Visuo-motor integration in unresponsive wakefulness syndrome: A piece of the puzzle towards consciousness detection?
Abstract
Purpose: The unresponsive wakefulness syndrome (UWS) is characterized by either a profound unawareness or an impairment of large-scale cortico/subcortical connectivity. Nevertheless, some individuals with UWS could show residual markers of consciousness and cognition. In this study, we applied an electrophysiological approach aimed to identify the residual visuomotor connectivity patterns that are thought to be linked to awareness, in patients with chronic disorder of consciousness (DOC).
Methods: We measured some markers of visuomotor and premotor-motor integration in 14 patients affected by DOC, before and after the application of transcranial direct current stimulation, delivered over the dorsolateral prefrontal cortex and the parieto-occipital area, paired to transorbital alterning current stimulation.
Results: Our protocol induced a potentiation of the electrophysiological markers of visuomotor and premotor-motor connectivity, paired to a clinical improvement, in all of the patients with minimally conscious state and in one individual affected by UWS.
Conclusions: Our protocol could be a promising approach to potentiate the functional connectivity within large-scale visuomotor networks, thus allowing identifying the patients suffering from a functional locked-in syndrome (i.e. individuals showing an extreme behavioral motor dysfunction although with somehow preserved cognitive functions that can be identified only through para-clinical tests) within individuals with UWS.
1Introduction
The clinical detection of awareness signs, including purposeful behavioral responsiveness to stimuli, has a pivotal role in the differential diagnosis of chronic disorders of consciousness (DOC). In fact, the lack of awareness characterizes the unresponsive wakefulness syndrome (UWS) (previously named vegetative state - VS), whereas patients with minimally conscious state (MCS) show “inconsistent but clearly discernible behavioral evidence of consciousness” (Giacino et al., 2002; Laureys et al., 2010a). Notably, awareness impairment and, thus, the limitation of behavioral responsiveness are proportionally related to the level of connectivity disruption within a wide cortical/subcortical neuromatrix that supports consciousness generation and maintenance, besides the arousal system (Laureys et al., 2010b). Nevertheless, the patients suffering from a functional locked-in syndrome (fLIS) show an extreme behavioral motor dysfunction although with a partial preservation of higher cognitive functions and cerebral connectivity, as advanced neurophysiological and neuroimaging approaches have shown (Bruno et al., 2011). Such condition may arise from lesions at one or more levels of the sensory-motor system. On the other hand, patients with classic (i.e., quadriplegia and anartria, with eye-coded communication) and total LIS (i.e., a rare syndrome characterized by complete immobility including eye movements) typically suffer from a brainstem injury and show very limited signs of awareness due to profound sensory and motor deficits, although with preserved self-awareness and cognitive capacities, besides a normal brain network connectivity (Haig et al., 1987; Laureys et al., 2005). Indeed, it is extremely difficult to properly assess awareness in such patients, since either their movements may be minimal or inconsistent, or no cognitive output could be possible (Giacino and Zasler, 1995). In fact, fLIS, total LIS, and patients suffering from UWS are behaviorally indistinguishable, and it is possible to reach the differential diagnosis only through para-clinical approaches, including functional neuroimaging and advanced neurophysiological paradigms (Bruno et al., 2011).
Hence, a covert cognition and a residual complex cerebral connectivity characterize patients affected by fLIS and total LIS, whereas individuals with UWS lack of such markers. Nevertheless, the study of a sensory-motor system that is unable to contribute to the generation of purposeful behaviors, is challenging in patients with DOC, and thus the misdiagnosis rate is still high (Bekinschtein and Manes, 2008).
To this end, the study of large-scale sensory-motor integration processes supporting awareness may be useful in an attempt to differentiate patients with fLIS (Kotchoubey et al., 2013). In particular, the visuomotor integration (VMI) assessment seems a promising approach, as suggested by its clinical usefulness in terms of differential diagnosis and prognosis (Troiano et al., 2012; Hildebrandt et al., 2007). Moreover, VMI enrolls different and complex cortical-subcortical networks that are related to visual processes at conscious level (Grossberg, 2003; Humphreys et al., 1997). On the other hand, the first-level VMI processes (i.e. the paired activation of primary motor and primary visual cortices) do not correlate with awareness preservation (reflecting instead alerting functions, motor attention and preparation) (Katsuki and Constantinidis, 2012; Monti et al., 2013).
Several works have shown the possibility to bring to light the above mentioned complex integrative processes by means of non-invasive neurostimulation techniques, including the repetitive transcranial magnetic stimulation (rTMS) and the transcranial direct current stimulation (tDCS). Such approaches could boost neural plasticity by means of long-term potentiation or depression-like mechanisms (LTP or LTD) (Ziemann et al., 2008) within the primary motor cortex (M1) (Rioult-Pedotti et al., 2000; Sanes and Donoghue, 2000), the sensory-motor areas (Stefan et al., 2000; Wolters et al., 2003), and the pain-matrix (Garcia-Larrea and Peyron, 2013; Suppa et al., 2013; Naro et al., 2015b). In addition, such paradigms do not necessarily require a substantial patient’s cooperation. Interestingly, the assessment and the modulation of VMI through non-invasive neuromodulation could be helpful in the differential diagnosis of UWS and fLIS. Indeed, we may argue that patients with UWS who show an improvement of the cortical functional connectivity and the visuomotor output after a proper neuromodulation approach should be no longer considered as UWS, but fLIS. To this end, we assessed the large-scale visuo-premotor-motor functional connectivity in a clinically defined UWS sample and in a control group of MCS and healthy subjects (HC), before and after the administration of a paired associative stimulation protocol consisting of tDCS over dorsolateral prefrontal cortex (DLPFC) and parieto-occipital areas (POA), and trans-orbital alterning current stimulation (tACS). More in detail, we measured some parameters of cortical excitability and connectivity, by means of single- and dual-site TMS, and the visual-stimuli event-related potentials. Since a standard visual pathway stimulation is extremely challenging in patients with DOC, owing to the low and inconsistent cooperation, we chose a tACS approach capable to evoke visual potentials regardless patient’s cooperation. We stimulated the DLPFC and the POA by means of the dual-site tDCS, because of their important role in the stimulus-driven (bottom-up) and the purpose-driven (top-down) neural processes (Grossberg, 2003; Humphreys et al., 1997). More in detail, the POA’s intrinsic circuits constitute the common origin of three distinct pathways: i) the parieto-prefrontal, involved in top-down control of eye movements and in spatial working memory; ii) the parieto-premotor, mediating ocular, reaching, and grasping movements; and iii) the parieto-temporal, underlying the complex spatial processing required for navigating through the environment.
Since voluntary eye-movements are an important clinical marker of the level of awareness, we chose such double-site tDCS approach.
We hypothesized that our experimental protocol could improve the motor area excitability, the visuo-prefrontal functional connectivity, and the visuomotor output, allowing bringing to light covert signs ofawareness in patients who were clinically defined UWS.
2Methods
2.1Subjects
Of the 32 subjects suffering from chronic DOC attending the Neurorehabilitative Unit of the IRCCS Centro Neurolesi “Bonino-Pulejo” (Messina, Italy), we enrolled 14 patients who met the criteria for VS/MCS diagnosis (The Multi-Society Task Force on PVS, 1994; Giacino et al., 2002), and the following exclusion criteria: a DOC condition lasting less than 3 months after the brain injury; other severe neurological or systemic diseases; critical conditions (i.e. inability to breathe independently, hemodynamic instability); cortical excitability-modifying drugs assumption beyond L-DOPA and baclofen; presence of epileptic history, pace-maker, aneurysms clips, neurostimulator, brain/subdural electrodes or other electromechanical devices; presence of electroencephalographic (EEG) suppression-burst pattern; absence of visual evoked potential (VEP). In addition, we included in the study 7 HC (4 females and 3 males, mean age 55.3 ± 5.8 years) as control group.
We reported the clinic-demographic characteristics in Table 1. DOC etiology consisted of a post-anoxic or a post-traumatic brain damage.The neurological examination mainly showed a pattern of spastic tetraparesis. EEG examination evidenced a continuous slowing in theta and/or delta frequency ranges. Our Research Institute Ethics Committee approved the present study and either the HC or the legal guardian of each patient gave their written informed consent.
2.2Clinical assessment
Two neurologists skilled in DOC diagnosis independently evaluated the patients through the JFK CRS-R. This scale is a reliable and standardized tool, which integrates neuropsychological and clinical assessment, and includes the current diagnostic criteria for coma, VS, and MCS, allowing the clinician to assign the patient to the most appropriate diagnostic category. Hence, the CRS-R represents an appropriate approach for characterizing the level of consciousness and for monitoring the neurobehavioral function recovery (Gerrard et al., 2014). The CRS-R was daily administered for 30 consecutive days, at different times, in order to steadily establish the level of consciousness impairment.
