Pentapeptide WN5 targets the gut microbiota in alleviating Alzheimer’s disease pathologies
Abstract
BACKGROUND:
Antioxidant peptides have gained attention as potential therapeutic agents for Alzheimer’s disease (AD). The gut microbiota is also increasingly being recognized as central to AD progression and a potential therapeutic target for the disease.
OBJECTIVE:
Using a recently-identified antioxidant pentapeptide (Trp-Pro-Pro-Lys-Asn, WN5), we sort to test the hypothesis that dietary components could target the microbiota to inhibit Aβ aggregation and relieve AD-related cognitive impairments.
METHOD:
An Aβ42 aggregation cell model was employed to predict the ability of WN5 to inhibit Aβ aggregation. APP/PS1 mice were then used to explore the learning and memory-improving capacity of WN5 by targeting the gut microbiota.
RESULTS:
WN5 dose-dependently attenuated cellular Aβ-aggregation. Oral administration of WN5 (WN5_G) was associated with decreased microbial diversity and tended to impact the abundance of several major bacterial species associated with AD. The observed microbiota changes were significantly associated with reduced hippocampal Aβ aggregation (17.6±0.71 for WN5_G and 25.4±1.7 for the control group; p < 0.009) and improved cognitive performance. However, these observations were absent when WN5 was administered intraperitoneally (WN5_Ip).
CONCLUSION:
The results from this preliminary study suggested that WN5 could be useful in ameliorating AD-related symptoms via the gut-brain-axis and further emphasize the significance of the gut microbiota in AD.
1Introduction
Alzheimer’s disease (AD) is a neurodegenerative disorder of unknown etiology, characterized by amyloid-β (Aβ) plaque accumulation in the brain, resulting in progressive learning and memory impairment [1]. Indeed, all five drugs so far approved by the U.S. FDA for its treatment could only relieve the symptoms. The need for alternative mechanisms and agents for the treatment of AD have attracted tremendous research attention globally [2]. Drug and therapeutic agents for AD have tended to target Aβ aggregation [3] or the gut-brain-axis through the gut microbiota which has been reported to be strongly associated with pathological changes in AD in recent years [2]. Diet is considered one of the important modifiable risk factors in AD. Different dietary components such as antioxidants, vitamins, polyphenols, and peptides have gained enormous attention for their roles in the treatment of cognitive impairments [4, 5]. Indeed peptides have been shown to regulate memory through both peripheral and central functions [6]. Some anti-Aβ aggregation small peptides have already been shown to be effective in rodent and in silico models of AD and this had been attributed to their low molecular weight, high bioavailability, high selectivity, and low toxicity [7, 8]. Our research team recently identified a novel peptide (Trp-Pro-Pro-Lys-Asn, WN5) from walnut hydrolysate which demonstrated a strong antioxidant capacity [9]. Interestingly, antioxidant peptides are known to also inhibit Aβ aggregation [7]. Selecting an appropriate therapeutic target remains one of the key issues in the development of efficient AD therapuetics. Therapeutic peptides have often been administered by injection. However long-term vein injection in mice poses a big practical challenge. A more feasible alternative for long term treatment in mice would be intraperitoneal injection [10, 11]. It has been reported that on the one hand the oral administration of peptide drugs may be negatively impacted by the physiology of the gastrointestinal environment; on the other hand, gut microbial species may modify the structure of peptide drugs to enhance its efficacy [11]. Therefore using peptide WN5, we sort to test the hypothesis that dietary components could target the microbiota to inhibit Aβ aggregation and ameliorate cognitive impairments in AD.
2Methods and materials
2.1Synthesis of peptide PW5
The identification and purification of peptide WN5 was previously reported elsewhere [9]. In this study, WN5 was chemically synthesized by GL Biochem (Shanghai) Co., Ltd (Shanghai, China) using L-isomers of each amino acid by solid-phase synthesis via the fluorenylmethoxycabonyl (Fmoc) method. It was then stored at –20 °C for subsequent usage.
