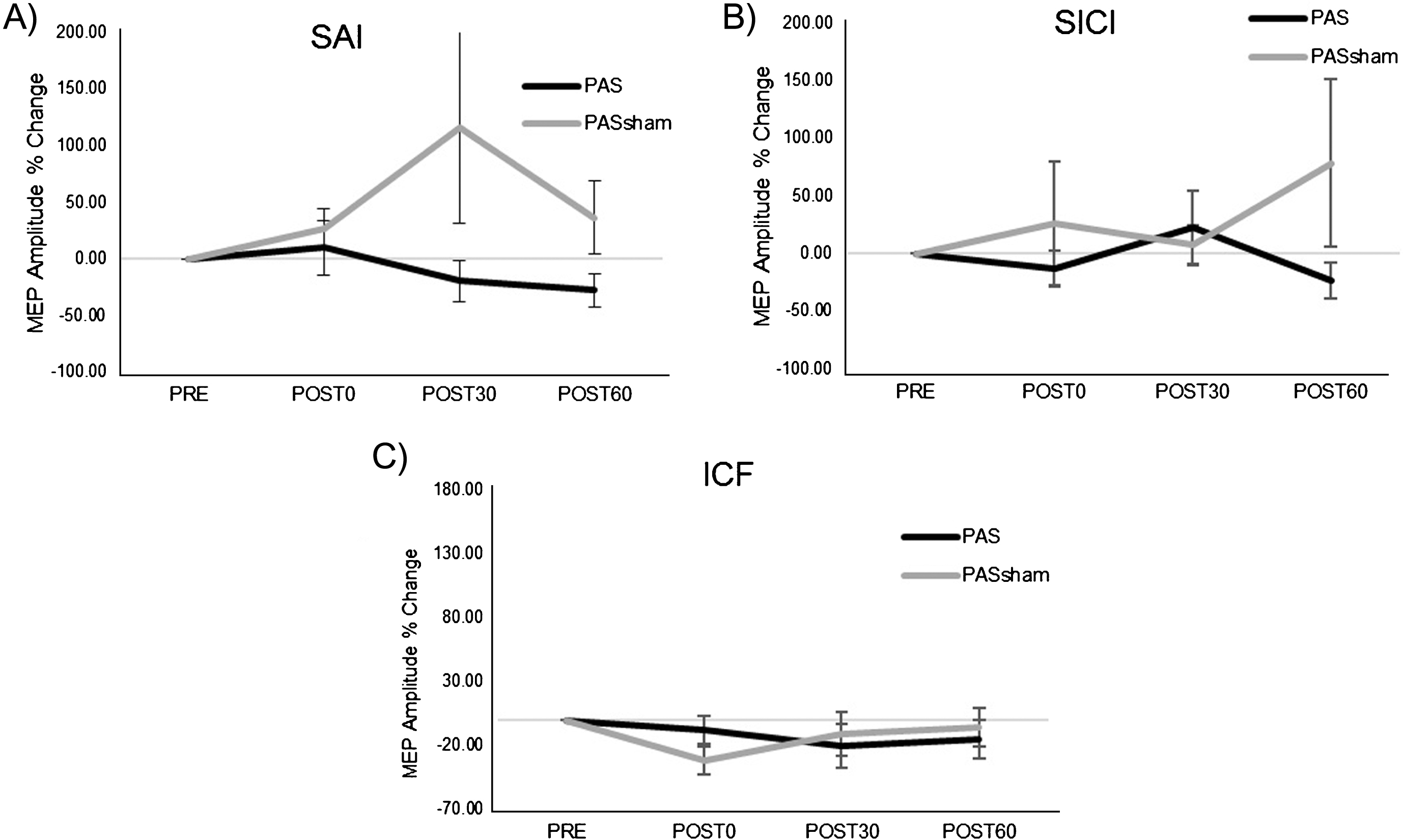
3.2Behavioral measures
There was an overall decrease in RT in both paretic and nonparetic limbs over time in both PAS and PASSHAM condition. There was not a significant difference in RT between PAS and PASSHAM conditions, as indicated by no effect of condition for either the paretic (F1,4 = 0.48, p = 0.54) or nonparetic limbs (F1,4 = 0.57, p = 0.49). Improved motor skill performance was observed (decreased RT) over time in both limbs, though the paretic limb failed to meet our adopted level of significance (paretic: F1,5 = 2.64, p = 0.11; nonparetic: F1,5 = 5.12, p = 0.02) (Fig. 4). Correlation analyses revealed a significant negative relationship between change in MEP amplitude (1 mV) versus change in RT across POST testing time points (r = –0.651, p < 0.01) (Fig. 5) where larger increases in MEP amplitude were correlated with faster RTs during SRTT performance.