2.3Conditioning protocols
Each participant underwent four different protocols:i) a real (real_tDCS+real_tACS); ii) a sham (sham_tDCS+sham_tACS); iii) a tDCS_alone (real_tDCS +sham_tACS); and iv) a tACS_alone (real_tACS+sham_tDCS). We administered the protocols in a random scheme (i, ii, iii, and iv) and in different sessions, at one-day of interval. Participants and experimenters who analyzed data were blinded on scheme procedure.
For the real_tDCS, we used a battery-driven stimulator (Brain Stim, E.M.S., Bologna, Italy) with a couple of conductive-rubber electrodes, placed in saline-soaked sponges (active electrode 5×5 cm) over F3 (DLPFC) and PO5 (POA) (according to the 10/20 International System). The current stimulation ramped up/down during the first/last 30 sec of stimulation, at 1 mA of intensity. Current density was always below the safety limit of 52 μA/cm2. The device kept the impedance below 10 kΩ (Miranda et al., 2006; Bikson et al., 2010; Nitsche et al., 2003).
For the real_tACS, we extra-ocularly applied 4 electrodes (sintered Ag/AgCl ring electrodes) to both eyelids of the left eye (while the eye was kept closed), cabled to a battery-driven stimulator (BrainStim, E.M.S., Bologna, Italy) (Sabel et al., 2011). FPz served as reference electrode. At first, we determined the phosphene threshold in our HC sample, applying bursts of 15 pulses (10 ± 2 ms at 20 ± 5 Hz; the values ranged randomly in order to avoid habituation phenomena) and increasing the current intensity by steps of 10 μA per second (starting from zero). We set the stimulation frequency in HC, increasing the frequency by steps of 1–5 Hz per second, from the α-range (the minimum stimulation frequency applicable) to the flicker-fusion frequency (i.e. the maximal temporal resolution frequency at which the intermittent tACS stimulus appeared as steady to the average of the HC), at an intensity of ≤0.8 mA.The mean values of these parameters were applied in each participant affected by DOC.
Concerning the real_protocol, we delivered 150 visual stimuli during each tDCS protocol, at the same intensity used in VEP elicitation, at 0.25 Hz (i.e., 300 stimuli in 20 min). For the sham_tDCS, we switched-off the stimulator after 30 sec, whereas the sham_tACS consisted of 50 stimuli (i.e. at 0.08 Hz).
2.4Single-pulse, paired-pulse and dual-site TMS measures
We used a high-power Magstim2002 Stimulator (Magstim, Whitland, Dyfed, UK) in order to elicit the motor evoked potential (MEP) through magnetic monophasic stimuli. We held the coil tangentially to the scalp with the handle pointing backwards and laterally, at a 45° angle from the sagittal plane, approximately perpendicular to the central sulcus of the left hemisphere, on the optimal scalp site to get the wider MEP amplitude from the relaxed right abductor pollicis brevis muscle (APB) (motor hot-spot). The rise time of the magnetic monophasic stimulus was ∼100 μs with a to-zero of ∼800 μs. The current flowed in handle direction during the rise-time of the magnetic field, thus with a posterior-anterior direction. We preliminarily evaluated the resting motor threshold (RMT), defined as the smallest magnetic stimulus intensity able to evoke a peak-to-peak MEP of 50 uV in resting right APB, in at least five-out-ten consecutive tracks (Rossini et al., 1994). Then, we applied an intensity of stimulation of 120% of RMT (test stimulus). We applied Ag-AgCl surface electrodes to the right APB, using a classic muscle belly-tendon montage, for recording EMG activity. A Digitimer D360 Amplifier (Digitimer Ltd., Welwyn Garden City, Herts, UK) amplified, filtered (32 Hz-1 KHz), and stored the signals (at 10KHz on a personal computer for off-line analysis) (Signal Software, Cambridge Electronic Design, Cambridge, UK). During the experiments, we continuously monitored the EMG activity through visual (oscilloscope) and auditory (speakers) feedback, to ensure the complete muscle relaxation.
Concerning the short-latency intracortical facilitation (SICF), we delivered two juxta-RMT stimuli (being the former the conditioning stimulus, the latter the test one) at an interstimulus interval of 2.5 ms (Tokimura et al., 1996), whereas we applied two supra-threshold stimuli (at 120% RMT) at an interstimulus interval of 150 ms for the long-latency intracortical inhibition (LICI) (Valls-Sole et al., 1992). We measured the mean amplitude of the conditioned MEP as percentage of the amplitude of the unconditioned MEP (test), which was taken as a measure of cortical excitability. We registered 15 unconditioned MEPs, 10 LICI, and 10 SICF, randomly intermingled in a single trial, at a frequency of 0.25 Hz (test duration ∼9 min). All data are given as mean or percentage difference in comparison to baseline values ± standard error (se).
Concerning premotor-motor interaction, we measured the effects on MEP amplitude of a dual-site TMS approach (using two figure-of-eight monophasic coils) on PMdright-M1left and PMvleft-M1left (interstimulus interval of 7 ms, conditioning stimulus at 70% RMT on the premotor area, test stimulus over M1 at 120% RMT), SMA-M1left (CS at an interstimulus interval of 6 ms, conditioning stimulus at 3 cm anteriorly to Cz -preSMA- at 70% RMT, test stimulus over M1 at 120% RMT), and PPCleft-M1left (conditioning stimulus at an interstimulus interval of 10 ms, at 90% RMT, test stimulus over M1 at 120% RMT) (Civardi et al., 2001; Koch et al., 2007a,b; Davare et al., 2008). We registered10 conditioned MEPs for each premotor-motor interaction in different trials, intermingled with 10 unconditioned MEPs, delivered at a frequency of 0.25 Hz (test duration ∼12 min). We measured the mean amplitude of the conditioned MEP as percentage of the amplitude of the unconditioned (test) MEP, which was taken as a measure of premotor-motor excitability. All data are given as mean or percentage difference in comparison to baseline values ± se.
2.5VEP, VMI, and ERP
Since a standard VEP assessment in patients with DOC is extremely challenging owing to the low and inconsistent cooperation, we chose a tACS approach in order to elicit VEP (Gall et al., 2010, 2011). We delivered 100 visual stimuli over left eye, while kept closed, with the same characteristics used for tACS, at a frequency of 1 Hz (test duration ∼1.6 min). We recorded the EEG activity from 3 scalp sites, using Ag/AgCl electrodes positioned over the mid-occipital (MO, 5 cm above nasion), left lateral occipital (LLO, 5 cm lateral to MO), and left temporal area (LT, 5 cm lateral to LLO) (Suppa et al., 2015). An electrode over the mid-frontal position (12 cm above nasion) served as reference, whereas one over right mastoid as ground. Impedance was ≤5 kΩ. We amplified and acquired the signals at 5 kHz through a 1401plus AD laboratory interface (Cambridge Electronic Design, Cambridge, UK), registered and filtered (0.5–70 Hz+50 Hz notch) through a Digitimer D360 (Digitimer Ltd, Welwyn Garden City, UK), and stored on a personal computer for off-line analysis (Signal software; Cambridge Electronic Design, UK). We registered a biphasic evoked component (N75-P100) from MO in HC, which was similar to standard pattern-reversal VEP.
In a different VEP session, we applied a tACS protocol in analogy to an odd-ball paradigm (Machado et al., 2014), in which we randomly shifted the stimulation frequency and the intensity of ± 25% in an 80:20 frequent:infrequent ratio. Each participant underwent a block of 500 trials (test duration ∼8 min), in which there was a 95% chance of 1–4 infrequent stimuli preceding a frequent one and a 5% chance of 5–7 infrequent preceding a frequent one. Hence, we were able to register an ERP from LLO and LT regardless participant’s cooperation. For ERP analysis, we filtered at 0.3/25 Hz+notch (50 Hz), epoched (ranging from −100 ms by visual stimulus onset to 600 ms after) after visual and independent component analysis inspection for artifact removal, and averaged EEG signals for each channel. Then, we measured the amplitude of the registered ERP.
We assessed the VMI by means of paired visual stimuli (conditioning stimulus through left-eye tACS) and MEP (test stimulus by means of single-pulse TMS over M1left). We chose two individually-adapted interstimulus intervals that were clearly linked to inhibitory (VEP latency +40 ms) or facilitatory (VEP latency +100 ms) effects on MEP amplitude in healthy individuals (Suppa et al., 2015). We registered 15 unconditioned MEP intermingled with 15 VEP-MEP interactions for each interstimulus intervals in a single trial, delivered at a frequency of 0.2 Hz (test duration ∼9 min). We measured the mean amplitude of the conditioned MEP as percentage of the amplitude of the unconditioned (test) MEP, which was taken as a measure of VMI strength. All the data are given as mean or percentage difference in comparison to baselinevalues ± se.
