Cut and paste the genome: Genome editing for research and therapy
Abstract
Genome engineering, or alternatively called ‘genome editing,’ has been one of the rapidly growing fields of biotechnology for the last few decades. Scientists are now making targeted modifications of genome in any organism of choice with improved precision. In this mini review, we provide basic, fundamental theory and mechanisms of the well-known genome editing technologies such as zinc-finger nucleases (ZFNs), transcription activator-like effector nucleases (TALENs) and clustered regulatory interspaced short palindromic repeats (CRISPR)/Cas9 system. We also discuss its role in the field of genetic research and highlight its therapeutic potential as an indispensable tool for the development of personalized medicine to come in near future.
1Introduction
For the last few decades, the biotechnology to decipher individual human genome such as whole-genome sequencing (WGS, [1]) and whole-exome sequencing (WES, [2]) advanced greatly, lowering barriers to decoding the information embedded in human genome. Such technological advances are anticipated to revolutionize our understanding of clinical genetics and to deliver personalized medicine in near future. Nevertheless, the present challenge lies in converting this tremendous amount of data (i.e., billions of nucleotides that comprise DNA of an individual) into clinically relevant information, such as how genotype affects phenotype. To transform the data into functionally relevant knowledge, technologies such as targeted gene inactivation by homologous recombination [3] or targeted gene knockdown by RNAi [4] have been utilized as means of providing empirical information to elucidate the function of genes of interest. However, the former has limitations such as extremely low efficiency of correct insertion into the target chromosome (1 in 106∼1 in 109) [5] and potential adverse mutagenesis [6], while the latter has limitations including incomplete and temporary knockdown of target gene and undesired off-target effects [7]. More recently, genome engineering technologies, commonly referred to as ‘genome editing,’ have been emerged, enabling scientists to make targeted modifications to the genome in practically any organism of choice with improved precision [7a]. This technology utilizes engineered nucleases which are complex of sequence-specific DNA binding domains and nonspecific DNA cleavage modules [8]. In this brief review, we aim to provide basic information on this genome editing technologies and discuss the applications and the therapeutic potential of these technologies, as well as future prospects.
2Principle mechanisms of genome editing technologies
Genome editing technology involves customized programmable DNA-binding nucleases such as zinc-finger nucleases (ZFNs) [8], transcription activator-like effector nucleases (TALENs) [9], and clustered regularly interspaced short palindromic repeats (CRISPR)/Cas9 (CRISPR-associated 9) nucleases [10]. Although each nuclease has unique mode of action, in principle, these nucleases recognize, bind to, and cleave chromosomal DNA to create site-specific DNA double strand breaks (DSBs). Subsequently, these DSBs trigger endogenous DNA repair systems, such as homology directed repair (HDR) or error-prone non-homologous end joining (NHEJ), resulting in targeted genome modification (Fig. 1) [11].
3Programmable DNA-binding nucleases
3.1Zinc-finger nucleases (ZFNs)
First discovered in transcription factor from Xenopus laevis [12], Cys2-His2 zinc-fingers are the most common sequence-specific DNA-binding motifs found in all eukaryotic organisms [13]. Each zinc-finger motif consists of about 30 amino acids in a ββα configuration, and two cysteines and two histidines coordinate a single zinc atom in individual zinc-finger [14]. Amino acids in positions −1, 3, and 6 on the α-helix contact 3 base pairs in the major groove of DNA [15]. Thus, theoretically, a combination of 6 separate zinc-fingers that each recognizes a 3 base pair DNA sequence can consequently recognize a specific DNA sequence of 18 base pairs, which is long enough sequence to specify a unique site in the human genome. The construction of such unnatural, synthetic zinc-fingers that recognized 18 base pairs of DNA sequence made application of zinc-finger proteins for recognizing specific DNA sequence possible [16]. However, it was the combination of zinc-finger proteins with cleavage domain of endonuclease that made possible to realize the concept of targeted genome engineering. Isolated from Flavobacterium okeanokoites, the type II restriction endonuclease Fok I recognizes the DNA sequence of 5’-GGATG-3’ and cleaves 9th and 13th base pairs from the recognition site, regardless of the sequence at the cleaved site [17]. When the cleavage domain of Fok I endonuclease was combined with zinc-finger protein to generate zinc-finger-Fok I fusion proteins, these hybrid fusion proteins (ZFNs) were able to cut DNA at the predetermined sequences [18].
Since Fok I cleavage domain must dimerize to catalyze DNA cleavage, during ZFN-mediated site specific DNA cleavage, ZFN target sites consisted of two zinc-finger binding sites separated by 5∼7 base pairs of spacer sequence are occupied by two ZFNs on each strand and the cleavage domain of each ZFN dimerize on the spacer sequence and cleaves it (Fig. 2). Currently there are 3 large sets of constructed ZF proteins (ZFPs) from independent groups, namely The Scripps Research Institute [19–21], Sangamo Biosciences [22], and ToolGen [23], are available. Recently published articles on the use of ZFNs are listed in Table 1. The contents of Table 1 are not based on scientific significance by any means. It is just examples of recent research trend involving use of ZFNs. As demonstrated in the Table below, researches on ZFNs are still active, and most of the cases the ZFNs are utilized as a tool for studying function of certain genes by inducing gene disruptions. However, there are also studies to correct mutations in human cells, implicating the therapeutic potential of genome editing using ZFNs.
3.2Transcription activator-like effector nucleases (TALENs)
TAL (transcription activator-like) proteins were first identified as secreted proteins from bacterial plant pathogen Xanthomonas, and the named based on observation that they activated transcription of endogenous pathogenic genes in plants [35, 36]. Once they enter the nucleus of host cells, they bind to effector-specific sequences on the promoters of host genes to initiate transcription [37]. TALENs are composed of DNA-binding domain (DBD), N-terminal, C-terminal, and FokI endonuclease domain. The DBD of TALEN is composed of ‘repeats’ that itself composed of 33∼35 amino acids [38].
Among these amino acids, the amino acid residues at position 12 and 13 of each repeat decide which nucleotide of DNA they bind to (i.e., Asparagine-Isoleucine (NI) ⟶ Adenine (A), Asparagine-Glycine (NG) ⟶ Thymine (T), Aspargine-Asparagine (NN) ⟶ Guanine (G), Histidine-Aspartic Acid (HD) ⟶ Cytosine (C)) so they are named as ‘repeat-variable di-residue (RVD) [39]. It is this one RVD to one nucleotide match between the RVD of TALENs and chromosomal DNA that underpins target sequence specificity of TALENs (Fig. 3A). Furthermore, this sequence specific interaction and simplicity of its coding system made generation of customized TALE domains that target DNA sequence of interest possible [40, 41].