Fig.4
Response time (RT) on a modified version of the SRTT (mean±SE) for each hand during PAS and PASSHAM conditions during the first random block for testing time point. There was no significant difference in RT between PAS and PASSHAM conditions for either the paretic or nonparetic hand performance. RT significantly decreased over time in the nonparetic hand (p = 0.02) and in the paretic hand but was not a statistically significant effect. (P: paretic, NP: nonparetic).
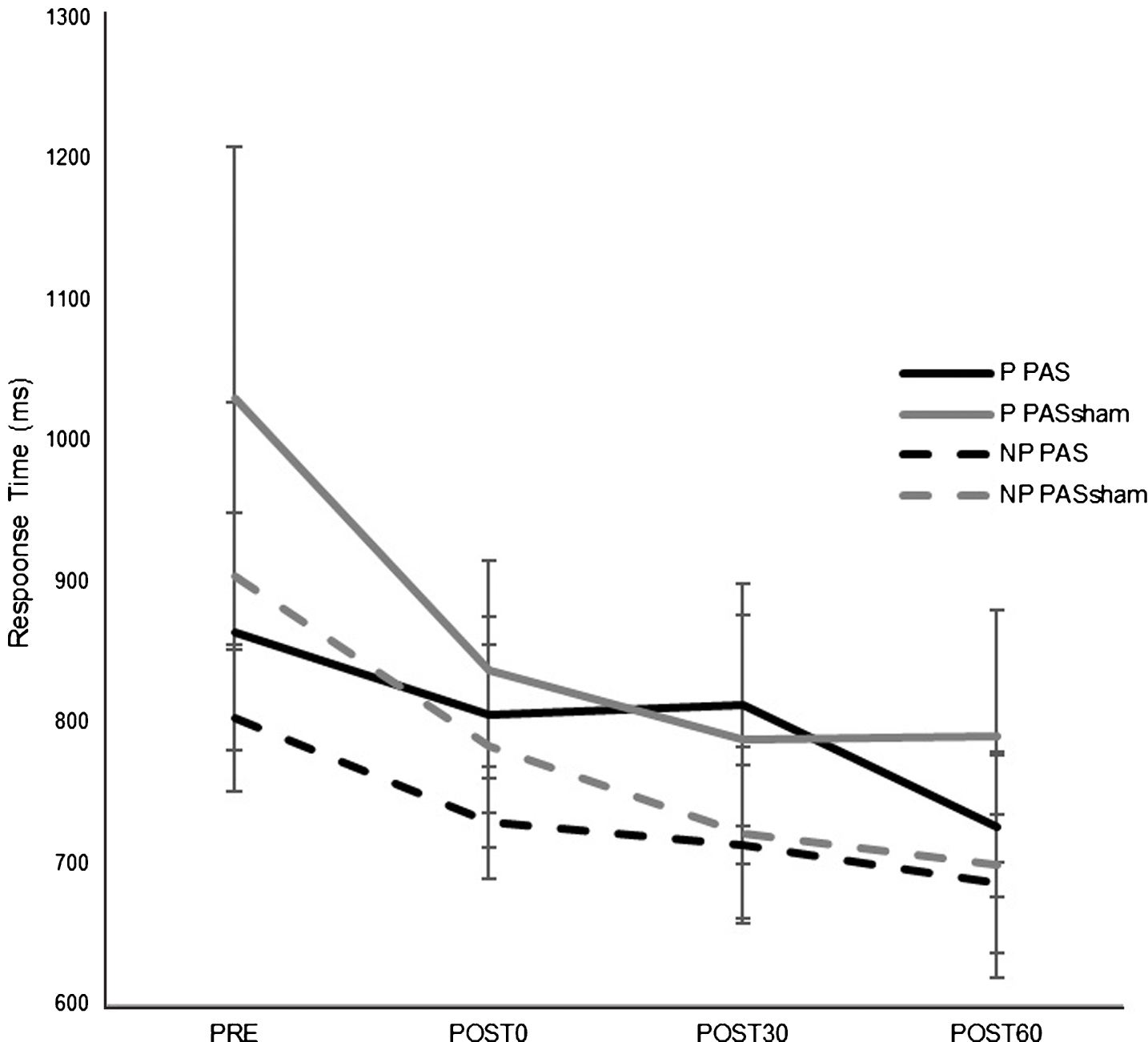
Fig.5
Relationship between change in MEP amplitude (1 mV) (mean±SE) versus change in SRTT RT. Exploratory analyses showed a negative relationship between change in MEP amplitude change in RT across POST testing time points suggesting greater PAS-induced increases in cortical excitability were associated with larger improvements in motor skill performance (lower RTs).