2.6Study design
HC sat in a comfortable armchair in a darkened room, whereas we carried-out the patients’ experimental procedure at bedside, in a darkened environment. HC and patients had their right eye covered by an eye-patch. We applied a real electrophysiological protocol, a sham, a tDCS_alone, and a tACS_alone protocol as control experiments, in each group. The protocols were delivered at one-day of interval, in a random delivery scheme (i, ii, iii, and iv). Before (TPRE) and after the end of each conditioning protocol (immediately, T0, 30-min, T30, and 60-min, T60), we assessed the peak-to-peak MEP amplitude from the left M1, the intracortical circuit excitability assessed through paired-pulse TMS over the left M1 (SICF and LICI), the premotor-motor interactions (PPC-M1, SMA-M1, PMd-M1, PMv-M1), and the VMI (combining tACS and TMS pulses over M1 at specific interstimulus intervals). Moreover, we measured the VEP and ERP parameters elicited by tACS. We individually adapted each site of TMS/tDCS stimulation and recording according to a recently performed brain MRI of each participant. We assessed the clinical effects of our protocols in patients with DOC through the JFK CRS-R. Figure 1 summarizes the experimental design.
2.7Data acquisition and statistical analysis
We performed the baseline clinical and electrophysiological comparisons through unpaired t-tests. We evaluated the effects of the conditioning protocols on each dependent variable (visuomotor CRS-R, RMT, MEP, LICI, SICF, PMd-M1, PMv-M1, SMA-M1, PPC-M1, VMI40 ms, VMI100 ms, VEP, ERPLT, and ERPLLO) in separated three-way repeated-measure analyses of variance (rmANOVAs), implying time (four levels: TPRE, T0, T30, and T60) and protocol (four levels: real_protocol, sham_protocol, tDCS_alone, and tACS_alone) as within-subject factors, and group (three levels: MCS, UWS, and HC) as between-subject factor. The Greenhouse-Geisser method was used if necessary to correct for non-sphericity. Conditional on a significant F-value, we performed post-hoc t-tests (Bonferroni) to explore the strength of main effects and the patterns of interaction between the experimental factors. All statistical tests were applied two-tailed. A significant p-value was <0.05. All data are given as means or percent changes ± se. We calculated a Fisher Z-transformation in order to assess an eventual correlation among clinical, demographic, and electrophysiological parameters.
3Results
We did not observe any effects in patients andHC, either during or after the entire experimentalprocedure.
3.1DOC/HC clinical and electrophysiological differences at baseline
We showed the baseline CRS-R total scores in Table 1 (MCS-UWS comparison p = 0.003). There were no differences concerning either DOC etiology or the demographic characteristics.We resumed in Table 2 the baseline DOC and HC electrophysiological differences. RMT, MEP amplitude, VEP amplitude and latency were similar in the three groups, whereas paired-pulse TMS, ERP, and VMI measures were significantly different concerning either DOC-HC (p < 0.05 for each MCS-HC and UWS-HC comparison, by means of unpaired t-tests) or MCS-UWS comparison (Table 2). Indeed, the patients suffering from DOC showed an increased facilitatory (SICF) and a strongly reduced inhibitory tone (SICI), a clear alteration of cortico-cortical interactions, with a prevalence of facilitatory connections, increased latency and reduced amplitudes of VEPs and visual-ERPs (although HC showed ERPs from both LLO and LT electrodes, and the MCS only from LLO electrodes, whereas UWS did not show any ERP). Each parameter was more impaired in MCS than UWS.
3.2Conditioning protocol’s effects on clinical assessment
Although rmANOVA did not show any significant timeXgroupXprotocol interaction, we observed an increase of one point at the CRS-R visuomotor sub-item at T0 in the two patients with MCS (n. 4 and 5) and in one UWS (n. 3), only after the real_protocol. Indeed, such patients with MCS upgraded from “fixation” (2 points at the CRS-R visuomotor sub-item) to “following with eyes an object” (3 points), whereas the UWS from “visual startle” (1 point) to “visual fixation” (2 points). Such effects were short-lasting, since they were not detectable at either T30 or T60.
3.3Conditioning protocol electrophysiological effects
We summarized the statistical analysis data in Table 3. RMT, LICI, ERPLLO and ERPLT latency, PMv-M1, and VEP latency and amplitude did not significantly vary after each conditioning protocol. Concerning MEP, ERPLLO amplitude, PMd-M1, and SICF, we observed a significant amplitude increase at T0 and T30 in HC (the electrophysiological values reverted at T60 at the baseline ones), and in MCS at T0 (the electrophysiological values reverted at T30 and T60 at baseline ones), only after the real_protocol, whereas none of the patients with UWS, but one (n.3), showed such effects (Fig. 2). PPC-M1, VMI40ms, and VMI100ms after-effects following real_protocol were particularly evident either in HC (up to T30) or in MCS (at T0), but not in UWS, with the exception of the patient n. 3. The tDCS_alone protocol induced after-effects that were similar to real_protocol, although non-significant and limited to MEP amplitude increase and PPC-M1 facilitation. Moreover, there were no effects to be referred to visuomotor processes. Either the tACS_alone or the sham_protocol were totally ineffective. Figure 2 shows the percentual changes of the electrophysiological parameters. Concerning the UWS subject n. 3, we found MCS-like real_protocol after-effects at T0, which were ∼14% lower in strength than the mean values of patients with MCS. Moreover, we identified a small ERPLLO only at T0.
3.4Correlations
We assessed the correlations between the amounts of electrophysiological TPRE/T0 changes in HC group, in an attempt to better understand the direction of the effects induced by the real_protocol. Interestingly, the VMI% increase was related to the PMd-M1% (Z = 3.5, p = 0.001) and the SMA-M1% increase (Z = 2, p = 0.04). Moreover, we observed a correlation between the PPC-M1 facilitation and the ERPLT-amplitude increase (Z = 3, p = 0.006).
4Discussion
Recent neurophysiological and functional neuroimaging studies have suggested that the lack of behavioral responsiveness in patients affected by DOC does not necessarily imply unawareness, since a patient could not show purposeful behavior owing to motor-output or cognitive deterioration rather than connectivity impairment (Bruno et al., 2011; Formisano et al., 2013). Interestingly, some residual patterns of cortical connectivity within visuomotor areas have been previously shown in some individuals with UWS (Owen et al., 2006; Monti et al., 2010, 2013).
For the first time ever, we assessed the presence of residual large-scale visuomotor functional connectivity in a DOC sample by means of an electrophysiological approach consisting of paired tDCS and tACS. Only the real_protocol induced a potentiation of the M1 excitability (MEP amplitude and SICF increase), the premotor-motor connectivity (increased facilitatory and decreased inhibitory connections), and the visual basic cognitive process (ERPLT increase in HC, and ERPLLO amplitude increase in HC, in patients with MCS, and in one subject affected by UWS). In addition, such after-effects were strongly inter-correlated (VMI and PMd-M1, VMI and SMA-M1, PPC-M1 and ERPLT amplitude) and paralleled by a transient visuomotor CRS-R score improvement in some patients (MCS n. 4 and 5; UWS n. 3). In detail, some patients moved from the “perceptual awareness” (i.e. the potential to perceive the external world and to interact with it, expressed at least by the visual startle at the CRS-R) to a transient “visual consciousness” (at least visual fixation at the CRS-R). Nevertheless, since both some patients with UWS and MCS showed a stable visual fixation at the CRS-R (a score of 2 at visuomotor domain), the visual fixation could not be per se considered as a marker of MCS, as also suggested by functional neuroimaging studies (e.g. Bruno et al., 2010). On the other hand, we may argue that even UWS could have a residual potential to interact with external word through VMI processes, as also suggested by other studies concerning different sensory modalities, including pain processing (de Tommaso et al., 2015). Notably, a “general visual consciousness” (the highest grade of the VMI process that also enroll other extra-visual circuitries) needs more functional and structured visuomotor networks (O’Regan and Noë, 2011), which were not assessed by our protocol.
Hence, the after-effects of our real_protocol may suggest the possibility of a visuomotor output facilitation leading to a higher conscious level, even in some patients affected by UWS. This finding may be due to a potentiation of the residual premotor-motor, visuomotor, and visuo-cognitive functions,thus suggesting a diagnosis of fLIS. Indeed, the preserved connectivity and the covert awareness may subtend a covert partial consciousness even in some patients with UWS (i.e. fLIS), as previously hypothesized (Laureys et al., 2010; Giacino and Zasler, 1995; Bekinschtein and Manes, 2008; Monti et al., 2013; Perrin et al., 2006). Classic and total LIS are instead characterized by nearly-normal brain connectivity.
4.1tDCS-tACS physiology
A recent VEP-rTMS protocol induced a LTP/LTD-like plasticity within visuomotor areas (Suppa et al., 2015), probably by means of a topographically specific spike-timing dependent plasticity (STDP) within M1. Moreover, several studies have shown that tDCS may also induce cortical polarity changes within M1in a LTP-likewise (involving voltage-gated sodium channels and glutamatergic NMDA receptors) (Rizzo et al., 2014; Liebetanz et al., 2002; Nitsche et al., 2005; Ridding et al., 2000; Ridding and Uy, 2003; Kaelin-Lang et al., 2002; Naro et al., 2015a). Thus, our paradigm could have induced a LTP-like potentiation by means of hetero-synaptic STDP mechanisms within M1 (involving visual -tACS- and motor -TMS- inputs). The induction of a STDP mechanism is further corroborated by the lack of substantial effects following either the tDCS_alone, or the tACS_alone, or the sham_protocol. The after-effects we observed seem therefore to depend on the combination of the tDCS and tACS protocols.