The TALE domains can be assembled in predetermined combinations and linked to the Fok I nuclease to drive sequence-specific DSB of chromosomal DNA [42]. TALENs, in pairs, bind to opposing target sequences so that the linked Fok I nucleases come in contact with the spacer from opposite sides. The Fok I nucleases from each TALEN forms Fok I dimer and it cleaves double-stranded DNA (Fig. 3B). As indicated in the Table 2, TALEN-mediated genome editing is also widely used to facilitate gene disruption, addition, and correction.
3.3Clustered regulatory interspaced short palindromic repeat (CRISPR)/Cas9
The existence of CRISPR was first discovered by Nakata et al. in studying iap gene of E.coli [62]. What they found was 29 nucleotide-long repeats interspaced by 5 intervening 32 nucleotide-long, non-repetitive sequences. The actual word CRISPR for describing such repeat sequences was first coned by Jansen in 2002 [63]. The CRISPR loci are composed of a set of CRISPR-associated (Cas) genes and a series of repeats (direct repeats, approximately 20–50 base pairs) interspaced by unique, non-repetitive sequences (spacers).
These non-repetitive sequences correspond to the sequences of foreign genetic elements (protospacers) [64]. the protospacers are flanked by a short protospacer adjacent motif (PAM) that is either located on the 3’ (type II CRISPR) or 5’ (type I CRISPR) of foreign DNA [65]. Additionally, leader sequence, rich in A+T sequences, is known to serve as a promoter element for the CRISPR loci [66] (Fig. 4). CRISPR is an essential components of RNA-based adaptive immune systems of bacteria and archaea [67]. In response to foreign genetic element challenges, bacteria and archaea incorporate short fragments of foreign genetic elements into host genome as shown in Fig. 4. When CRISPR locus is transcribed, the long primary transcript is processed to produce a library of short CRISPR-derived RNAs (crRNAs) [68, 69]. Each crRNA has a complementary sequence to a previously encountered foreign genetic elements, and it mediates detection and subsequent destruction of foreign nucleic acids [70]. This unique immune system can be divided into 3 distinct CRISPR types (type I-III) based on gene conservation and locus organization [71]. Especially in the type II CRISPR/Cas system, crRNAs hybridize with trans-activating crRNAs (tracrRNAs) to facilitate RNA-guided sequence-specific DNA cleavage by Cas9 proteins. Cas9 utilizes RNA-DNA pairing to target foreign DNA, and Cas9-RNA complex-mediated DNA cleavage requires recognition of PAM where DNA strand separation and RNA-DNA hybrid formation occurs(Fig. 5) [72].
DNA sequence. In fact, reprogrammed RNA-guided nucleases have been demonstrated to be competent in facilitating gene disruption, addition, and correction in human cells and other model organisms (Table 3).
Compared to ZFNs and TALENs, CRISPR/Cas9 system has higher efficiency and shortest target length, and it can also facilitate multiplex targeting [79]. Furthermore, Cas9 can be used for applications other than usual category of gene modifications (disruption, addition, and correction), such as, but not limited to, transcriptional control or DNA labeling [80, 81].
4Perspectives: The role of genome editing in achieving personalized medicine
Although it is in its infancy, over the last few decades, genome editing has come of age and tremendous interest has been placed on its utility as a tool for basic/clinical genetics. Accumulating data also indicate its potential as a dexterous and powerful means of personalized medicine which is expected to provide individual patient with ‘tailored’ therapeutics near future. Promising results using these site-specific nucleases in therapeutic approaches against severe combined immune deficiency (SCID) [82], sickle cell disease [83], and hemophilia B [83], make the hopes of personalized medicine up ever more. For ‘personalized medicine’ to be realized, close cooperation of related biotechnologies and disciplines is required (Fig. 6).
For example, an ideal starting point of personalized medicine would be genomic information analysis of an individual patient. Biotechnologies such as WGS and WES would be a valuable tool for this task. From the genomic analysis by sequencing can identify specific genetic defects of patients (i.e., single nucleotide polymorphism, SNP), and the relationship between specific SNP and phenotypic manifestation can be systemically examined both in vitro and in vivo. At this stage comes the genome editing technology. With the genome editing technology (i.e., gene knock in/out, point mutation, gene correction, and etc.) the functional consequences of a certain SNP can be determined in details (research-oriented utilization of genomic editing). Furthermore, genomic editing can serve as a part of therapeutic process that requires genomic modifications (i.e., gene correction of induced pluripotent stem cells (iPS) from specific patient). Thus, the genome editing technology can be both research-oriented and therapy-oriented applications. Although there are still some unsolved issues regarding detailed mechanisms of individual genome editing such as how foreign sequences are selected and incorporated into the CRISPR loci of host, it is undoubtful that these technology eventually revolutionizes the field of genetic research as well as our understanding of diseases and opens a new paradigm in fighting currently incurable diseases.
Conflicts of interest
The authors declare no conflict of interest.
Acknowledgments
This study was supported by a Korea Science and Engineering Foundation grant funded by the Korean government (MEST) (2014030459) and a grant from the Korea Health 21 R&D Project, Ministry of Health & Welfare, Republic of Korea (A120478).