2.2Intracellular Aβ42 aggregation
2.2.1Cell viability
MTT assay was used to study the effects of peptide WN5 on the viability of HEK-293-E22G cells [12]. The HEK-293-E22G cell is a modified version of the Aβ42-mCherry florescent screen in vitro using Flpin-T-Rex HEK293 cell line to screen intracellular Aβ42 aggregation [13]. The E22G-mCherry gene HEK293 cells were stably transfected with E22G-mCherry gene, and thus are able to overexpress the Aβ-42-mCCherry protein stably. This model has the ability to mimic the rapid and aggressive amyloid fibril formation in cultured cell and the Aβ1–42 labelled with mCherry enable the Aβ1–42 aggregation to be observed and quantified in the study of amyloidogenesis [12]. HEK-293-E22G cells were seeded in a 96-well plate for 24 h and treated with 0.05 mM (WN5_L) and 0.5 mM (WN5_H) WN5 samples for 48 h. MTT solution was added to each well and incubated for an extra 4 h and detected at 490 nm by microplate ELISA reader.
2.2.2Image analysis
Intracellular Aβ-42 aggregation was monitored with IncuCyte ZOOM live cell imaging system (Essen BioScience, MI, USA) and images were recorded every 4 hours [12]. The experiment was prepared in triplicates and 9-fields of vision were photographed in each hole. Aβ aggregation rate was computed based on formula one (1) below.
(1)
2.2.3Flow cytometry
Flow cytometry analysis (FACScan flow, Beckhan, Sanjose, CA, USA) was performed to assess the red fluorescence Aβ–42 aggregate formation within the cell. Cells were seeded at a density of 2× 105 cells/well in a 6-well plate and treated with 0.05 mM and 0.5 mM WN5 samples for 48 h. MTT solution was added to each well and incubated for an extra 4 h and detected at 490 nm by microplate ELISA reader. Untreated cells were used as the vehicle control.
2.3Animal studies
6 months old specific pathogen-free (SPF) male APPSWE/PS1 ΔE9 (B6C3-Tg (APPswe,PSEN1dE9)85Dbo/Mmjax, also known as APP/PS1, stock No: 34829-JAX, https://www.jax.org/strain/004462) [14] transgenic mice were acquired from the Nanjing Biomedical Research Institute of Nanjing University (Certificate No. SCXK 2015-0001). Age-matched wild-type (WT) littermates were used as controls. APPswe/PSEN1dE9 mice are mice on a C57BL/6J background commonly available commercially through the Jackson Labs. These Transgenic mice carry two transgenes with AD-linked mutations; namely a chimeric mouse/human APP with the Swedish mutation and human PSEN1 lacking exon 9 (dE9), both of which are under the control of the mouse prion protein promoter. Mice were housed in single cages under 12 h light/12 h dark cycle at 22–24 °C and 45±5% humidity and allowed to feed ad libitum. All animal experimental procedures were carried out at the Laboratory Animal Institute of Jinan University (Guangzhou, China) in strict accordance with its institutional guidelines (permit number: 149 20170313184750).
2.3.1Treatment groups
Mice were allowed a week to acclimatize to the laboratory environment. Four experimental groups were set up: APP/PS1 transgenic mice group (n = 5, AD group), APP/PS1 mice orally administered with WN5 (n = 6, WN5_G group), APP/PS1 mice intraperitoneally administered with WN5 (n = 6, WN5_Ip group), and wild-type group (n = 5, WT group). The peptide treated groups were accordingly administered 400 mg/kg/day peptide WN5 for 12 weeks, starting at age 6 months. The WT and AD groups were given a daily dose of saline also for 12 weeks. Mice were sacrificed at 9 months old.
2.3.2Morris water maze test
Morris water maze and shuttle box tests were adapted to assess learning and memory impairment in the various treatment groups. The method used was as reported by Wang et al. [12]. Briefly, mice were placed in a circular, water-filled tank (47-inch diameter) in an environment rich with extra maze cues. A submerged platform was located in a fixed spatial location 1 cm below the water surface, independent of a mouse’s starting position on a particular trial. In this manner, mice needed to utilize extra maze cues to locate the platform. The marks of N, S, E, and W, on four directions of the tank wall, served as spatial cues with which the mice could learn of the location of the hidden platform. A white edible pigment was used to make it easy to trace the APP/PS1 mice in the water. Each mouse was given four trials each day for 6 consecutive days within 1 h after intervention using a fixed set of start location. The maximum trial length was 60 s and if mice did not reach the platform within the allotted time, they were manually guided to find it. On reaching the invisible escape platform, mice were left on it for an extra 5 s to observe the spatial cues in the environment to guide future navigation to the platform. The temperature of the water was monitored every hour so that mice were tested in water of 20–24 °C. The latent, escaping-times, and the time spent in the valid region (around the platform, 1.5 fold) were measured and used as the spatial learning ability of mice in the Morris water maze test.