Concerning the neural pathways supporting our after-effects, several studies have proposed that primary visual cortices could firstly elaborate the visuo-spatial information to generate visual perception and rapid-onset response (in analogy to other sensory-motor integration processes) (Keliris et al., 2010; Leopold and Logothetis, 1996; Saron et al., 2001; Tokimura et al., 2000; Valeriani et al., 1999; Sowman et al., 2014). Then, visual information may flow through extra-striate, temporal, parieto-occipital, DLPFC, premotor, and subcortical regions (maybe cerebellum and basal ganglia), in order to generate spatial localization processing, oculo-motor control, visual attention, motor planning and execution, and visually-guided motor command (Kravitz et al., 2011).The activity within the frontal, temporal, and parieto-occipital areas may oversee the conscious contents of visual information, whereas premotor and motor areas may organize conscious motor planning (Dolce et al., 2011). Hence, we may hypothesize that our protocol enrolled both dorsal and ventral visual pathways (Goodale and Milner, 1992), mainly involving the PPC and SMA (that have an updating effect of on visual feedback-based, active motor planning, motor attention, and motor intentions) (Desmurget and Grafton, 2000; Jeannerod et al., 1994; Ikkai and Curtis, 2011). In addition, we cannot exclude a possible role of the contralateral homologous areas and transcallosal connections, since we limited our measurements to the left hemisphere (Koch et al., 2009; Suppa et al., 2015).
Notably, we have to acknowledge other issues concerning the physiological effects of our combined real_protocol: i) meta-plasticity phenomena could play an important role in supporting and regulating our after-effects. Indeed, the sequential application of two tDCS protocols may have involved either homeostatic or non-homeostatic meta-plasticity mechanisms (Bienenstock et al., 1982; Davis, 2006), suggesting the preservation of stabilizing plasticity phenomena even in individuals with UWS; ii) Suppa et al. (2015) applied a pattern-reversal visual stimulation protocol (paired to rTMS) in an attempt to activate the left temporal hemi-retina and focally trigger brain networks. We instead stimulated the entire left retina, and a double tDCS protocol was applied. Hence, we triggered brain networks with lower topographic specificity than the VEP-rTMS protocol; iii) since RMT, VEP and MEP parameters were not different at baseline between MCS and UWS, and RMT and VEP did not vary after the conditioning protocols, we can exclude the possibility that baseline cortical excitability or VEP differences could have influenced the after-effects. Moreover, in reason of the blinded condition of participants concerning the different experimental sessions, we may exclude differences in the attentive level in the HC participants (Stefan et al., 2004).
4.2tDCS-tACS after-effects in patients with DOC
The potentiation of the M1 excitability, the premotor-motor connectivity, the visuo-cognitive function, and the visuomotor output in our patients may suggest the induction of VMI processes through time-locked neural activities that encompass premotor and parieto-occipital networks (spreading from the posterior to the anterior cortical areas and vice versa) and other subcortical areas (maybe including thalamus and basal ganglia) (Monti et al., 2013; Suppa et al., 2015; Thiebaut de Schotten et al., 2014; Bruno et al., 2010; Petrides and Pandya, 2006; Di et al., 2014; Dum and Strick, 2005; O’Shea et al., 2007; Buch et al., 2010). Interestingly, it has been shown that visual pursuits depend on the activity of the mesiofrontal-precuneal cortex, which represents an important hub within the neural correlates of consciousness and is clearly impaired in patients suffering from severe chronic DOC (Bruno et al., 2010). The re-appearance or the enhancement of such visuomotor responses could therefore express a functional upgrading -although transient - of the residual cortico-cortical and the brainstem-thalamo-cortical networks supporting VMI processes (Dolce et al., 2011; Bruno et al., 2010; Di et al., 2014; Riganello and Sannita, 2009).
It has been hypothesized that plasticity and connectivity recovery in individuals suffering from DOC might depend on the modulation of post-ischemic LTP (Crepelet al., 1993; Di Filippo et al., 2008), the production of specific neurotrophins (e.g. Brain-Derived Neurotrophic Factor; Kokaiaet al., 1998), and the regulation of excitatory/inhibitory dynamics within cortical and thalamo-cortical circuits (Dinget al., 2011). Thereby, it is conceivable that one or more of these mechanisms may have been triggered by the real_protocol, and could have favored the recruitment of silent or stunned cortico-cortical and cortico-subcortical connections, maybe involving the Schiff’s mesocircuit model (Schiff, 2010).
Moreover, the bottom-up (stimulus salience) and top-down attention mechanisms (spatial and feature-driven attention, task goals) following the interaction between the ventral and the dorsal visual streams could have an important role in the clinical after-effects (Corbetta and Shulman, 2002; Currasco, 2011; Block, 2011; Pinto et al., 2013). Nevertheless, such issue needs to be further investigated by means of proper visuomotor tasks.
4.3Study limitations, conclusions and future perspectives
In our opinion, our study proposes a promising approach in an attempt to identify residual patterns of VMI and large-scale fronto-parietal connectivity in patients affected by severe DOC, including UWS.
Noteworthy, the small sample size and the consequent mixed etiology represent a main limiting factor in our study. Nonetheless, it is difficult to study a large sample of patients with DOC, since the negative outcome of such patients is still unfortunately high.
However, our data further support the importance of patient’s cooperation independent diagnostic approaches, aimed at assessing the consciousness level and differentiating real UWS from the patients that are clinically unable to express awareness signs (Formisano et al., 2013; Bruno et al., 2011; Schnakers et al., 2014; Naro et al., 2014). In addition, the possibility to identify such partially preserved cortico-cortical and cortico-subcortical networks in DOC may be useful in the selection of candidate patients for the deep brain stimulation (Schiff et al., 2007) or therapeutic and rehabilitative trials by means of non-invasive neurostimulation approaches.
REFERENCE
1 | Bäumer T, Schippling S, Kroeger J, Zittel S, Koch G, Thomalla G, Rothwell JC, Siebner HR, Orth M, Münchau A(2009) Inhibitory and facilitatory connectivityfrom ventral premotor to primary motor cortex in healthy humans atrest—a bifocal TMS studyClin Neurophysiol120: 917241731 |
2 | Bekinschtein T, Manes F(2008) Evaluating brain function in patients with disorders of consciousnessCleve Clin J Med75S2: 7176 |
3 | Bienenstock EL, Cooper LN, Munro PW(1982) Theory forthe development of neuron selectivity, orientation specificity andbinocular interaction in visual cortexJ Neurosci2: 13248 |
4 | Bikson M, Datta A, Rahman A, Scaturro J(2010) Electrode montages for tDCS and weak transcranial electrical stimulation, role of “return” electrode’s position and sizeClin Neurophysiol121: 1219761978 |
5 | Block N(2011) Perceptual consciousness overflows cognitive accessTrends Cogn Sci15: 12567575 |
6 | Bruno MA, Laureys S, Demertzi A(2013) Coma and disorders of consciousnessHand Clin Neurol118: 205213 |
7 | Bruno MA, Vanhaudenhuyse A, Schnakers C, Boly M, Gosseries O, Demertzi A, Majerus S, Moonen G, Hustinx R, Laureys S(2010) Visual fixation in the vegetative state, an observational case series PET studyBMC Neurol10: 35 |
8 | Bruno MA, Vanhaudenhuyse A, Thibaut A, Moonen G, Laureys S(2011) From unresponsive wakefulness to minimally conscious PLUS and functional locked-in syndromes, recent advances in our understanding of disorders of consciousnessJ Neurol258: 713731784 |