References
1 | Ng PC, Kirkness EF(2010) Whole genome sequencingMethods in Molecular Biology628: 215226 |
2 | Rabbani B, Tekin M, Mahdieh N(2014) The promise of whole-exome sequencing in medical geneticsJournal of Human Genetics59: 515 |
3 | Capecchi MR(2005) Gene targeting in mice: Functional analysis of the mammalian genome for the twenty-first centuryNature Reviews Genetics6: 507512 |
4 | Mohr SE, Smith JA, Shamu CE, Neumuller RA, Perrimon N(2014) RNAi screening comes of age: Improved techniques and complementary approachesNature Reviews Molecular Cell Biology15: 591600 |
5 | Capecchi MR(1989) Altering the genome by homologous recombinationScience244: 12881292 |
6 | Vasquez KM, Marburger K, Intody Z, Wilson JH(2001) Manipulating the mammalian genome by homologous recombinationProceedings of the National Academy of Sciences of the United States of America98: 84038410 |
7 | Moore CB, Guthrie EH, Huang MT, Taxman DJ(2010) Short hairpin RNA (shRNA): Design, delivery, and assessment of gene knockdownMethods in Molecular Biology629: 141158 |
{ label needed for ref[@id='r7a'] } | Wang W, Xu X, Li Z, Lendlein A, Ma N(2014) Genetic engineering of mesenchymal stem cells by non-viral gene deliveryClin Hemorheol Microcirc58: 1948 |
8 | Urnov FD, Rebar EJ, Holmes MC, Zhang HS, Gregory PD(2010) Genome editing with engineered zinc finger nucleasesNature Reviews Genetics11: 636646 |
9 | Joung JK, Sander JD(2013) TALENs: A widely applicable technology for targeted genome editingNature Reviews Molecular Cell Biology14: 4955 |
10 | Maggio I, Holkers M, Liu J, Janssen JM, Chen X, Goncalves MA(2014) Adenoviral vector delivery of RNA-guided CRISPR/Cas9 nuclease complexes induces targeted mutagenesis in a diverse array of human cellsScientific Reports4: 5105 |
11 | Kim H, Kim JS(2014) A guide to genome engineering with programmable nucleasesNature Reviews Genetics15: 321334 |
12 | Miller J, McLachlan AD, Klug A(1985) Repetitive zinc-binding domains in the protein transcription factor IIIA from Xenopus oocytesThe EMBO Journal4: 16091614 |
13 | Pabo CO, Peisach E, Grant RA(2001) Design and selection of novel Cys2His2 zinc finger proteinsAnnual Review of Biochemistry70: 313340 |
14 | Beerli RR, Barbas CF3rd(2002) Engineering polydactyl zinc-finger transcription factorsNature Biotechnology20: 135141 |
15 | Pavletich NP, Pabo CO(1991) Zinc finger-DNA recognition: Crystal structure of a Zif268-DNA complex at 2.1 AScience252: 809817 |
16 | Liu Q, Segal DJ, Ghiara JB, Barbas CF3rd(1997) Design of polydactyl zinc-finger proteins for unique addressing within complex genomesProceedings of the National Academy of Sciences of the United States of America94: 55255530 |
17 | Sugisaki H, Kanazawa S(1981) New restriction endonucleases from Flavobacterium okeanokoites (FokI) and Micrococcus luteus (MluI)Gene16: 7378 |
18 | Kim YG, Cha J, Chandrasegaran S(1996) Hybrid restriction enzymes: Zinc finger fusions to Fok I cleavage domainProceedings of the National Academy of Sciences of the United States of America93: 11561160 |
19 | Dreier B(2005) Development of zinc finger domains for recognition of the 5’-CNN-3’ family DNA sequences and their use in the construction of artificial transcription factorsThe Journal of Biological Chemistry280: 3558835597 |
20 | Dreier B, Beerli RR, Segal DJ, Flippin JD, Barbas CF3rd(2001) Development of zinc finger domains for recognition of the 5’-ANN-3’ family of DNA sequences and their use in the construction of artificial transcription factorsThe Journal of Biological Chemistry276: 2946629478 |
21 | Segal DJ, Dreier B, Beerli RR, Barbas CF3rd(1999) Toward controlling gene expression at will: Selection and design of zinc finger domains recognizing each of the 5’-GNN-3’ DNA target sequencesProceedings of the National Academy of Sciences of the United States of America96: 27582763 |
22 | Liu Q, Xia Z, Zhong X, Case CC(2002) Validated zinc finger protein designs for all 16 GNN DNA triplet targetsThe Journal of Biological Chemistry277: 38503856 |
23 | Bae KH(2003) Human zinc fingers as building blocks in the construction of artificial transcription factorsNature Biotechnology21: 275280 |
24 | Park A, Liegel RP, Ronchetti A, Ebert AD, Geurts A, Sidjanin DJ(2014) Targeted disruption of Tbc1d20 with zinc-fingernucleases causes cataracts and testicular abnormalities in miceBMC Genetics15: 135 |
25 | Salabi F, Nazari M, Chen Q, Nimal J, Tong J, Cao WG(2014) Myostatin knockout using zinc-finger nucleases promotesproliferation of ovine primary satellite cells in vitro Journal of Biotechnology192PA: 268280 |
26 | Sampson KE, Brinker A, Pratt J, Venkatraman N, Xiao Y, Blasberg J, Steiner T, Bourner M, Thompson DC(2015) Zinc finger nuclease-mediated gene knockout results in loss of transport activity for P-glycoprotein, BCRP, andMRP2 in Caco-2 cellsDrug metabolism and disposition: the biological fate of chemicals43: 199207 |
27 | Ding W, Hu Z, Zhu D, Jiang X, Yu L, Wang X, Zhang C, Wang L, Ji T, Li K, He D, Xia X, Liu D, Zhou J, Ma D, Wang H(2014) Zinc finger nucleases targeting the human papillomavirus E7 oncogene induce E7 disruptionand a transformed phenotype in HPV16/18-positive cervical cancer cellsClinical cancer research: an officialjournal of the American Association for Cancer Research20: 64956503 |