2.3.3Shuttle box test
The shuttle box test was adopted to assess the learning ability of mice to avoid harmful stimuli. The method used was as reported by Wang et al. [12]. The test was performed in a black box consisting of two compartments separated by a black wall with a hole in the lower middle part. One of the two chambers was illuminated and the other was dark. The test was conducted for 7 consecutive days. Before the experiment, mice were placed into the apparatus for 5 min (habituation stage). Afterwards, the mouse received the training trial. When the mouse entered the dark compartment, it would receive the electric foot shock (36 V) through the copper grid floor. The step-through latency (latency) and the total time spent in the dark chamber (electric shock time) were measured as the memory capacity of the mice.
2.4Immunohistochemistry Analysis
“After 12 weeks of treatment, mice were sedated with pentobarbital and well-perfused with saline. Brain tissues were harvested, dissected and hemispheres fixed in 4% PFA for 24 h. Then the hemispheres were serially dehydrated with 70%, 80%, and 95% alcohol, 45 min each. This was followed by 3 consecutive dehydration treatments with 100% alcohol, 1 hour each. Tissues were cleared with xylene each time before the next alcohol treatment, 1 hour each, and then immersed in paraffin in each case also for 1 hour each, before finally embedding the tissue in a paraffin block. The paraffin-embedded tissue blocks were then processed into section (5μm) and mounted on positively charged slides for immunohistochemistry. Aβ immunohistochemical staining was performed according to the standard protocols: the rabbit anti-Aβ42 antibody buffer (1 : 1500, Abcam) was added onto the sections of the slides and incubated in a humidified chamber overnight. Primary antibodies were detected with biotinylated goat anti-rabbit IgG (1 : 200) in conjunction with the DAB kit coupled with diaminobenzidine substrate. Stained brain tissues were observed and captured with an Olympus IX-73 microscope. Quantification analysis of the hippocampal Aβ deposits was by the ImageJ software”.
2.5DNA extraction and 16S rRNA gene sequencing
Fresh fecal samples were collected before and after treatment and stored for microbial profiling. Bacterial genomic DNA was extracted from fecal pellets using the QIAamp DNA Stool Mini Kit (Qiagen, Duesseldorf, Germany) according to the manufacturer’s protocol. The samples were sequenced for 16 S rRNA genes, targeting the V3–V4 regions. The 16S rRNA gene sequences from the fecal samples were conducted and analyzed using the Majorbio I-Sanger Cloud Platform (www.i-sanger.com). Processed sequences were subjected to subsampled, open-reference, operational taxonomic unit (OTU) picking against Silva reference data base (Release 138, http://www.arb-silva.de), with unmatched reads clustered by 97% sequence similarity that resulted into OTUs using UCLUST. Differences in alpha diversity measurements were calculated in Quantitative Insights Into Microbial Ecology (QIIME, v 1.9.1) with a non-parametric t-test and FDR correction for multiple comparisons, and beta diversity differences were analyzed with the principal coordinate analysis (PCoA). Linear discriminant analysis (LDA) combined with effect size measurements (LEfSe) was used to investigate the bacterial taxa differentially represented between groups. GraphPad Prism 6 software was used to identify significant differences in bacteria relative abundances between groups using a one-way analysis of variance (ANOVA).
2.6Statistical analysis
Microbial population analysis was as described above. Other experimental results were expressed as mean±standard error of the mean (SEM). For multiple comparisons, one way ANOVA with Bonferroni corrections was generated for normally distributed data whiles a Krustal-Wallis test on ranks was performed for data that failed the normality test. GraphPad Prism 6 software was then used to generate graphical representations of the results. In all analyses, results were deemed significant at p < 0.05.
3.Results
3.1Peptide WN5 inhibited in vitro Aβ42 aggregation
We first studied the ability of a walnut pentapeptide, WN5 (Trp-Pro-Pro-Lys-Asn, WPPKN; Fig. 1A) to inhibit Aβ aggregation in AD in vitro (Fig. 1B). There was no obvious toxicity to the viability of HEK-293-E22G cells (Fig. 1C) pre-incubated with peptide WN5 at 0.05 and 0.5 mM (p > 0.05). Flow cytometry images (Fig. 1D) showed that WN5 (0.05 and 0.5 mM) significantly inhibited the formation of Aβ aggregates compared to the vehicle-treated model. Quantification analysis confirmed that the number of Aβ aggregates (Fig. 1E), total area of Aβ aggregation (Fig. 1F), fluorescence intensity (Fig. 1G), and Aβ aggregation rate (Fig. 1H) were significantly lower in the WN5-treated medium in a dose-dependent manner compared to the model (p < 0.05). Taking together, these data revealed that, peptide WN5 is capable of inhibiting the accumulation of Aβ aggregates in vitro.