9 | Buch ER, Mars RB, Boorman ED, Rushworth MF(2010) A network centered on ventral premotor cortex exerts both facilitatory and inhibitory control over primary motor cortex during action reprogrammingJ Neurosci30: 413951401 |
10 | Carrasco M(2011) Visual attention: The past yearsVis Res51: 1314841525 |
11 | Civardi C, Cantello R, Asselman P, Rothwell JC(2001) Transcranial magnetic stimulation can be used to test connections to primary motor areas from frontal and medial cortex in humansNeuroimage14: 614441453 |
12 | Corbetta M, Shulman G(2002) Control of goal-directed and stimulus-driven attention in the brainNat Rev Neurosci3: 3201215 |
13 | Crepel V, Hammond C, Chinestra P, Diabira D, Ben-Ari Y(1993) A selective LTP of NMDA receptor-mediated currents induced by anoxia in CA hippocampal neuronsJ Neurophysiol70: 520452055 |
14 | Davare M, Lemon R, Olivier E(2008) Selective modulation of interactions between ventral premotor cortex and primary motor cortex during precision grasping in humansJ Physiol586: Pt1127352742 |
15 | Davis GW(2006) Homeostatic control of neural activity, from phenomenology to molecular designAnnu Rev Neurosci29: 307323 |
16 | de Tommaso M, Navarro J, Lanzillotti C, Ricci K, Buonocunto F, Livrea P, Lancioni GE(2015) Cortical responses to salient nociceptive and not nociceptive stimuli in vegetative and minimal conscious stateFront Hum Neurosci9: 17 |
17 | Desmurget M, Grafton S(2000) Forward modeling allows feedback control for fast reaching movementsTrends Cogn Sci4: 11423431 |
18 | Di Filippo M, Tozzi A, Costa C, Belcastro V, Tantucci M, Picconi B, Calabresi P(2008) Plasticity and repair in the post-ischemic brainNeuropharmacology55: 3353362 |
19 | Di H, Nie1 Y, Hu X, Tong Y, Heine L, Wannez S, Huang W(2014) Assessment of visual fixation in vegetative and minimally conscious statesBMC Neurology14: 147 |
20 | Ding MC, Wang Q, Lo EH, Stanley GB(2011) Cortical excitation and inhibition following focal traumatic brain injuryJ Neurosci31: 401408514094 |
21 | Dolce G, Lucca LF, Candelieri A, Rogano S, Pignolo L, Sannita WG(2011) Visual pursuit in the severe disorder of consciousnessJ Neurotrauma28: 711491154 |
22 | Dum RP, Strick PL(2005) Frontal lobe inputs to the digit representations of the motor areas on the lateral surface of the hemisphereJ Neurosci25: 613751386 |
23 | Formisano R, D’Ippolito M, Catani S(2013) Functionallocked-in syndrome as recovery phase of vegetative stateBrain Inj27: 111332 |
24 | Gall C, Fedorov AB, Ernst L, Borrmann A, Sabel BA(2010) Repetitive transorbital alternating current stimulation in optic neuropathyNeuroRehabilitation27: 4335341 |
25 | Gall C, Sgorzaly S, Schmidt S, Brandt S, Fedorov A, Sabel BA(2011) Noninvasive transorbital alternating currentstimulation improves subjective visual functioning andvision-related quality of life in optic neuropathyBrainStimul4: 4175188 |
26 | Garcia-Larrea L, Peyron R(2013) Pain matrices andneuropathic pain matrices, A review.,S-SPain154: S143 |
27 | Gerrard P, Zafonte R, Giacino JT(2014) Coma recoveryscale-revised, evidentiary support forhierarchical grading of level of consciousnessArch Phys Med Rehabil95: 1223352341 |
28 | Giacino J, Ashwal T, Childs S, Cranford N, Jennett R, Katz B, Kelly DI, Rosenberg JP, Whyte JH, Zafonte J, Zasler ND(2002) The minimally conscious state, definition and diagnostic criteriaNeurology58: 3349353 |
29 | Giacino JT, Zasler ND(1995) Outcome after severe traumatic brain injury, coma vegetative state and the minimally responsive stateJ Head Trauma Rehab10: 14056 |
30 | Goodale MA, Milner AD(1992) Separate visual pathways for perception and actionTrends Neurosci15: 12025 |
31 | Grossberg S(2003) How does the cerebral cortex work? Development, learning, attention, and -D vision by laminar circuits of visual cortexBehav Cogn Neurol Rev2: 14776 |
32 | Haig AJ, Katz RT, Sahgal V(1987) Mortality andcomplications of the locked-in syndromeArch Phys MedRehabil68: 12427 |
33 | Hildebrandt H, Happe S, Deutschmann A, Basar-Eroglu C, Eling P, Brunhöber J(2007) Brain perfusion and VEP reactivity in occipital and parietal areas are associated torecovery from hypoxic vegetative stateJ Neurol Sci260: 1-2150158 |
34 | Humphreys GW, Riddoch MJ, Price J(1997) Top-downprocesses in object identification: Evidence from experimentalpsychology, neuropsychology and functional neuroanatomyPhil Trans R Soc Lond B352: 135812751282 |
35 | Ikkai A, Curtis CE(2011) Common neural mechanismssupporting spatial working memory, attention and motor intentionNeuropsychologia49: 614281434 |
36 | Jeannerod M, Decety J, Michel F(1994) Impairment of grasping movements following a bilateral posterior parietal lesionNeuropsychologia32: 4369380 |
37 | Kaelin-Lang A, Luft AR, Sawaki L, Burstein AH, Sohn YH, Cohen LG(2002) Modulation of human cortico-motor excitability by somatosensory inputJ Physiol540: Pt2623633 |
38 | Katsuki F, Constantinidis C(2012) Early involvement ofprefrontal cortex in visual bottom-up attentionNatNeurosci15: 811601166 |
39 | Keliris GA, Logothetis NK, Tolias AS(2010) The role of the primary visual cortex in perceptual suppression of salient visual stimuliJ Neurosci30: 371235312365 |
40 | Koch G, Fernandez Del Olmo M, Cheeran B, Ruge D, Schippling S, Caltagirone C, Rothwell JC(2007) Focal stimulation of the posterior parietal cortex increases the excitability of the ipsilateral motor cortexJ Neurosci27: 2568156822 |
41 | Koch G, Franca M, Mochizuki H, Mni B, Caltagirone C, Rothwell JC(2007) Interactions between pairs of transcranial magnetic stimuli over the human left dorsal premotor cortex differ from those seen in primary motor cortexJ Physiol578: Pt2551562 |
42 | Koch G, Ruge D, Cheeran B, Fernandez Del Olmo M, Pecchioli C, Marconi B, Versace V, Lo Gerfo E, Torriero S, Oliveri M, Caltagirone C, Rothwell JC(2009) TMS activation of inter-hemisphericpathways between the posterior parietal cortex and thecontralateral motor cortexJ Physiol587: Pt1742814292 |
43 | Kokaia Z, Andsberg G, Yan Q, Lindvall O(1998) Rapid alterations of BDNF protein levels in the rat brain after focal ischemia: Evidence for increased synthesis and anterograde axonal transportExper Neurol154: 2289301 |
44 | Kotchoubey B, Veser S, Real R, Herbert C, Lang S, Kübler A(2013) Towards a more precise neurophysiological assessment of cognitive functions in patients with disorders of consciousnessRestor Neurol Neurosci31: 4473485 |
45 | Kravitz DJ, Saleem KS, Baker CI, Mishkin M(2011) A new neural framework for visuo-spatial processingNat Rev Neurosci12: 4217230 |
46 | Laureys S, Celesia GG, Cohadon F, Lavrijsen J, León-Carrión J, Sannita WG, Sazbon L, Schmutzhard L, von Wild KR, Zeman A, Dolce Gthe European TaskForce onDisorders of Consciousness(2010) Unresponsivewakefulness syndrome: A new name for the vegetative state orapallic syndromeBMC Med8: 68 |
47 | Laureys S, Faymonville ME, Luxen A, Lamy M, Franck G, Maquet P(2010) RestorationofthalamocorticalconnectivityafterrecoveryfrompersistentvegetativestateLancet355: 921717901791 |
48 | Laureys S, Pellas F, Van Eeckhout P, Ghorbel S, Schnakers C, Perrin F, Berre J, Faymonville ME, Pantke KH, Damas F, Lamy M, Moonen G, Goldman S(2005) The locked-in syndrome: What is it like to be conscious but paralyzed and voiceless?Prog Brain Res150: 495511 |
49 | Leopold DA, Logothetis NK(1996) Activity changes in early visual cortex reflect monkeys’ percepts during binocular rivalryNature379: 6565549553 |
50 | Liebetanz D, Nitsche MA, Tergau F, Paulus W(2002) Pharmacological approach to the mechanisms of transcranial DC stimulation induced after-effects of human motor cortex excitabilityBrain125: Pt1022382247 |
51 | Machado S, Arias-Carrión O, Sampaio I, Bittencourt J, Velasques B, Teixeira S, Nardi AE, Piedade R, Ribeiro P(2014) Source imaging of P visual evoked potentials and cognitive functions in healthy subjectsClin EEG Neurosci45: 4262268 |