28 | Yi G(2014) CCR5 gene editing of resting CD4(+) T cells by transient ZFN expression from HIV envelope pseudotyped nonintegrating lentivirus confers HIV-1 resistance in humanized miceMolecular Therapy Nucleic Acids3: e198 |
29 | Liu X(2014) Generation of mastitis resistance in cows by targeting human lysozyme gene to beta-casein locus using zinc-finger nucleasesProceedings Biological Sciences / The Royal Society281: 20133368 |
30 | Lombardo A(2011) Site-specific integration and tailoring of cassette design for sustainable gene transferNature Methods8: 861869 |
31 | Merling RK, Sweeney CL, Chu J, Bodansky A, Choi U, Priel DL, Kuhns DB, Wang H, Vasilevsky S, De Ravin SS, Winkler T, Dunbar CE, Zou J, Zarember KA, Gallin JI, Holland SM, Malech HL(2015) An AAVS1-targeted minigene platformfor correction of iPSCs from all five types of chronic granulomatous diseaseMolecular therapy: the journal ofthe American Society of Gene Therapy23: 147157 |
32 | Zhang W(2014) Targeted genome correction by a single adenoviral vector simultaneously carrying an inducible zinc finger nuclease and a donor templateJournal of Biotechnology 188C16 |
33 | Zou J, Mali P, Huang X, Dowey SN, Cheng L(2011) Site-specific gene correction of a point mutation in human iPS cells derived from an adult patient with sickle cell diseaseBlood118: 45994608 |
34 | Sebastiano V(2011) In situ genetic correction of the sickle cell anemia mutation in human induced pluripotent stem cells using engineered zinc finger nucleasesStem Cells29: 17171726 |
35 | Bogdanove AJ, Voytas DF(2011) TAL effectors: Customizable proteins for DNA targetingScience333: 18431846 |
36 | Kay S, Hahn S, Marois E, Hause G, Bonas U(2007) A bacterial effector acts as a plant transcription factor and induces a cell size regulatorScience318: 648651 |
37 | Bogdanove AJ, Schornack S, Lahaye T(2010) TAL effectors: Finding plant genes for disease and defenseCurrent Opinion in Plant Biology13: 394401 |
38 | Cermak T(2011) Efficient design and assembly of custom TALEN and other TAL effector-based constructs for DNA targetingNucleic Acids Research39: e82 |
39 | Moscou MJ, Bogdanove AJ(2009) A simple cipher governs DNA recognition by TAL effectorsScience326: 1501 |
40 | Miller JC(2011) A TALE nuclease architecture for efficient genome editingNature Biotechnology29: 143148 |
41 | Zhang F, Cong L, Lodato S, Kosuri S, Church GM, Arlotta P(2011) Efficient construction of sequence-specific TAL effectors for modulating mammalian transcriptionNature Biotechnology29: 149153 |
42 | Li T(2011) TAL nucleases (TALNs): Hybrid proteins composed of TAL effectors and FokI DNA-cleavage domainNucleic Acids Research39: 359372 |
43 | Zhang Z, Wu E, Qian Z, Wu WS(2014) A multicolor panel of TALE-KRAB based transcriptional repressor vectors enabling knockdown of multiple gene targetsScientific Reports4: 7338 |
44 | Elbaz I, Lerer-Goldshtein T, Okamoto H, Appelbaum L(2014) Reduced synaptic density and deficient locomotor response inneuronal activity-regulated pentraxin 2a mutant zebrafishFASEB Journal: Official Publication of the Federationof American Societies for Experimental Biology |
45 | Choi J, Suzuki KI, Sakuma T, Shewade L, Yamamoto T, Buchholz DR(2014) Unliganded thyroid hormone receptor alpha regulates developmental timing via gene repression as revealed by gene disruption in Xenopus tropicalisEndocrinologyen20141554 |
46 | Kabir S, Hockemeyer D, de Lange T(2014) TALEN gene knockouts reveal no requirement for the conserved human shelterin protein Rap1 in telomere protection and length regulationCell Reports9: 12731280 |
47 | Lugassy J(2014) Modulation of TCR responsiveness by the Grb2-family adaptor, GadsCellular Signalling27: 125134 |
48 | Tatsumi Y, Takeda M, Matsuda M, Suzuki T, Yokoi H(2014) TALEN-mediated mutagenesis in zebrafish reveals a role forr-spondin 2 in fin ray and vertebral developmentFEBS Letters588: 45434550 |
49 | Liu C, Xiao L, Li F, Zhang H, Li Q, Liu H, Fu S, Li C, Zhang X, Wang J, Staunstrup NH, Li Y, Yang H(2014) Generation of outbred Ace2 knockout mice by RNA transfection of TALENs displaying colitis reminiscentpathophysiology and inflammationTransgenic research |
50 | Zhang Z, Zhu B, Ge W(2015) Genetic analysis of zebrafish gonadotropin (FSH and LH) functions by TALEN-mediated genedisruptionMolecular Endocrinology29: 7698 |
51 | Wen L, Fu L, Guo X, Chen Y, Shi YB(2015) Histone methyltransferase Dot1L plays a role in postembryonic development inXenopus tropicalisFASEB Journal: Official Publication of the Federation of American Societies for ExperimentalBiology29: 385393 |
52 | Chen B(2015) Disruption of microRNA-21 by TALEN leads to diminished cell transformation and increased expression of cell-environment interaction genesCancer Letters356: 506516 |
53 | Krentz NA, Nian C, Lynn FC(2014) TALEN/CRISPR-mediated eGFP knock-in add-on at the OCT4 locus does not impact differentiation of human embryonic stem Cells towards endodermPloS One9: e114275 |
54 | Li HL, Fujimoto N, Sasakawa N, Shirai S, Ohkame T, Sakuma T, Tanaka M, Amano N, Watanabe A, Sakurai H, Yamamoto T, Yamanaka S, Hotta A(2015) Precise correction of the dystrophin gene in duchenne muscular dystrophypatient induced pluripotent stem cells by TALEN and CRISPR-Cas9Stem cell reports4: 143154 |