Fig. 1
Peptide WN5 dose-dependently reduces Aβ42 aggregate formation in vitro. A.WN5 was derived from walnut and its chemical structure was used in this study. B. Schematic representation of the in vitro study. C. Cell viability of WN5 at 0.05 and 0.5 mM in HEK-293- E22G cells. D. Images of Aβ42-aggregate formation in HEK-293-E22G cells treated with WN5 or vehicle, capture with an IncuCyte Zoom live cell imaging system. From left to right: Model (AD) group, WN5 at 0.05 mM, and WN5 at 0.5 mM. E. Total number of Aβ42-aggregates in cells treated with 0.05mM and 0.5 mM WN5 or vehicle-treated. F. Area of total Aβ aggregate (μm2). G. Total objects integrated intensity (RFU× μm2/Image). H. Aβ42-aggregation ratio in cells after treatment with PW5 or vehicle.
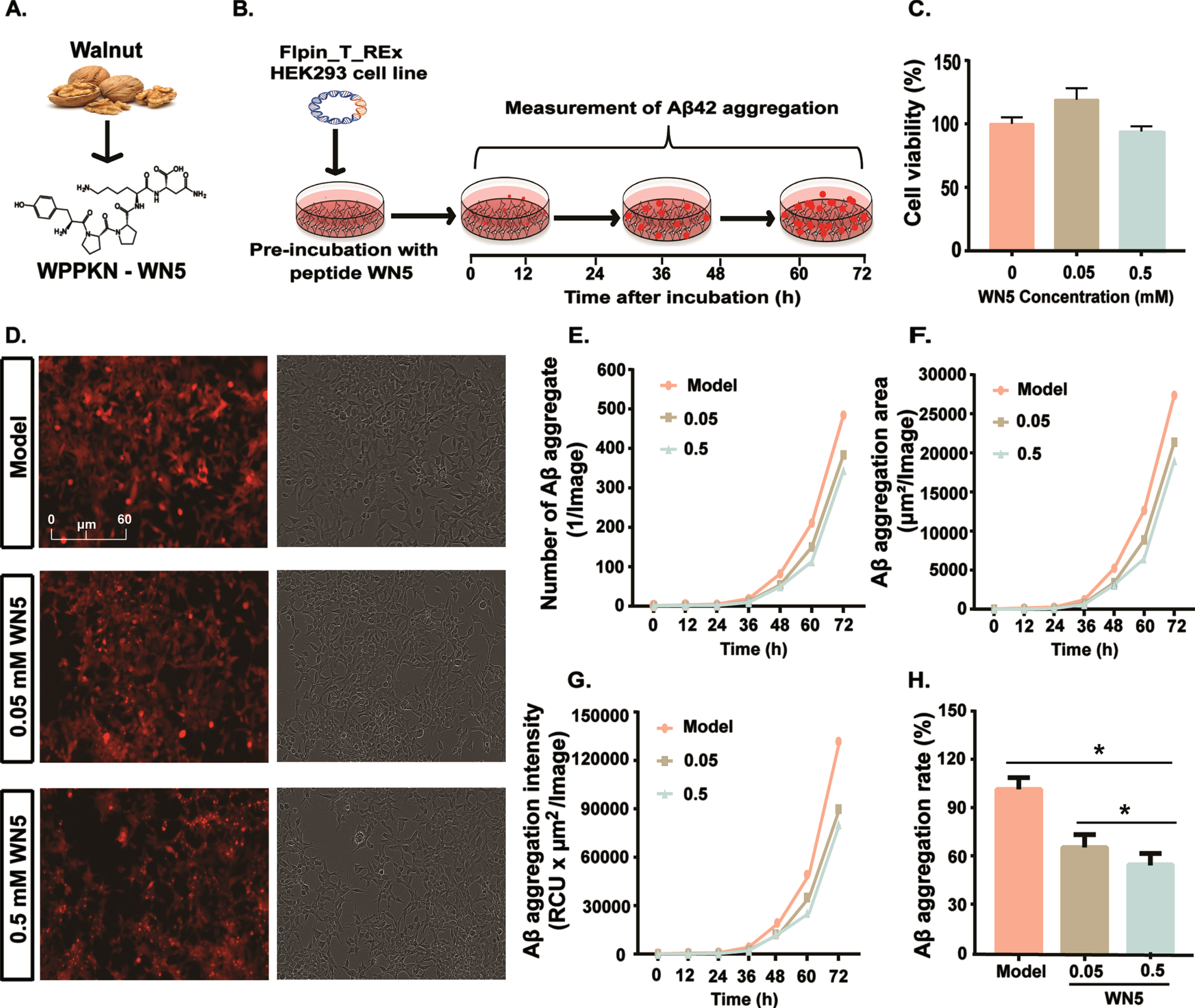
3.2WN5 ameliorated learning and memory impairment in APP/PS1 mice
APP/PS1 transgenic mice were randomized into 4 experimental groups as follows: APP/PS1 transgenic mice group (n = 5, AD group), APP/PS1 mice orally administered with WN5 (n = 6, WN5_G group), APP/PS1 mice intraperitoneally administered with WN5 (n = 6, WN5_Ip group), and wild-type group (n = 5, WT group). The peptide treated groups were accordingly administered 400 mg/kg/day peptide WN5 for 12 weeks, starting at age 6 months. The WT and AD groups were given a daily dose of saline also for 12 weeks. Learning and memory impairment were then assessed in the various treatment groups using the Morris water maze and shuttle box tests. Fig. 2A showed the schematic representation of the cognitive function study. From the Krustal Wallis test results (Fig. 2B), there were significant differences in escape latency among the groups, (chi-square = 9.259, p < 0.026, df = 3) with a mean rank score of 3.75, 16.17, 13.00, and 10.50 for the WT, AD, WN5_Ip AND wn5_G groups respectively. Pairwise comparison analysis revealed that the differences existed between the WT and AD groups (p < 