52 | Miranda PC, Lomarev M, Hallett M(2006) Modeling thecurrent distribution during transcranial direct currentstimulationClin Neurophysiol117: 716231629 |
53 | Monti MM, Laureys S, Owen AM(2010) The vegetative stateBMJ341: c3765 |
54 | Monti MM, Pickard JD, Owen AM(2013) Visual cognitionin disorders of consciousness, from V to top-down attentionHum Brain Mapp34: 612451253 |
55 | Naro A, Calabrò RS, Russo M, Leo A, Pollicino P, Quartarone A, Bramanti P(2015) Can transcranial directcurrent stimulation be useful in differentiating unresponsivewakefulness syndrome from minimally conscious state patients?Restor Neurol Neurosci33: 2159176 |
56 | Naro A, Leo A, Russo M, Quartarone A, Bramanti P, Calabrò RS(2015) Shapingthalamo-cortical plasticity, a marker ofcortical pain integration in patients with post-anoxicunresponsive wakefulness syndrome?Brain Stimul8: 197104 |
57 | Naro A, Russo M, Leo A, Bramanti P, Quartarone A, Calabrò RS(2014) A single session of repetitivetranscranial magnetic stimulation over the dorsolateral prefrontalcortex in patients with unresponsive wakefulness syndrome,preliminary resultsNeurorehabil Neural RepairDOI: 10.1177/1545968314562114 |
58 | Nitsche MA, Liebetanz D, Lang N, Antal A, Tergau F, Paulus W(2003) Safety criteria for transcranial direct currentstimulation (tDCS) in humansClin Neurophysiol114: 1122202222 |
59 | Nitsche MA, Seeber A, Frommann K, Klein CC, RochfordC , Nitsche MS, Fricke K, Liebetanz D, Lang N, Antal A, Paulus W, Tergau F(2005) Modulatingparameters ofexcitability during and after transcranial direct currentstimulation of the human motor cortexJ Physiol568: Pt1291303 |
60 | O’Shea J, Sebastian C, Boorman ED, Johansen-Berg H, Rushworth MF(2007) Functional specificity of humanpremotor-motor cortical interactions during action selectionEurJ Neurosci26: 720852095 |
61 | O’Regan JK, Noë A(2011) A sensorimotor account ofvision and visual consciousnessBehav Brain Sci24: 59391031 |
62 | Owen AM, Coleman MR, Boly M, Davis MH, Laureys S, Pickard JD(2006) Detecting awareness in the vegetative stateScience313: 57921402 |
63 | Perrin F, Schnakers C, Schabus M, Degueldre C, Goldman S, Brédart S, Faymonville ME, Lamy M, Moonen G, Luxen A, Maquet P, Laureys S(2006) Brain response to one’s own name in vegetative state, minimally conscious state and locked-in syndromeArch Neurol63: 4562569 |
64 | Petrides M, Pandya DN(2006) Efferent association pathways originating in the caudal prefrontal cortex in the macaque monkeyJ Comp Neurol498: 2227251 |
65 | Pinto Y, van der Leij AR, Sligte IG, Lamme VAF, Scholte HS(2013) Bottom-up and top-down attention are independentJ Vis13: 316 |
66 | Ridding MC, Uy J(2003) Changes in motor cortical excitability induced by paired associative stimulationClin Neurophysiol114: 814371444 |
67 | Ridding MC, Brouwer B, Miles TS, Pitcher JB, Thompson PD(2000) Changes in muscle responses to stimulation of the motor cortex induced by peripheral nerve stimulation in human subjectsExp Brain Res131: 1135143 |
68 | Riganello F, Sannita WG(2009) Residual brain processing in the vegetative stateJ Psychophysiol23: 11826 |
69 | Rioult-Pedotti MS, Friedman D, Donoghue JP(2000) Learning-induced LTP in neocortexScience290: 5491533536 |
70 | Rizzo V, Terranova C, Crupi D, Sant’Angelo A, Girlanda P, Quartarone A(2014) Increased transcranial direct current stimulation after effects during concurrent peripheral electrical nerve stimulationBrain Stimul7: 1113121 |
71 | Rossini PM, Barker AT, Berardelli A, Caramia MD, Caruso G, Cracco RQ, Dimitrijevid MR, Hallett M, Katayama Y, Liicking CH, Maertens de Noordhout AL, Marsden CD, Murray NMF, Rothwell JC, Swash M, Tomberg C(1994) Non-invasive electrical and magnetic stimulation of the brain, spinal cord and roots, principles and procedures for routine clinical application. Report of an IFCN committeeElectroencephalogr Clin Neurophysiol91: 27992basic |
72 | Sabel BA, Fedorov AB, Naue N, Borrmann A, Herrmann C, Gall C(2011) Non-invasive alternating current stimulation improves vision in optic neuropathyRestor Neurol Neurosci29: 6493505 |
73 | Sanes JN, Donoghue JP(2000) Plasticity and primary motor cortexAnnu Rev Neurosci23: 393415 |
74 | Saron CD, Schroeder CE, Foxe JJ, Vaughan HGJr(2001) Visual activation of frontal cortex, segregation from occipital activityBrain Res Cogn BrainRes12: 17588 |
75 | Schiff ND(2010) Recovery of consciousness after brain injury: A mesocircuit hypothesisTrends Neurosci33: 119 |
76 | Schiff ND, Giacino JT, Kalmar K, Victor JD, Baker K, Gerber M, Fritz B, Eisenberg B, Biondi T, O’Connor J, Kobylarz EJ, Farris S, Machado A, McCagg C, Plum F, Fins JJ, Rezai AR(2007) Behavioral improvements with thalamic stimulation after severe traumatic brain injuryNature448: 7153600603 |
77 | Schnakers C, Giacino JT, Løvstad M, Habbal D, Boly M, Di H, Majerus S, Laureys S(2014) Preserved Covert Cognition in Non-communicative Patients With Severe Brain Injury?Neurorehabil Neural Repair29: 4308317 |
78 | Sowman PF, Dueholm SS, Rasmussen JH, Mrachacz-Kersting N(2014) Induction of plasticity in the human motor cortex by pairing an auditory stimulus with TMSFront Hum Neurosci8: 398 |
79 | Stefan K, Kunesch E, Cohen LG, Benecke R, Classen J(2000) Induction of plasticity in the human motor cortex by paired associative stimulationBrain123: Pt3572584 |
80 | Stefan K, Wycislo M, Classen J(2004) Modulation of associative human motor cortical plasticity by attentionJ Neurophysiol92: 16672 |
81 | Suppa A, Biasiotta A, Belvisi D, Marsili L, La Cesa S, Truini A, Cruccu G, Berardelli A(2013) Heat-evokedexperimental pain induces long-term potentiation-like plasticityin human primary motor cortexCereb Cortex23: 819421951 |
82 | Suppa A, Li Voti P, Rocchi L, Papazachariadis O, Berardelli A(2015) Early Visuo-motor Integration Processes Induce LTP/LTD-Like Plasticity in the Human Motor CortexCereb Cortex25: 3703712 |
83 | The Multi-Society Task Force on PVS(1994) Medical aspects ofthe persistent vegetative stateN Engl J Med330: 2114991508 |
84 | Thiebaut de Schotten M, Urbanski M, Valabregue R, Bayle DJ, Volle E(2014) Subdivision of the occipital lobes, an anatomical and functional MRI connectivity studyCortex56: 121137 |
85 | Tokimura H, Di Lazzaro V, Tokimura Y, Oliviero A, ProficeP , Inolsa A, Mazzone P, Tonali P, Rothwell JC(2000) Short latency inhibition of human hand motor cortex bysomatosensory input from the handJ Physiol523: Pt2503513 |
86 | Tokimura H, Ridding MC, Tokimura Y, Amassian VE, Rothwell JC(1996) Short latency facilitation between pairs ofthreshold magnetic stimuli applied to human motor cortexElectroencephalogr Clin Neurophysiol101: 4263272 |
87 | Trojano L, Moretta P, Loreto V, Cozzolino A, Santoro L, Estraneo A(2012) Quantitative assessment of visual behavior in disorders of consciousnessJ Neurol259: 9188895 |
88 | Valeriani M, Restuccia D, Di Lazzaro V, Oliviero A, Profice P, Le Pera D, Saturno E, Tonali P(1999) Inhibition of the human primary motor area by painful heat stimulation of the skinClin Neurophysiol110: 814751480 |
89 | Valls-Sole J, Pascual-Leone A, Wassermann EM, Hallett M(1992) Human motor evoked responses to paired transcranial magnetic stimuliElectroencephalogr Clin Neurophysiol85: 6355364 |
90 | Wolters A, Sandbrink F, Schlottmann A, Kunesch E, Stefan K, Cohen LG, Benecke R, Classen J(2003) A temporally asymmetric Hebbian rule governing plasticity in the human motor cortexJ Neurophysiol89: 523392345 |
91 | Ziemann U, Paulus W, Nitsche MA, Pascual-Leone A, ByblowWD , Berardelli A, Siebner HR, Classen J, Cohen LG, Rothwell JC(2008) Consensus, motor cortex plasticityprotocolsBrain Stimul1: 3164182 |
Figures and Tables
Fig.1
Summarizes the experimental design. We carried the clinical (CRS-R) and the electrophysiological measurements (single pulse – sp-, paired-pulse – pp-, dual-site – ds- TMS, and visuomotor integration -VMI) before (TPRE) and after (T0, T30, and T60) each conditioning protocol: real_protocol, sham_protocol, tDCS_alone, and tACS_alone.