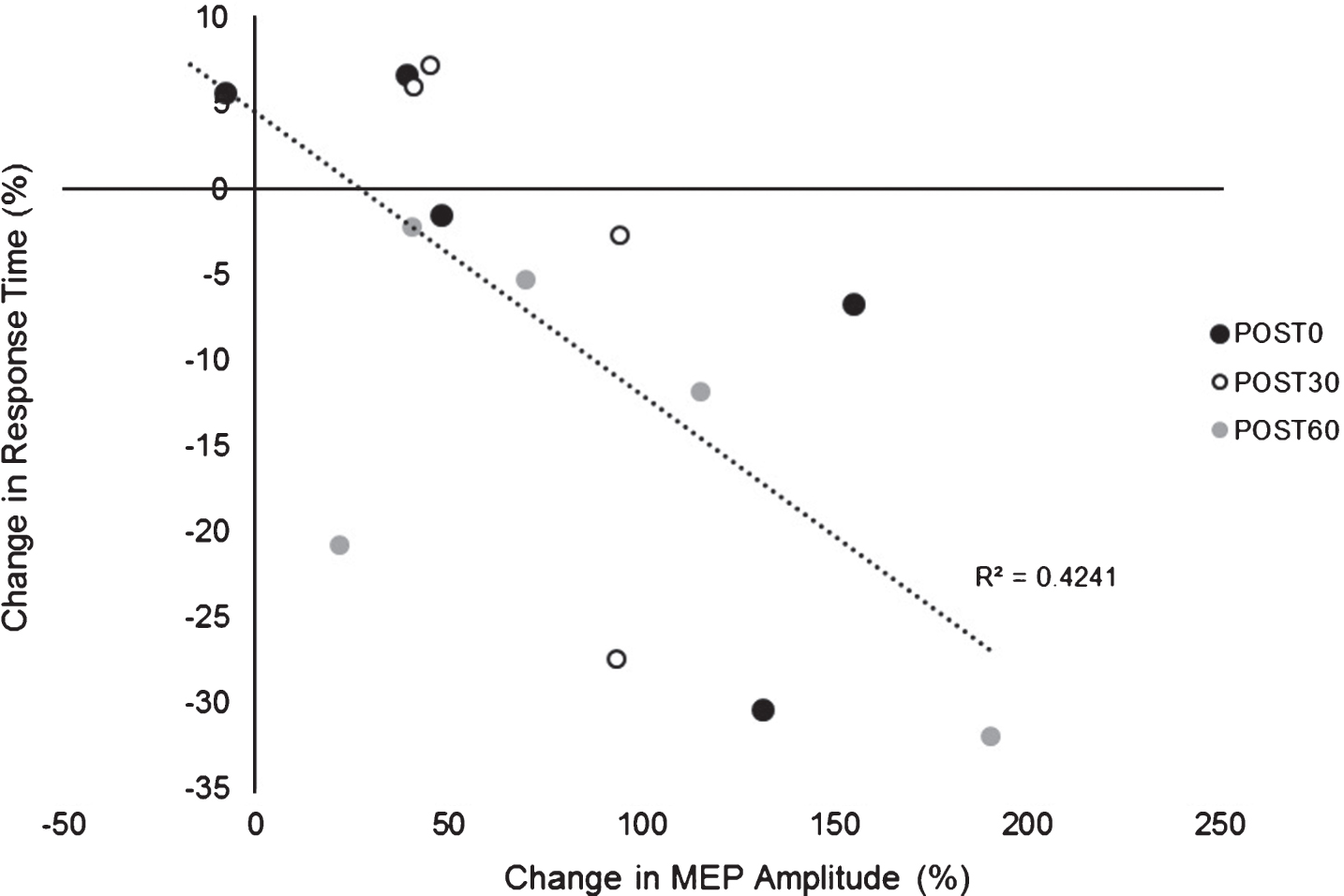
4Discussion
Results of the present study suggest a traditional PAS protocol can increase corticomotor excitability in individuals in the chronic stage of post-stroke motor recovery. PAS did not significantly impact the degree of motor performance improvement in either the paretic or nonparetic limb, although both paretic and nonparetic limbs showed improvements in motor performance over time. Interestingly, exploratory correlation analyses provide preliminary evidence of an association between change in corticomotor excitability and the degree of motor performance improvement following PAS in individuals post-stroke. Together, these findings suggest that PAS may effectively enhance neuroplasticity in a subgroup of individuals post-stroke and could potentially offer a therapeutic adjuvant in combination with rehabilitation strategies to enhance motor recovery in these individuals.