55 | Ousterout DG(2013) Reading frame correction by targeted genome editing restores dystrophin expression in cells from Duchenne muscular dystrophy patientsMolecular Therapy: The Journal of the American Society of Gene Therapy21: 17181726 |
56 | Ramalingam S, Annaluru N, Kandavelou K, Chandrasegaran S(2014) TALEN-Mediated generation and genetic correction of disease-specific human induced pluripotent stem cellsCurrent Gene Therapy14: 461472 |
57 | Suzuki K(2014) Targeted gene correction minimally impacts whole-genome mutational load in human-disease-specific induced pluripotent stem cell clonesCell Stem Cell15: 3136 |
58 | Ma N(2013) Transcription activator-like effector nuclease (TALEN)-mediated gene correction in integration-free beta-thalassemia induced pluripotent stem cellsThe Journal of Biological Chemistry288: 3467134679 |
59 | Sun N, Zhao H(2014) Seamless correction of the sickle cell disease mutation of the HBB gene in human induced pluripotent stem cells using TALENsBiotechnology and Bioengineering111: 10481053 |
60 | Low BE, Krebs MP, Joung JK, Tsai SQ, Nishina PM, Wiles MV(2014) Correction of the Crb1rd8 allele and retinal phenotype in C57BL/6N mice via TALEN-mediated homology-directed repairInvestigative Ophthalmology & Visual Science55: 387395 |
61 | Dupuy A(2013) Targeted gene therapy of xeroderma pigmentosum cells using meganuclease and TALENPloS One8: e78678 |
62 | Ishino Y, Shinagawa H, Makino K, Amemura M, Nakata A(1987) Nucleotide sequence of the iap gene, responsible for alkaline phosphatase isozyme conversion in Escherichia coli, and identification of the gene productJournal of Bacteriology169: 54295433 |
63 | Jansen R, Embden JD, Gaastra W, Schouls LM(2002) Identification of genes that are associated with DNA repeats in prokaryotesMolecular Microbiology43: 15651575 |
64 | Shah SA, Erdmann S, Mojica FJ, Garrett RA(2013) Protospacer recognition motifs: Mixed identities and functional diversityRNA Biology10: 891899 |
65 | Mojica FJ, Diez-Villasenor C, Garcia-Martinez J, Almendros C(2009) Short motif sequences determine the targets of the prokaryotic CRISPR defence systemMicrobiology155: 733740 |
66 | Pougach K(2010) Transcription, processing and function of CRISPR cassettes in Escherichia coliMolecular Microbiology77: 13671379 |
67 | Wiedenheft B, Sternberg SH, Doudna JA(2012) RNA-guided genetic silencing systems in bacteria and archaeaNature482: 331338 |
68 | Deltcheva E(2011) CRISPR RNA maturation by trans-encoded small RNA and host factor RNase IIINature471: 602607 |
69 | Gesner EM, Schellenberg MJ, Garside EL, George MM, Macmillan AM(2011) Recognition and maturation of effector RNAs in a CRISPR interference pathwayNature Structural & Molecular Biology18: 688692 |
70 | Garneau JE(2010) The CRISPR/Cas bacterial immune system cleaves bacteriophage and plasmid DNANature468: 6771 |
71 | Makarova KS(2011) Evolution and classification of the CRISPR-Cas systemsNature Reviews Microbiology9: 467477 |
72 | Sternberg SH, Redding S, Jinek M, Greene EC, Doudna JA(2014) DNA interrogation by the CRISPR RNA-guided endonuclease Cas9Nature507: 6267 |
73 | Mehrabian M(2014) CRISPR-Cas9-based knockout of the prion protein and its effect on the proteomePloS One9: e114594 |
74 | Cho SW, Kim S, Kim JM, Kim JS(2013) Targeted genome engineering in human cells with the Cas9 RNA-guided endonucleaseNature Biotechnology31: 230232 |
75 | Cong L(2013) Multiplex genome engineering using CRISPR/Cas systemsScience339: 819823 |
76 | Mali P(2013) RNA-guided human genome engineering via Cas9Science339: 823826 |
77 | Wu Y, Zhou H, Fan X, Zhang Y, Zhang M, Wang Y, Xie Z, Bai M, Yin Q, Liang D, Tang W, Liao J, Zhou C, Liu W, Zhu P, Guo H, Pan H, Wu C, Shi H, Wu L, Tang F, Li J(2015) Correction of a genetic disease byCRISPR-Cas9-mediated gene editing in mouse spermatogonial stem cellsCell research25: 6779 |
78 | Long C, McAnally JR, Shelton JM, Mireault AA, Bassel-Duby R, Olson EN(2014) Prevention of muscular dystrophy in mice by CRISPR/Cas9-mediated editing of germline DNAScience345: 11841188 |
79 | Chen L(2014) Advances in genome editing technology and its promising application in evolutionary and ecological studiesGiga Science3: 24 |
80 | Hsu PD, Lander ES, Zhang F(2014) Development and applications of CRISPR-Cas9 for genome engineeringCell157: 12621278 |
81 | Konermann S, Brigham MD, Trevino AE, Joung J, Abudayyeh OO, Barcena C, Hsu PD, Habib N, Gootenberg JS, Nishimasu H, Nureki O, Zhang F(2015) Genome-scale transcriptional activation by an engineeredCRISPR-Cas9 complexNature517: 583588 |
82 | Urnov FD(2005) Highly efficient endogenous human gene correction using designed zinc-finger nucleasesNature435: 646651 |
83 | Li H(2011) In vivo genome editing restores haemostasis in a mouse model of haemophiliaNature475: 217221 |
Figures and Tables
Fig.1
Schematics of genome editing process.
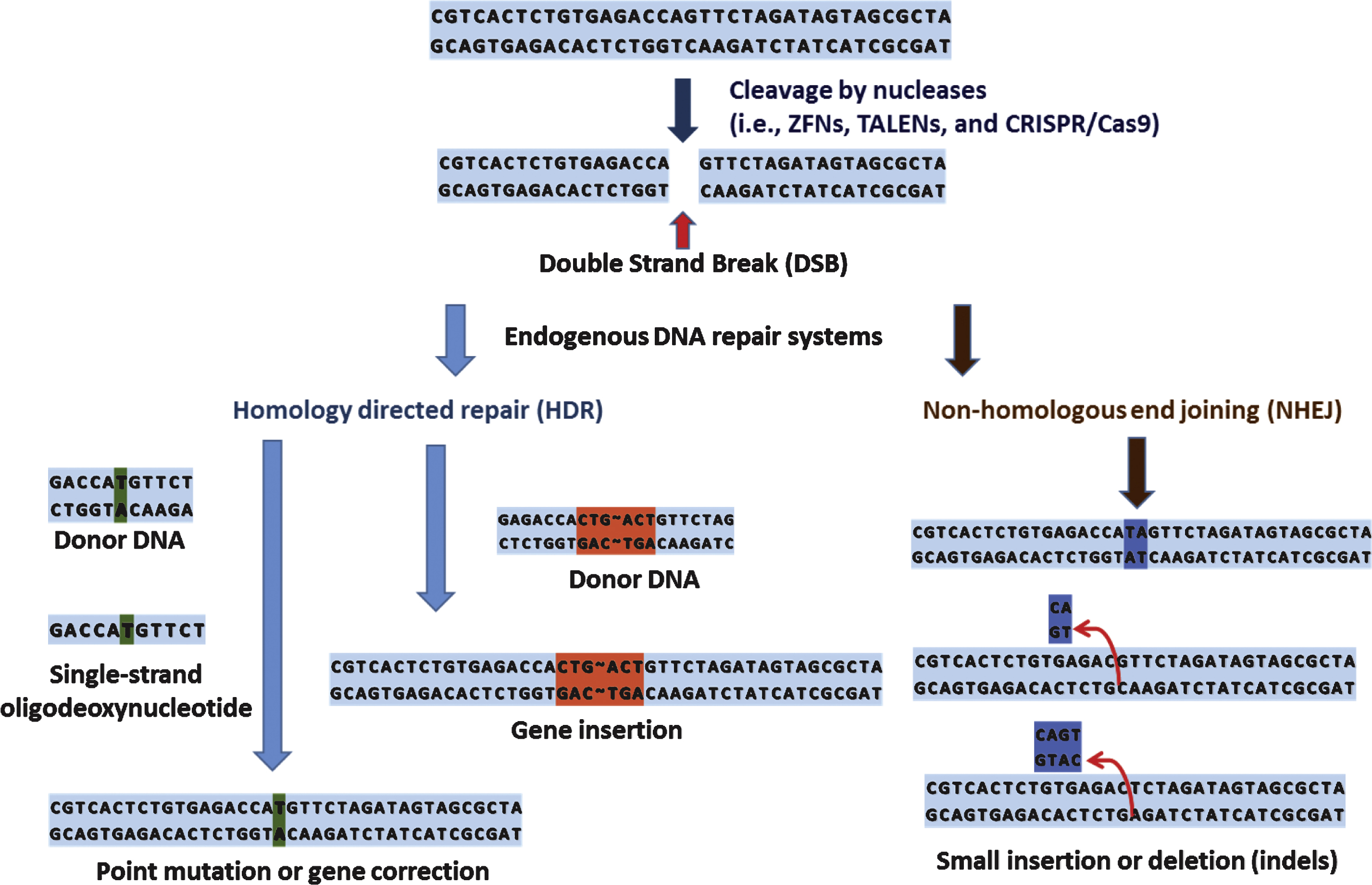
Fig.2
ZFN dimerize on the spacer sequence via sequence-specific interaction between ZFP and DNA. Amino acids inpositions −1, 3, and 6 on the α-helix recognizes and contact 3 base pairs in the major groove of DNA. FokI cleavage domains from each ZFN dimerize over the spacer sequence to facilitate DBS.