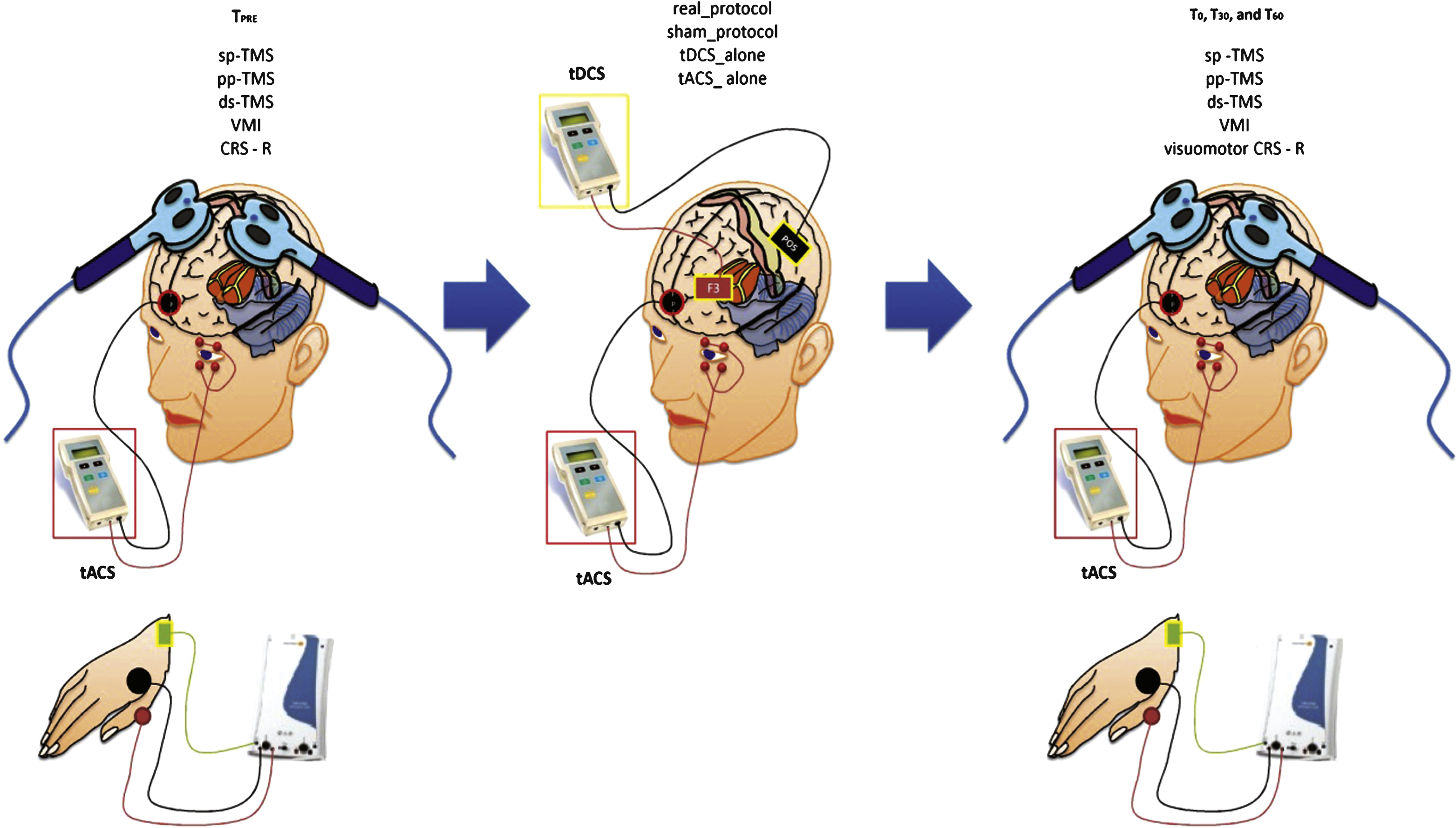
Fig.2
The electrophysiological after-effects following the real_protocol (the ineffective protocols are not shown), which were significant up to T30 for HC ( *), and to T0 for MCS (#). UWS patients did not show any significant after-effects (except for n. 3). Indeed, UWS showed before and after the real_protocol a wide impairment of cortical excitability and connectivity, whereas MCS showed an amelioration of such parameters. Values are expressed as percent of the unconditioned one. Error bars refer to standard error.
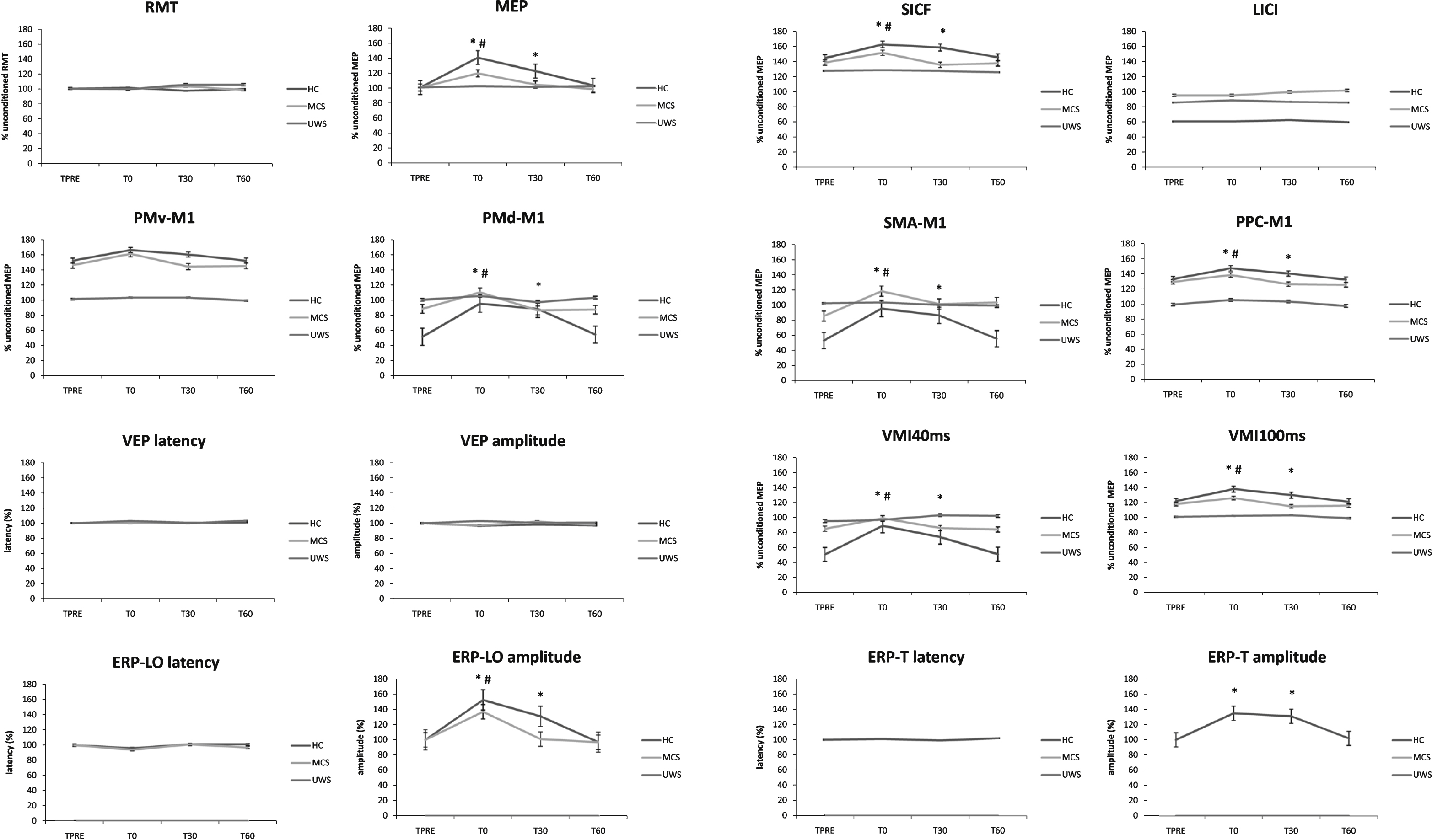
Table 1
The Clinical and demographic characteristics of the whole sample. We reported the individual CRS-R mean values ± sd (the CRS-R was daily administered for 30 consecutive days before the protocol enrollment). We marked in bold the patients who showed a visuo-motor improvement after the real_protocol application
DOC | gender | etiology | age | BI | MRI | CRS-R | ||||||
total | A | V | M | OM | C | Ar | ||||||
MCS | F | A | 72 | 6 | WMH | 18 ± 0.7 | 4 ± 1.4 | 4 ± 0.6 | 5 ± 1.9 | 1 ± 1.8 | 1.8 ± 0.9 | 3 ± 1.3 |
M | T | 51 | 18 | WMH, RBG_h | 15 ± 1.6 | 3 ± 1.5 | 3 ± 0.9 | 4 ± 1.7 | 1 ± 1.2 | 0.6 ± 0.3 | 3 ± 0.8 | |
F | A | 66 | 9 | WMH | 12 ± 1.7 | 1 ± 0.9 | 3 ± 1.2 | 2 ± 0.8 | 2 ± 0.7 | 1.6 ± 0.9 | 3 ± 0.9 | |
F | T | 70 | 22 | LFb_h | 15 ± 0.6 | 3 ± 1.6 | 2 ± 0.3 | 5 ± 0.5 | 1 ± 0.6 | 0.6 ± 0.2 | 3 ± 1.9 | |
M | T | 33 | 8 | multiple h | 13 ± 0.8 | 2 ± 1 | 2 ± 0.7 | 3 ± 0.5 | 2 ± 1.2 | 1.9 ± 0.5 | 3 ± 1.4 | |
F | A | 41 | 15 | WMH | 12 ± 1 | 1 ± 0.5 | 1 ± 0.6 | 3 ± 0.5 | 2 ± 0.3 | 0.6 ± 0.4 | 3 ± 1.9 | |
M | T | 35 | 16 | WMH, RBG_h | 11 ± 0.2 | 1 ± 1.8 | 1 ± 1.7 | 3 ± 0.7 | 2 ± 1.8 | 1.7 ± 0.6 | 3 ± 0.1 | |
mean ± sd | 53 ± 17 | 13 ± 6 | 14 ± 0.9 | 2 ± 1.2 | 2 ± 0.9 | 4 ± 0.9 | 1.6 ± 1.1 | 1 ± 1.3 | 3 ± 1.1 | |||
UWS | M | A | 53 | 8 | WMH | 5 ± 0.4 | 1 ± 1.1 | 1 ± 1.2 | 1 ± 1.1 | 1 ± 0.6 | 0.4 ± 0.1 | 1 ± 1.5 |
F | T | 26 | 3 | DAI, SAH | 4 ± 1.4 | 1 ± 0.7 | 1 ± 0.8 | 1 ± 1.5 | 0 ± 1.4 | 1.3 ± 1 | 1 ± 0.6 | |
F | T | 66 | 8 | RFP_h | 7 ± 1 | 0 ± 1.2 | 2 ± 1.5 | 2 ± 1.4 | 1 ± 1.4 | 1 ± 1 | 1 ± 2 | |
F | A | 62 | 11 | WMH | 6 ± 1 | 1 ± 1.3 | 1 ± 1.2 | 2 ± 0.6 | 0 ± 0.3 | 0.8 ± 0.9 | 2 ± 0.4 | |
M | T | 61 | 9 | SAH | 4 ± 0.7 | 1 ± 1 | 1 ± 0.2 | 1 ± 0.2 | 0 ± 1.2 | 1.1 ± 0.2 | 1 ± 1.6 | |
M | A | 69 | 11 | WMH | 7 ± 1.3 | 1 ± 0.1 | 1 ± 0.7 | 2 ± 0.7 | 1 ± 1.1 | 1.4 ± 0.6 | 2 ± 0.9 | |
F | T | 74 | 12 | DAI, SAH | 6 ± 0.3 | 1 ± 1.4 | 2 ± 1.4 | 1 ± 1.4 | 0 ± 0.7 | 0.4 ± 0.6 | 2 ± 1.4 | |
mean ± sd | 59 ± 16 | 9 ± 3 | 6 ± 1 | 0.9 ± 0.4 | 1.3 ± 0.5 | 1.5 ± 0.5 | 0.4 ± 0.5 | 0.9 ± 0.6 | 1.6 ± 0.5 |
Etiology: A, post-anoxic, T, post-traumatic brain injury; BI: brain injury onset in months; age in years; MRI: structural patterns including WMH (white matter hyper-intensity), _h (hemorrhagic lesion), RFP (right fronto-polar), RBG (basal ganglia), LFb (left fronto-basal), SAH (sub-arachnoid hemorrhage), DAI (diffuse axonal injury); CRS-R: Coma Recovery Scale-revised including auditory (A), visual (V), motor (M), oro-motor (OM), communication domain (C), and arousal induction (Ar); sd: standard deviation.