0.018) but not the treatment groups. As expected, the WT mice spent more time in the platform quadrant to locate the platform compared with the AD mice (p < 0.010) and the WN5 treated mice also performed better than the AD group mice (p < 0.05) in this regard (Fig. 2C). In the shuttle box test (Fig. 2D), WT mice could remember the alarm sound and reacted often (13.58±2.34 times) to avoid the electric shock (active avoidance time) compared to the AD group (5.59±0.91times) (p < 0.001). Similarly, the number of active avoidance times in the WN5_G (8.24±1.04) mice was better than the AD model mice (p < 0.05) but not the WN5_Ip group (7.11±1.04). Correspondingly, the electric shock time (Fig. 2E) in the AD group (25.82±2.18s) was longer than the WT (6.1±3.12s) and the WN5_G groups (16.55±4.96s). In summary, these results showed that oral administration of WN5 improved AD-associated learning and memory impairment and these improvements were mild or absent when administered by intraperitoneal injection.
Fig. 2
Oral administration of WN5 ameliorates learning and memory impairments in APP/PS1 mice. A. Schematic representation of experiment design. B. Latency (s) in the Morris water maze test. C. Time spent in the valid quadrant in the Morris water maze test. D. Active avoidance times (n) in the Shuttle box test. E. Electric shock time (s) of the Shuttle box test. Statistical analysis was performed by one-way analysis of variance. WT and AD groups were given a daily dose of saline also for 12 weeks. WN5-Ip and WN5-G mice received a daily dose of 400 mg/kg/day peptide by intraperitoneal injection and gavage respectively. The data are presented as mean±SEM. WT: wild-type mice (n = 5), AD: non-treated APP/PS1 transgenic mice (n = 5), PW5-G: APP/PS1mice orally administered with peptide WN5 in APP/PS1 mice (n = 6), PW5-Ip: APP/PS1 mice intraperitoneally administered with peptide PW5 in (n = 6). (*p < 0.05; **p < 0.01; ***p < 0.001).