Following PAS, we observed increases in a general measure of corticomotor excitability of the paretic hand following a time course similar to that previously described in neurologically-intact individuals (Player et al., 2012) and in individuals post-stroke following a modified PAS protocol (Castel-Lacanal et al., 2009) (Fig. 2). We did not observe a significant change in corticomotor excitability following the PASSHAM condition in the same individuals. The PAS-induced neuroplastic adaptations observed likely occur through LTP-like associative plasticity mechanisms (Nitsche et al., 2007; Stefan et al., 2002) and appear to occur specifically in cortical representations of muscles innervated by the peripheral nerve that was stimulated (Nitsche et al., 2007). In the present study, we observed the average magnitude of MEP amplitude increase at all post PAS time points in individuals post-stroke (POST0: +65%, POST30: +102%, POST60: +93%). Interestingly, this magnitude of corticomotor excitability increase was at least comparable to that previously reported in neurologically-intact controls and greater than that reported in elderly individuals where facilitatory PAS did not induce a significant neuroplastic effect (Muller-Dahlhaus et al., 2008; Player et al., 2012; Stefan et al., 2002). Additionally, previous reports found that only 66–76% of young neurologically-intact individuals responded to similar facilitatory PAS protocols immediately following the intervention (Muller-Dahlhaus et al., 2008; Player et al., 2012; Stefan et al., 2004). These findings are consistent with our results where 5/7 participants showed the expected response at the POST0 time point immediately following PAS. All participants in this study, however, also showed MEP facilitation at 30 and 60 minutes following PAS and no participants demonstrated a robust long-term depression (LTD)-like response to PAS, which is in contrast to some previous reports in young neurologically-intact controls (Muller-Dahlhaus et al., 2008; Player et al., 2012). Reasons for differences in both the magnitude of the response and the rate of responders between individuals post-stroke in the present study and previous studies could lie in differences in patient populations and the corticomotor potential for enhanced excitability. A greater potential for corticomotor excitability increase from already decreased baseline levels of corticomotor excitability in the ipsilesional cortex in individuals post-stroke may occur as a result of the lesion itself or could occur secondarily through disuse of the paretic arm and hand (Ngomo, Leonard, & Mercier, 2012). Moreover, the customization of the interstimulus interval based on individual N20 latencies used in the PAS protocol of the present study may have contributed to a greater magnitude of response and larger percentage of responders compared to previous reports (Muller-Dahlhaus et al., 2008; Player et al., 2012). Future research is needed to elucidate the potential underlying factors contributing to differences in results between studies involving different patient populations.
Further, we sought to investigate intracortical mechanisms that may have contributed to observed changes in general corticomotor excitability through intracortical inhibition of M1 elicited by afferent input (SAI) (Tokimura et al., 2000) and measures of paired-TMS pulse inhibition (SICI) and facilitation (ICF). The increased MEP amplitudes following PAS may be a consequence of reduction in intracortical inhibition on excitatory synapses onto local pyramidal neurons, as has been proposed by previous work regarding effects of PAS in individuals post-stroke (Castel-Lacanal et al., 2009). However, consistent with previous research that examined the effect of PAS on these intracortical measures in neurologically-intact participants, our results showed no significant change in intracortical measures of SAI, SICI, and ICF following PAS or PASSHAM conditions (Fig. 3) (Ridding, McKay, Thompson, & Miles, 2001; Russmann et al., 2009; Sale et al., 2007; Stefan et al., 2002). Thus, results of the present study do not support the concept that changes in intracortical inhibition or intracortical facilitation underlie PAS-induced changes in general corticomotor excitability in individuals post-stroke. It is possible that PAS-induced increases in corticomotor excitability post-stroke may have occurred subcortically, possibly through changes in thalamo-cortical connectivity via projections from thalamic nuclei to primary somatosensory and motor cortices (Carson & Kennedy, 2013). The afferent input and efferent stimuli of the facilitatory PAS protocol could have strengthened the disrupted connectivity in these pathways observed in neurologically-impaired and elderly adults (Fathi et al., 2010; Ueki et al., 2006) and potentially increased general corticomotor excitability within the primary motor cortex. This conjecture may be supported by previous work that demonstrated altering cerebellar neuronal activity through transcranial direct current stimulation (tDCS) blocked the effects of a facilitatory PAS protocol in neurologically-intact individuals, presumably by acting on thalamic synapses projecting to somatosensory and motor cortices (Hamada et al., 2012). Similar disruption studies of this type performed in individuals after stroke would be useful for understanding the neuroanatomical origins of PAS-induced neuroplasticity in these patient populations.
Evaluation of motor performance on the modified SRTT showed improvements in RT that occurred over time with task practice in both paretic and nonparetic limbs, with no observed differences between PAS versus the PASSHAM protocol (Fig. 4). In contrast to previous results in young neurologically-intact individuals (Celnik et al., 2009; Player et al., 2012) these results suggest that PAS did not enhance motor performance on this task in a cohort of individuals post-stroke after a single application. Interestingly, novel exploratory analyses showed a relationship between change in MEP amplitude and change in RT (Fig. 5). Individuals post-stroke with the largest PAS-induced increases in corticomotor excitability showed the greatest improvements in motor performance following PAS. A similar relationship between PAS-induced enhancements of corticomotor excitability and motor performance was previously reported in individuals with schizophrenia (Frantseva et al., 2008) while others did not detect a relationship in young neurologically-intact participants (Player et al., 2012). Together, these data suggest that differences in the neural mechanisms mediating PAS-related motor skill performance enhancement may exist between neurologically-intact versus neurologic patient populations. Future work is needed to compare PAS-induced neuroplastic and behavioral changes between individuals post-stroke and matched controls and the effectiveness over long-term interventions.