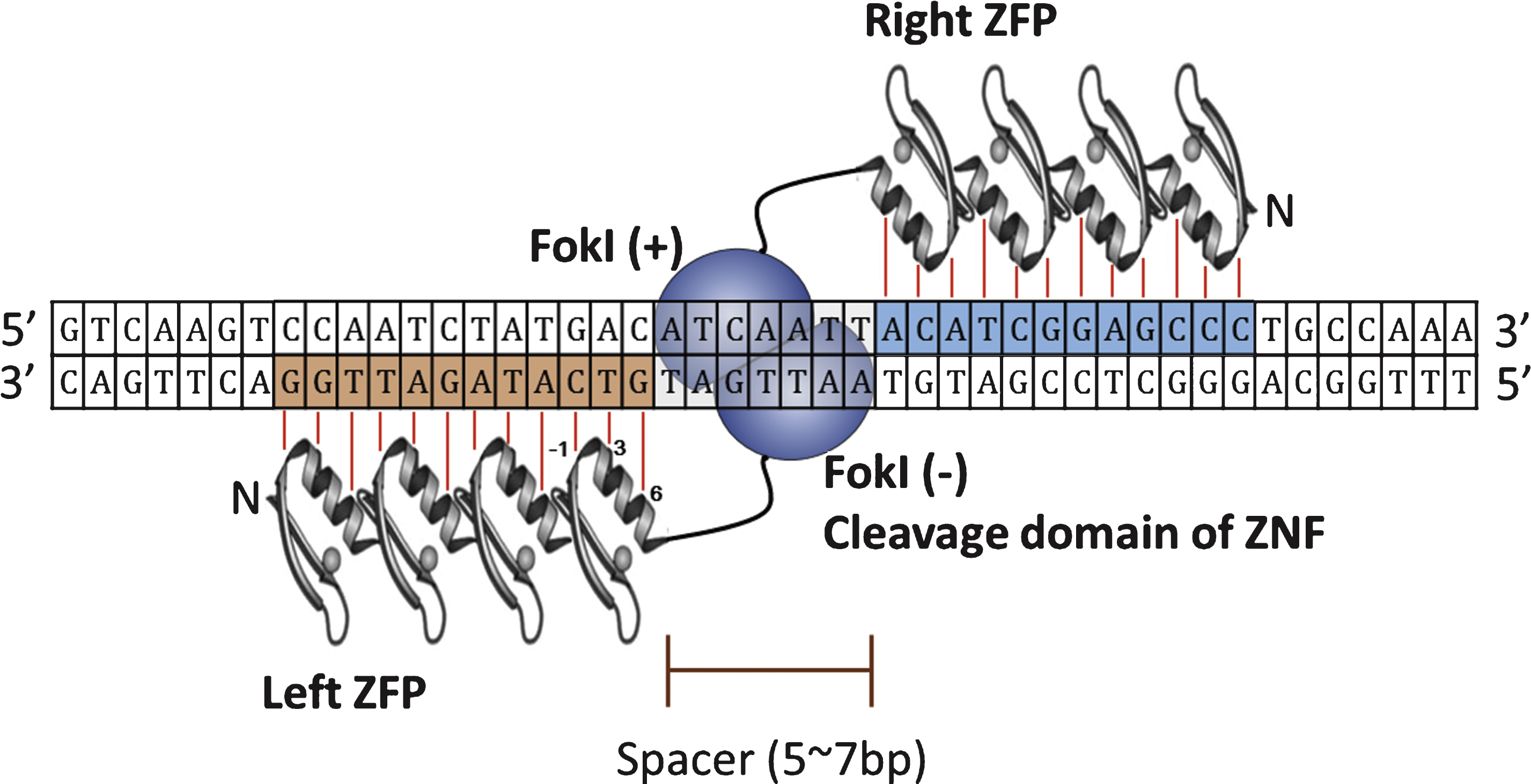
Fig.3
Structures of TALE and TALEN. A. Schematic presentation of TALE (TAL effector). Each TALE repeat contains 33–35 amino acids, and each RVD (amino acids at positions 12 and 13) recognizes a corresponding single base pair. RVD within a consensus repeat sequence is indicated in red. NLS: nuclear localization signal, AD: transcriptional activation domain. B. TALEN pair binds to specific DNA sequence recognized by TALEs. FokI cleavage domains from each TALE dimerize over the spacer sequence. In most cases, naturally occurring TALEs are preceded by T at the 5’-end [39] as indicated by red box.
![Structures of TALE and TALEN. A. Schematic presentation of TALE (TAL effector). Each TALE repeat contains 33–35 amino acids, and each RVD (amino acids at positions 12 and 13) recognizes a corresponding single base pair. RVD within a consensus repeat sequence is indicated in red. NLS: nuclear localization signal, AD: transcriptional activation domain. B. TALEN pair binds to specific DNA sequence recognized by TALEs. FokI cleavage domains from each TALE dimerize over the spacer sequence. In most cases, naturally occurring TALEs are preceded by T at the 5’-end [39] as indicated by red box.](https://content.iospress.com:443/media/jcb/2015/1-1/jcb-1-1-jcb15009/jcb-1-1-jcb15009-g003.jpg)
Fig.4
Schmatics of a generalized CRISPR locus. Upon introduction of foreign genetic elements from bacteriophages or plasmids, Cas proteins obtain spacers from the exogenous protospacer sequences and they are incorporated into the CRISPR locus of host genome.