Table 2
The comparison among electrophysiological parameters in HC, MCS and individuals with UWS. We found significant differences between MCS and UWS (pMCS/UWS), and DOC and HC ( *) (each unpaired t-tests, p < 0.05, except for RMT% , MEP amplitude, VEP amplitude and latency). UWS patients did not show ERPs, with a global impairment of cortical excitability, connectivity, and VEP latency and amplitude. MCS displayed an increase of cortical excitability (increased VMI100, SICF, PMv, and PPC; reduced VMI40, PMd, SMA, and LICI), a partially preserved premotor-motor and VMI connectivity, and the presence of ERPs limited to LO electrode. Some MCS/UWS differences approached the statistical significance (labeled as NS unpaired t - test ). Data are reported as mean ± se
Measures | HC | MCS | UWS | PMCS/UWS | |
sp | RMT (%) | 55 ± 3 | 58 ± 2 | 60 ± 9 | NS |
MEP (mV) | 0.7 ± 0.1 | 0.5 ± 0.1 | 0.4 ± 0.1 | NS | |
ds | PMv-M1 (%) | 125 ± 9 * | 142 ± 6 | 105 ± 7 | <0.001 |
PMd-M1 (%) | 51 ± 7 * | 92 ± 7 | 105 ± 9 | NS0.06 | |
SMA (%) | 53 ± 9 * | 94 ± 6 | 106 ± 8 | NS0.07 | |
PPC-M1 (%) | 123 ± 11 * | 148 ± 6 | 105 ± 7 | <0.001 | |
pp | LICI (%) | 60 ± 7 * | 94 ± 13 | 107 ± 5 | NS |
SICF (%) | 129 ± 9 * | 144 ± 13 | 167 ± 5 | NS0.06 | |
VMI40 (%) | 51 ± 8 * | 79 ± 6 | 97 ± 9 | <0.001 | |
VMI100 (%) | 112 ± 5 * | 122 ± 6 | 108 ± 9 | 0.01 | |
tACS | VEP latency (ms) | 102 ± 7 | 115 ± 2 | 121 ± 3 | NS |
VEP amplitude ( μV) | 19 ± 3 | 12 ± 2 | 8 ± 1 | NS | |
ERPLLO latency (ms) | 303 ± 10 * | 340 ± 15 | Absent | <0.001 | |
ERPLLO amplitude ( μV) | 17 ± 8 * | 6 ± 4 | Absent | 0.002 | |
ERPLT latency (ms) | 331 ± 12 * | Absent | Absent | NS | |
ERPLT amplitude ( μV) | 10 ± 4 * | Absent | Absent | NS |
sp: single pulse TMS; ds: dual-site TMS; pp: paired-pulse TMS.
Table 3
We observed significant real_tDCS after-effects in HC and MCS patients, whereas UWS did not show any significant after-effect at group level. The tDCS_alone induced after-effects that were similar to the real_protocol but non-significant and limited to the MEP amplitude increase and the PPC-M1 potentiation. The tACS_alone and the sham_protocol were totally ineffective
timeXgroupXprotocol interaction F (18,324) , p | real_protocol | ||||
HC t (1,6) , p | MCS t (1,6) , p | ||||
MEP amplitude | 3.0 <0.001 | T0 | 3.2, 0.02 | 3, 0.01 | |
T30 | 2.7, 0.03 | NS | |||
T60 | NS | ||||
ERPLLO amplitude | 3.2, <0.001 | T0 | 6.1, <0.001 | 2.5, 0.04 | |
T30 | 6, <0.001 | NS | |||
T60 | NS | ||||
ERPLT amplitude | NS | T0 | 6, <0.001 | NS | |
T30 | 5.1, 0.005 | NS | |||
T60 | NS | ||||
PMd-M1 % | 1.7, 0.04 | T0 | 6.4, <0.001 | 2.3, 0.04 | |
T30 | 3.2, 0.02 | NS | |||
T60 | NS | ||||
PPC-M1 % | 3.8, <0.001 | T0 | 3.2, 0.02 | 2.3, 0.04 | |
T30 | 2.4, 0.04 | NS | |||
T60 | NS | ||||
SICF % | 1.8, 0.02 | T0 | 2.9, 0.01 | 2.8, 0.01 | |
T30 | 2.7, 0.03 | NS | |||
T60 | NS | ||||
VMI40 % | 7.9, <0.001 | T0 | 9.1, <0.001 | 3.2, 0.02 | |
T30 | 6.3, 0.003 | NS | |||
T60 | NS | ||||
VMI100 % | 5.4, <0.001 | T0 | 3.8, 0.003 | 3.2, 0.02 | |
T30 | 3.2, 0.02 | NS | |||
T60 | NS | ||||
SMA-M1 % | 2.6, <0.001 | T0 | 2.8, 0.01 | 2.8, 0.01 | |
T30 | 2.7, 0.03 | NS | |||
T60 | NS |