The facilitation of corticomotor excitability after PAS was highly variable between individuals in this study with levels similar to those previously reported in neurologically-intact individuals (Stefan, Kunesch, Cohen, Benecke, & Classen, 2000b) and in wrist extensor muscles of individuals post-stroke (Castel-Lacanal et al., 2007). Though mean MEP amplitudes demonstrated increases in corticomotor excitability following PAS, individuals’ overall responses to PAS at all POST testing time points ranged from –17 to +318% increase from baseline. Variability in response to interventions is commonly observed in post-stroke patient populations (Koski et al., 2004; Palmer, Hsiao, Wright, & Binder-Macleod, 2017). Participants in the present study showing the greatest magnitude or corticomotor excitability increase may have sufficient neural substrate supporting PAS-induced enhancement of motor skill performance (Koski, Mernar, & Dobkin, 2004; Rushton, 2003). Though baseline measures of corticomotor function generally show limited prognostic ability for rehabilitation outcomes in the chronic stage of stroke recovery (Koski et al., 2004), individual corticomotor response to a single session of PAS might potentially be an important indicator for response to a long-term intervention, similar to findings reported for other post-stroke intervention strategies (Koski et al., 2004). Specifically, if corticomotor response to a single session of PAS revealed that an individual possessed a high degree of associative plasticity, then that individual may be a good candidate for subsequent PAS sessions coupled with rehabilitation focused on improving paretic hand function. In contrast, if corticomotor response to a single PAS session showed a low degree of associative plasticity, then PAS may not be an effective tool for that individual and rehabilitation efforts could focus on nonparetic limb compensation to maximize motor function. In this way, measurements of corticomotor response to a single PAS session could have clinical utility in the quantification of individual neuroplastic responses and ultimately help to individualize stroke rehabilitation for a heterogeneous patient population and maximize post-stroke functional outcomes. Additionally, Harris-Love and Harington (Harris-Love & Harrington, 2017) suggested using a framework which incorporates patient-, lesion site- and task-specific factors that may help to individualize stroke rehabilitation and improve outcomes for interventions utilizing noninvasive brain stimulation. Future research is needed to confirm these findings in larger stroke cohorts to determine specific patient characteristics that may explain variability in individual responses to PAS and to identify those individuals who may benefit most from PAS interventions.
4.1Limitations
Some limitations of the present study are important to consider in the interpretation of the results. The preliminary nature and small sample size of this study may have led to underpowered statistical analyses, particularly regarding analyses of intracortical and motor behavioral measures. The small cohort coupled with heterogeneity of lesion location and levels of motor impairment could have contributed to the high variability of responses to the PAS intervention. In this study, behavioral measures were a secondary outcome and consisted of a motor task that was found to be too challenging for two participants to perform with the paretic hand; future studies should consider a motor behavioral task assessment to allow for inclusion of individuals post-stroke across a wider spectrum of motor impairment levels. All participants in the present study were mild to moderately impaired; however, more severely impaired individuals after stroke could show different responses to PAS (McCambridge, Stinear, & Byblow, 2017). Additionally, we did not report a clinical measure of motor function in response to PAS, which will be a necessary step for demonstrating the therapeutic potential of PAS in stroke rehabilitation. Due to the number of assessments and time constraints of the testing session, we did not perform neurophysiologic assessments of the contralesional hemisphere. Assessment of potential neurophysiologic changes in the contralesional hemisphere following PAS could provide useful and important information, potentially explaining some observed changes within the ipsilesional hemisphere, and should be investigated in future studies. Lastly, we did not evaluate the status or integrity of other cortical pathways that could offer other potential targets for therapeutic applications (Harris-Love & Harrington, 2017).
5Conclusions
The present findings provide preliminary evidence for the effectiveness of PAS to induce changes in cortical neuroplasticity in chronic individuals post-stroke and are an important step for future studies investigating the clinical application of PAS interventions in patient populations. The observed findings support the effectiveness of PAS to enhance corticomotor excitability in individuals post-stroke and provide novel evidence that a relationship exists between PAS-induced corticomotor excitability change and post-stroke motor skill performance. Future work will need to clarify the behavioral relevance of PAS-induced corticomotor excitability changes in chronic stroke patient populations and neurophysiologic factors underlying the interindividual variability in response to PAS intervention.
Conflicts of interest
None.