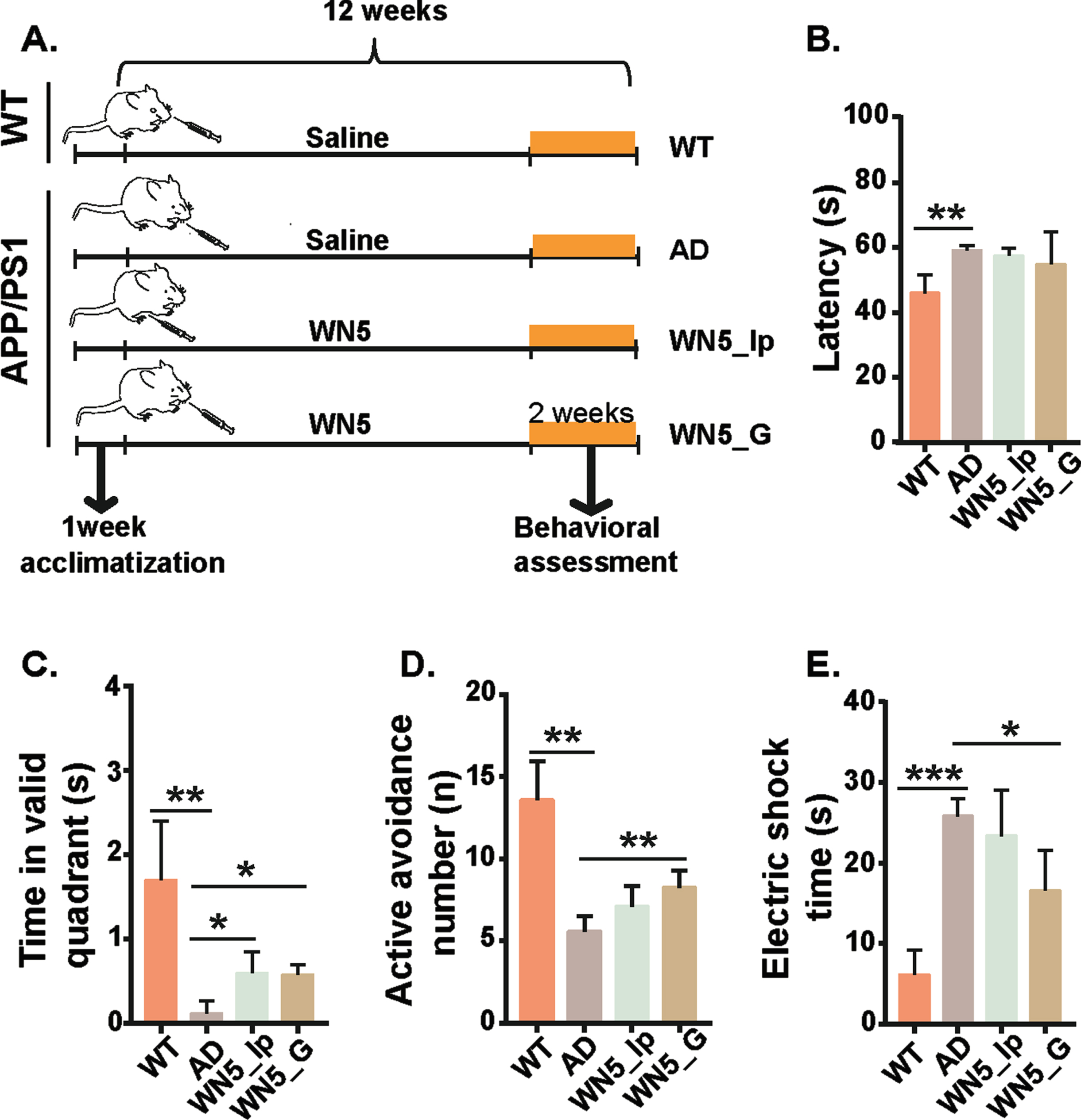
3.3Oral administration of WN5 reduced hippocampal Aβ plaques in APP/PS1 mice
We examined stained brain tissues of mice in the various treatment groups to ascertain the levels of Aβ plaque formation (Fig. 3A). From the immunohistochemistry (IHC) images (Fig. 3B), no obvious positive Aβ aggregates were seen in the WT mice. However, dense Aβ aggregates were observed in the AD mice and this was reduced in the WN5-treated mice group. The hippocampus is regarded to be more closely related to cognitive function; therefore, the Aβ load in the hippocampus was quantified (Fig. 3C). As expected, the Krustal-Wallis H test showed significant differences in Aβ-plaques among the treatment groups, (chi-square = 22.332, p < 0.001, df = 2), with a mean rank score of 29.23 for AD, 37.68 for WN5_Ip, and 14.71 for WN5_G groups. Surprisingly, there were significantly reduced Aβ-plaques in the WN5_G (17.6±1.7) compared to the AD group (25.4±1.7, p < 0.009) and WN5_Ip group (30.6±0.7, 0.001). However, no significant difference was observed between the AD and WN5_Ip groups (p > 0.05). It is also known that different sub-regions of the hippocampus (Fig. 3D) exert different cognitive functions and so we sort to analyze the level of Aβ aggregations in the CA1, CA2, and DG of the hippocampus. We found that there was no difference in Aβ plaque deposit in the CA1 region (Fig. 3E) between the AD (9.29±4.80) and WN5_Ip (13.89±5.16) mice but both groups had significantly increased plaque numbers compared with the WN5_G mice (4.86±3.65); (p < 0.011 for AD and p < 0.001 for WN5_Ip). In the CA2 region, however, (Fig. 3F), there was no significant difference in Aβ aggregation among the AD group (3.57±2.21) and WN5-treated groups but the WN5_G group (2.71±1.42) had significantly lower levels compared with the WN5_Ip group (4.67±2.91). Similar results were obtained for the DG region (Fig. 3G). Altogether these results implied that peptide WN5 could ameliorate Aβ plaque formation but only when administered orally and not intraperitoneally.