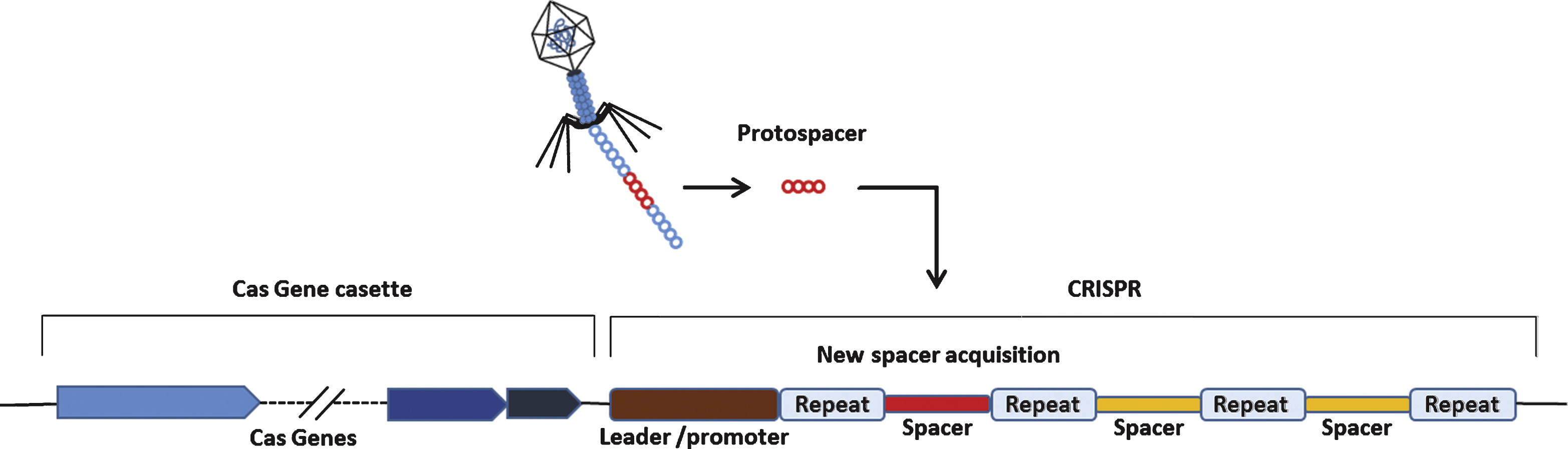
Fig.5
DNA cleavage by type II CRISPR nuclease system. In type II CRISPR, crRNA-tracrRNA hybrids complex with Cas9 to facilitate sequence-specific DNA cleavage. Filled triangle indicates cleavage by Cas ribonucleases. Small arrows indicate DNA cleavage by Cas9. sgRNA: single guide RNA.
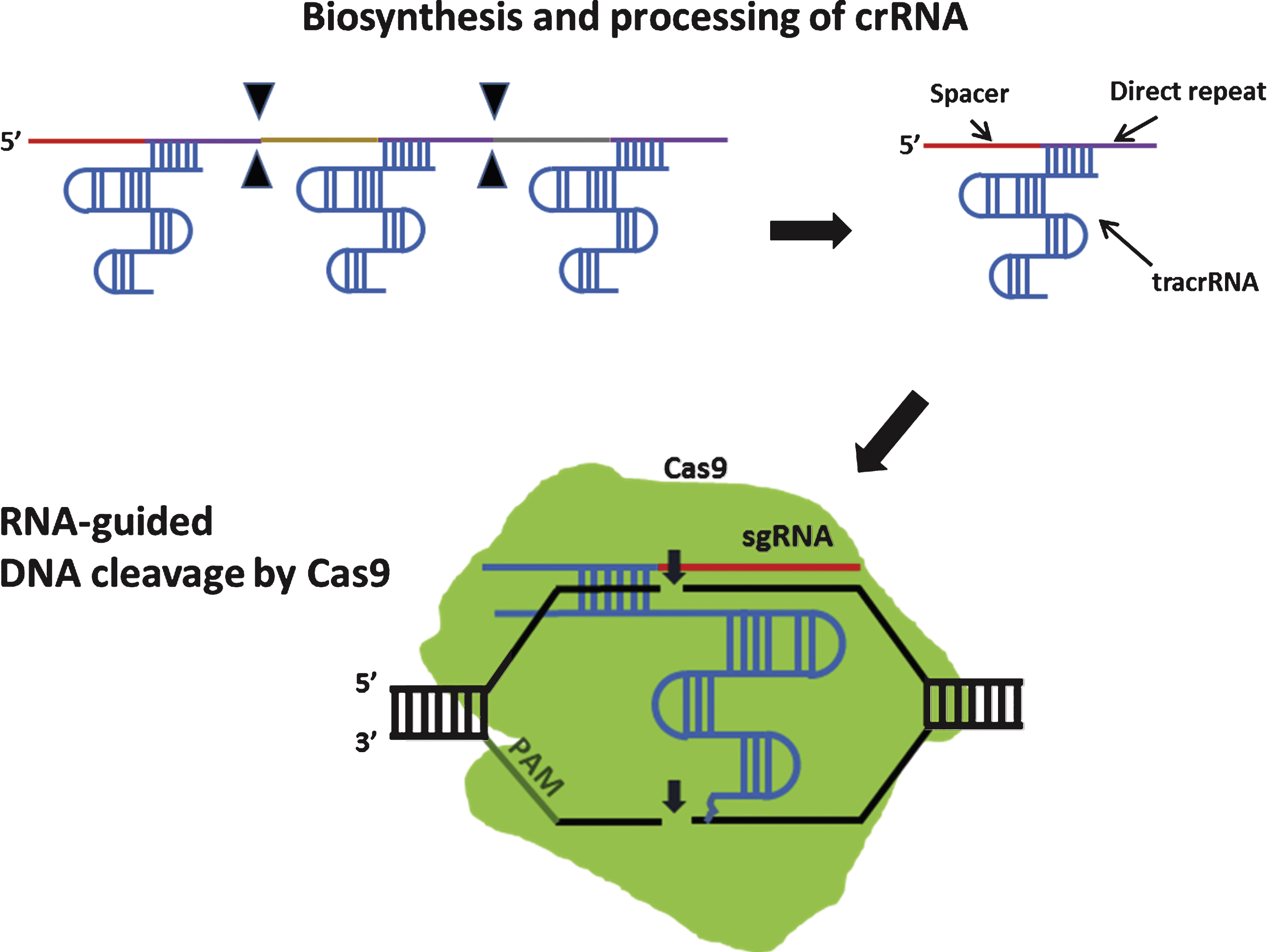
Fig.6
Example of genome editing utilized in personalized medicine.