Acknowledgments
Research reported in this publication was supported by the American Heart Association [AHA00035638] and the Eunice Kennedy Shriver National Institute Of Child Health & Human Development of the National Institutes of Health under Award Number K12HD055931. The content is solely the responsibility of the authors and does not necessarily represent the official views of the National Institutes of Health.
References
1 | Borich M.R. , Brodie S.M. , Gray W.A. , Ionta S. , & Boyd L.A. ((2015) ) Understanding the role of the primary somatosensory cortex: Opportunities for rehabilitation. Neuropsychologia, doi: 10.1016/j.neuropsychologia.2015.07.007 |
2 | Carson R.G. , & Kennedy N.C. ((2013) ) Modulation of human corticospinal excitability by paired associative stimulation. Frontiers in Human Neuroscience, 7: , 823. doi: 10.3389/fnhum.2013.00823 |
3 | Castel-Lacanal E. , Gerdelat-Mas A. , Marque P. , Loubinoux I. , & Simonetta-Moreau M. ((2007) ) Induction of cortical plastic changes in wrist muscles by paired associative stimulation in healthy subjects and post-stroke patients. Experimental Brain Research, 180: (1), 113–122. doi: 10.1007/s00221-006-0844-5 |
4 | Castel-Lacanal E. , Marque P. , Tardy J. , de Boissezon X. , Guiraud V. , & Chollet F. Moreau M.S. ((2009) ) Induction of cortical plastic changes in wrist muscles by paired associative stimulation in the recovery phase of stroke patients. Neurorehabilitation and Neural Repair, 23: (4), 366–372. doi: 10.1177/1545968308322841 |
5 | Celnik P. , Paik N.J. , Vandermeeren Y. , Dimyan M. , & Cohen L.G. ((2009) ) Effects of combined peripheral nerve stimulation and brain polarization on performance of a motor sequence task after chronic stroke. Stroke, 40: (5), 1764–1771. doi: 10.1161/STROKEAHA.108.540500 |
6 | Cohen D.A. , Pascual-Leone A. , Press D.Z. , & Robertson E.M. ((2005) ) Off-line learning of motor skill memory: A double dissociation of goal and movement. Proceedings of the National Academy of Sciences of the United States of America, 102: (50), 18237–18241. doi: 0506072102 [pii] |
7 | Conde V. , Vollmann H. , Taubert M. , Sehm B. , Cohen L.G. , Villringer A. , & Ragert P. ((2013) ) Reversed timing-dependent associative plasticity in the human brain through interhemispheric interactions. Journal of Neurophysiology, 109: (9), 2260–2271. doi: 10.1152/jn.01004.2012 |
8 | Delorme A. , & Makeig S. ((2004) ) EEGLAB: An open source toolbox for analysis of single-trial EEG dynamics including independent component analysis. Journal of Neuroscience Methods, 134: (1), 9–21. doi: 10.1016/j.jneumeth.2003.10.009 |
9 | Devanne H. , Lavoie B.A. , & Capaday C. ((1997) ) Input-output properties and gain changes in the human corticospinal pathway. Experimental Brain Research, 114: (2), 329–338. |
10 | Di Lazzaro V. , Pilato F. , Oliviero A. , Dileone M. , Saturno E. , Mazzone P. , & Rothwell J.C. ((2006) ) Origin of facilitation of motor-evoked potentials after paired magnetic stimulation: Direct recording of epidural activity in conscious humans. Journal of Neurophysiology, 96: (4), 1765–1771. doi: 00360.2006 |
11 | Elsner B. , Kugler J. , Pohl M. , & Mehrholz J. ((2016) ) Transcranial direct current stimulation (tDCS) for improving activities of daily living, and physical and cognitive functioning, in people after stroke. The Cochrane Database of Systematic Reviews, 3: doi: 10.1002/14651858 |
12 | Fathi D. , Ueki Y. , Mima T. , Koganemaru S. , Nagamine T. , Tawfik A. , & Fukuyama H. ((2010) ) Effects of aging on the human motor cortical plasticity studied by paired associative stimulation. Clinical Neurophysiology: Official Journal of the International Federation of Clinical Neurophysiology, 121: (1), 90–93. doi: 10.1016/j.clinph.2009.07.048 |
13 | Frantseva M.V. , Fitzgerald P.B. , Chen R. , Moller B. , Daigle M. , & Daskalakis Z.J. ((2008) ) Evidence for impaired long-term potentiation in schizophrenia and its relationship to motor skill learning. Cerebral Cortex (New York, N.Y.:1991), 18: (5), 990–996. doi: bhm151 |
14 | Goldring S. , Aras E. , & Weber P.C. ((1970) ) Comparative study of sensory input to motor cortex in animals and man. Electroencephalography and Clinical Neurophysiology, 29: (6), 537–550. |
15 | Hamada M. , Strigaro G. , Murase N. , Sadnicka A. , Galea J.M. , Edwards M.J. , & Rothwell J.C. ((2012) ) Cerebellar modulation of human associative plasticity. The Journal of Physiology, 590: (10), 2365–2374. doi: 10.1113/jphysiol.2012.230540 |
16 | Hao Z. , Wang D. , Zeng Y. , & Liu M. ((2013) ) Repetitive transcranial magnetic stimulation for improving function after stroke. The Cochrane Database of Systematic Reviews, 5: , CD008862. doi: 10.1002/14651858.CD008862.pub2 |
17 | Harris-Love M.L. , & Harrington R.M. ((2017) ) Non-invasive brain stimulation to enhance upper limb motor practice poststroke: A model for selection of cortical site. Frontiers in Neurology, 8: , 224. doi: 10.3389/fneur.2017.00224 |
18 | Humeau Y. , Shaban H. , Bissiere S. , & Luthi A. ((2003) ) Presynaptic induction of heterosynaptic associative plasticity in the mammalian brain. Nature, 426: (6968), 841–845. doi: 10.1038/nature02194 |
19 | Kilpatrick C.J. , Davis S.M. , Tress B.M. , Rossiter S.C. , Hopper J.L. , & Vandendriesen M.L. ((1990) ) Epileptic seizures in acute stroke. Archives of Neurology, 47: (2), 157–160. |
20 | Koski L. , Mernar T.J. , & Dobkin B.H. ((2004) ) Immediate and long-term changes in corticomotor output in response to rehabilitation: Correlation with functional improvements in chronic stroke. Neurorehabilitation and Neural Repair, 18: (4), 230–249. doi: 18/4/230 |
21 | Kujirai K. , Kujirai T. , Sinkjaer T. , & Rothwell J.C. ((2006) ) Associative plasticity in human motor cortex during voluntary muscle contraction. Journal of Neurophysiology, 96: (3), 1337–1346. doi: 01140.2005 |
22 | Manganotti P. , Patuzzo S. , Cortese F. , Palermo A. , Smania N. , & Fiaschi A. ((2002) ) Motor disinhibition in affected and unaffected hemisphere in the early period of recovery after stroke. Clinical Neurophysiology, 113: (6), 936–943. doi: 10.1016/S1388-2457(02)00062-7 |
23 | McCambridge A.B. , Stinear J.W. , & Byblow W.D. ((2017) ) Revisiting interhemispheric imbalance in chronic stroke: A tDCS study. Clinical Neurophysiology: Official Journal of the International Federation of Clinical Neurophysiology, 129: (1), 42–50. doi: S1388-2457(17)31108-2 |
24 | Muller-Dahlhaus J.F. , Orekhov Y. , Liu Y. , & Ziemann U. ((2008) ) Interindividual variability and age-dependency of motor cortical plasticity induced by paired associative stimulation. Experimental Brain Research, 187: (3), 467–475. doi: 10.1007/s00221-008-1319-7 |
25 | Ngomo S. , Leonard G. , & Mercier C. ((2012) ) Influence of the amount of use on hand motor cortex representation: Effects of immobilization and motor training. Neuroscience, 220: , 208–214. doi: 10.1016/j.neuroscience.2012.06.018 |
26 | Nitsche M.A. , Roth A. , Kuo M.F. , Fischer A.K. , Liebetanz D. , Lang N. , & ... Paulus W. ((2007) ) Timing-dependent modulation of associative plasticity by general network excitability in the human motor cortex. The Journal of Neuroscience: The Official Journal of the Society for Neuroscience, 27: (14), 3807–3812. doi: 27/14/3807 |
27 | Palmer J.A. , Hsiao H. , Wright T. , & Binder-Macleod S.A. ((2017) ) Single session of functional electrical stimulation-assisted walking produces corticomotor symmetry changes related to changes in poststroke walking mechanics. Physical Therapy, 97: (5), 550–560. doi: 10.1093/ptj/pzx008 |
28 | Player M.J. , Taylor J.L. , Alonzo A. , & Loo C.K. ((2012) ) Paired associative stimulation increases motor cortex excitability more effectively than theta-burst stimulation. Clinical Neurophysiology: Official Journal of the International Federation of Clinical Neurophysiology, 123: (11), 2220–2226. doi: 10.1016/j.clinph.2012.03.081 |
29 | Rapisarda G. , Bastings E. , de Noordhout A.M. , Pennisi G. , & Delwaide P.J. ((1996) ) Can motor recovery in stroke patients be predicted by early transcranial magnetic stimulation? Stroke; a Journal of Cerebral Circulation, 27: (12), 2191–2196. |
30 | Ridding M.C. , McKay D.R. , Thompson P.D. , & Miles T.S. ((2001) ) Changes in corticomotor representations induced by prolonged peripheral nerve stimulation in humans. Clinical Neurophysiology, 112: (8), 1461–1469. |