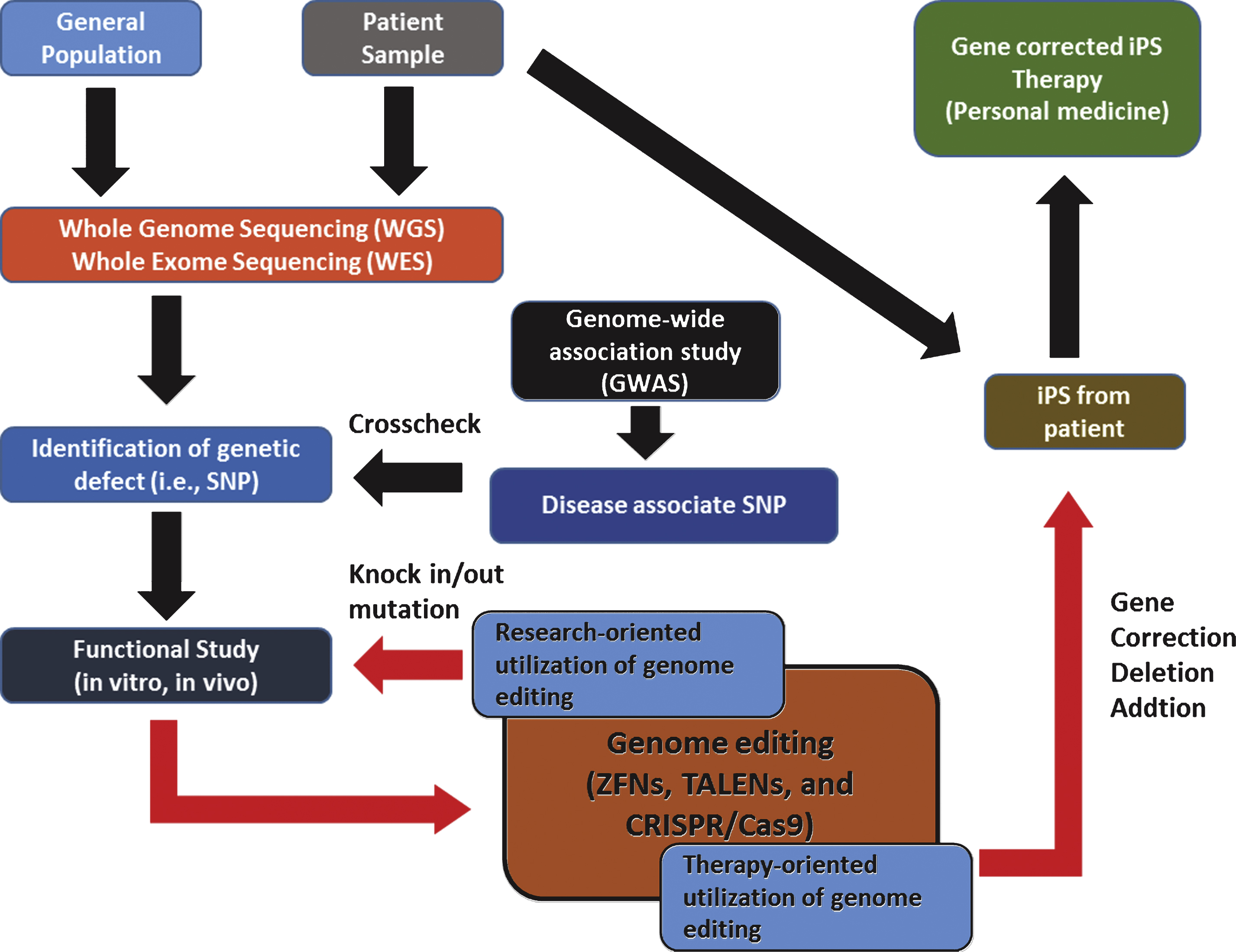
Table 1
Selected recent examples of ZFN-mediated genome editing in various organisms including human cells
Gene modification | Organism | Genes | Refs |
Disruption | Mouse | tbc1d20, rab3gap1, rab3gap2, rab18 | [24] |
Sheep | mstn | [25] | |
Human | MDR1, MRP2, BCRP | [26] | |
Human | HPV E7 | [27] | |
Humanized mouse | CCR5 | [28] | |
Addition | Cow | hLYZ | [29] |
Human | CCR5 | [30] | |
Correction | Human | AAVS1 | [31, 32] |
Human | HBB | [33, 34] |
Few selected, recent articles searched through Pubmed. The literature search was to overview the research trend regarding ZFN-mediated genome editing.
Table 2
Selected recent examples of TALEN-mediated genome editing in various organisms including human cells
Gene modification | Organism | Genes | Refs |
Disruption | Mouse | c-kit, PU.1 | [43] |
Zebrafish | nptx2a | [44] | |
X. tropicalis | TRα | [45] | |
Human | Rap1 | [46] | |
Human | Gads | [47] | |
Zebrafish | rspo2 | [48] | |
Mouse | ace2 | [49] | |
Zebrafish | fshb, lhb | [50] | |
X. tropicalis | dot1l | [51] | |
Human | miR-21 | [52] | |
Addition | Human | eGFP | [53] |
Correction | Human | DMD | [54, 55] |
Human | CCR5 | [56] | |
Human | HBB | [57–59] | |
Mouse | Crb1 | [60] | |
Human | XPC | [61] |
Few selected, recent articles searched through Pubmed. The literature search was to overview the research trend regarding TALEN-mediated genome editing.
Table 3
Selected recent examples of CRISPR/Cas-mediated genome editing in various organisms including human cells
Gene modification | Organism | Genes | Refs |
Disruption | Mouse | PrP | [73] |
Human | CCR5 | [74] | |
human | PVALB, EMX1 | [75] | |
Addition | Human | AAVS1 | [76] |
Correction | Mouse | Crygc | [77] |
Human | DMD | [54] | |
Mouse | DMD | [78] |
Few selected, recent articles searched through Pubmed. The literature search was to overview the research trend regarding CRISPR/Cas-mediated genome editing.