31 | Rossini P.M. , Burke D. , Chen R. , Cohen L.G. , Daskalakis Z. , Di Iorio R. , & ... Ziemann U. ((2015) ) Non-invasive electrical and magnetic stimulation of the brain, spinal cord, roots and peripheral nerves: Basic principles and procedures for routine clinical and research application. an updated report from an I.F.C.N. committee. Clinical Neurophysiology, 126: (6), 1071–1107. doi: 10.1016/j.clinph.2015.02.001 |
32 | Roy F.D. , & Gorassini M.A. ((2008) ) Peripheral sensory activation of cortical circuits in the leg motor cortex of man. The Journal of Physiology, 586: (Pt 17), 4091–4105. doi: 10.1113/jphysiol.2008.153726 |
33 | Rushton D.N. ((2003) ) Functional electrical stimulation and rehabilitation–an hypothesis. Med Eng Phys, 25: (1), 75–78. |
34 | Russmann H. , Lamy J.C. , Shamim E.A. , Meunier S. , & Hallett M. ((2009) ) Associative plasticity in intracortical inhibitory circuits in human motor cortex. Clinical Neurophysiology: Official Journal of the International Federation of Clinical Neurophysiology, 120: (6), 1204–1212. doi: 10.1016/j.clinph.2009.04.005 |
35 | Sale M.V. , Ridding M.C. , & Nordstrom M.A. ((2007) ) Factors influencing the magnitude and reproducibility of corticomotor excitability changes induced by paired associative stimulation. Experimental Brain Research, 181: (4), 615–626. doi: 10.1007/s00221-007-0960 |
36 | Stefan K. , Kunesch E. , Benecke R. , Cohen L.G. , & Classen J. ((2002) ) Mechanisms of enhancement of human motor cortex excitability induced by interventional paired associative stimulation. The Journal of Physiology, 543: (Pt 2), 699–708. doi: PHY_023317 |
37 | Stefan K. , Kunesch E. , Cohen L.G. , Benecke R. , & Classen J. ((2000) ) Induction of plasticity in the human motor cortex by paired associative stimulation. Brain: A Journal of Neurology, 123: (Pt 3), 572–584. |
38 | Stefan K. , Wycislo M. , & Classen J. ((2004) ) Modulation of associative human motor cortical plasticity by attention. Journal of Neurophysiology, 92: (1), 66–72. doi: 10.1152/jn.00383.2003 |
39 | Sung C.Y. , & Chu N.S. ((1989) ) Epileptic seizures in intracerebral haemorrhage. Journal of Neurology, Neurosurgery, and Psychiatry, 52: (11), 1273–1276. |
40 | Takatsuru Y. , Fukumoto D. , Yoshitomo M. , Nemoto T. , Tsukada H. , & Nabekura J. ((2009) ) Neuronal circuit remodeling in the contralateral cortical hemisphere during functional recovery from cerebral infarction. The Journal of Neuroscience: The Official Journal of the Society for Neuroscience, 29: (32), 10081–10086. doi: 10.1523/JNEUROSCI.1638-09.2009 |
41 | Taub E. , Crago J.E. , Burgio L.D. , Groomes T.E. , Cook E.W. , DeLuca S.C. 3rd , & Miller N.E. ((1994) ) An operant approach to rehabilitation medicine: Overcoming learned nonuse by shaping. Journal of the Experimental Analysis of Behavior, 61: (2), 281–293. doi: 10.1901/jeab.1994.61-281 |
42 | Tokimura H. , Di Lazzaro V. , Tokimura Y. , Oliviero A. , Profice P. , Insola A. , & Rothwell J.C. ((2000) ) Short latency inhibition of human hand motor cortex by somatosensory input from the hand. The Journal of Physiology, 523: (Pt 2), 503–513. doi: PHY_9995 |
43 | Traversa R. , Cicinelli P. , Pasqualetti P. , Filippi M. , & Rossini P.M. ((1998) ) Follow-up of interhemispheric differences of motor evoked potentials from the ‘affected’ and ‘unaffected’ hemispheres in human stroke. Brain Research, 803: (1-2), 1–8. doi: 10.1016/S0006-8993(98)00505-8 |
44 | Ueki Y. , Mima T. , Kotb M.A. , Sawada H. , Saiki H. , Ikeda A. , & ... Fukuyama H. ((2006) ) Altered plasticity of the human motor cortex in parkinson’s disease. Annals of Neurology, 59: (1), 60–71. doi: 10.1002/ana.20692 |
45 | Ward N.S. , & Cohen L.G. ((2004) ) Mechanisms underlying recovery of motor function after stroke. Archives of Neurology, 61: (12) doi: 10.1001/archneur.61.12.1844 |
46 | Ziemann U. , Rothwell J.C. , & Ridding M.C. ((1996) ) Interaction between intracortical inhibition and facilitation in human motor cortex. The Journal of Physiology, 496: (Pt 3), 873–881. |