Fig. 3
Intragastric administration of WN5 alleviates Aβ plaque formation in APP/PS1 mice. A. Schematic representation of the experimental design. B. Images showing Aβ42 aggregates in stained brain tissues. Scale bar: (400μm). C. Hippocampal Aβ plaque load. D. Image showing the sections of the hippocampus. E. Aβ plaques in the CA1 of the Hippocampus. F. Aβ plaque load in the CA2 of the Hippocampus. G. Aβ plaque load in the DG of the hippocampus. Red arrows point to Aβ aggregates. WT mice developed no Aβ plaque aggregation. *p < 0.05; ** p < 0.01; *** p < 0.001.
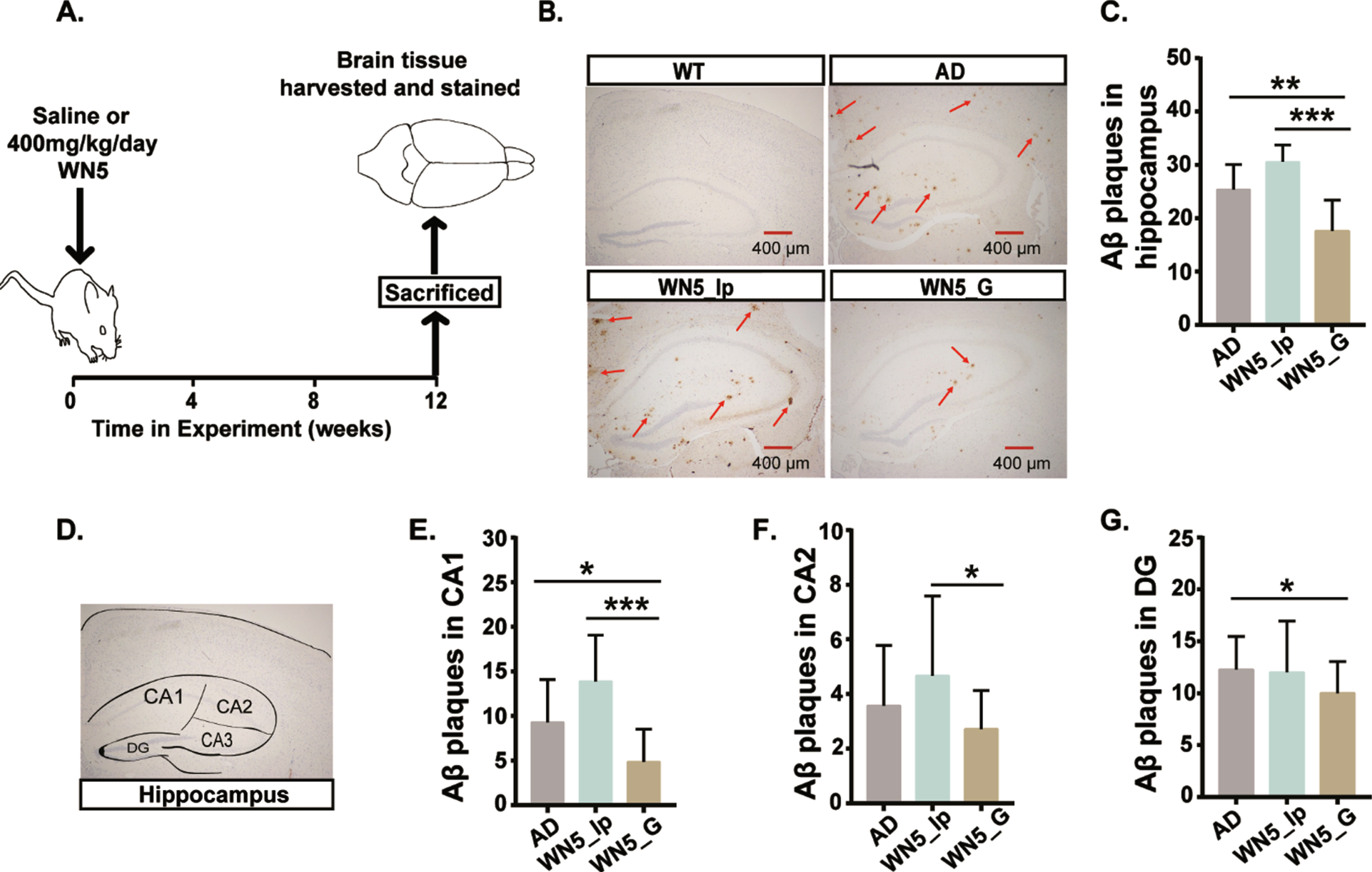
3.4Oral administration of WN5 significantly alters gut microbial species in APP/PS1 mice
The 16s rRNA gene sequencing analysis of fecal samples (Fig. 4A) showed that in the WT group, the bacterial population was dominated by Firmicutes (65.5%), Bacteroidetes (18.15%), Proteobacteria (6.1%), and Actinobacteria (4.1%) at the phylum level compared to 64.2%, 29.2%, 2.7% and 2.8% for Firmicutes, Bacteroidetes, Proteobacteria, and Actinobacteria respectively in the AD group at the beginning of the experiment. Furthermore, we observed a significant age-dependent shift in the gut microbiota composition between the WT and the AD mice. At the end of the treatment period (9 months old), we observed a decrease in Firmicutes (36.75%) and Actinobacteria (1.87%) with substantial increases in Bacteroidetes (17.70%), Proteobacteria (15.74%), and Verrucomicrobia (4.52%) in the AD group compared with decreases of 21.83% (Firmicutes) and 3.24% (Actinobacteria), and increases of 28.02% and 2.48% increase in Bacteroidetes and Proteobacteria, respectively, in the WT group at the phylum level (Fig. 4B). Interestingly, intragastric administration of WN5 was associated with a marginal increase in the abundance of Firmicutes (5.23%) and Verrucomicrobia (1.03%) and corresponding decreases in Bacteroidetes (1.57%) and Proteobacteria (4.86%) such that the gut microbiota structure at the phylum level resembled the WT group but substantially different from both the AD and WN5_Ip groups after the 12 weeks treatment (when mice were 9 months old). Alpha (α)-diversity analysis (Fig. 4C) revealed that diversity within treatment groups (before and after treatment) was not significantly altered except within the WN5_G group. However, the between-group analysis showed a significant decrease in diversity in the AD group compared to the WT (p < 0.05), whiles diversity in the WN5_G group was lower than the AD group and the WN5_Ip groups (p < 0.05). These implied that inter-group differences (between treatment groups at 9 months) in microbial composition were greater than the intra-group differences (within treatment group); and that oral administration of WN5 resulted in a significant alteration in microbial community numbers and evenness. Principal Coordinates Analysis (PCoA) showed no obvious separation in fecal samples between treatment groups along the PC1 (30.58%) and PC2 (16.23%) axis (Fig. 4D). However, a clear overlap involving WT and WN5_G groups was observed. Furthermore, LEfSe plot analysis displaying LDA scores of microbial taxa (Fig. 4E) revealed 20 bacterial genera were higher in abundance in the AD group compared to the WN5-treated groups (p < 0.05). These included f_Rikenellaceae (mean = 4.12; LDA = 3.69; p < 0.008), f_Erysipelotrichaceae (mean = 3.61; LDA = 3.24; p < 0.026), and f_Desulfovibrionaceae (mean = 4.52; LDA = 3.00; p < 0.014). From fig. 4F, it could be seen that f_Coriobacteriaceae was significantly higher in the WT group, and f_Erysipelotrichaceae, f_Enterobacteriaceae, and s_muciniphilia were elevated in the AD group. In the WN5-treated mice (Fig. 4G), 4 genera were highly elevated in the WN5_G group (c_Bacteroidia, f_Desulfovibrionaceae, f_Rikenellaceae and g_Helicobacter) whiles 6 were significantly higher in the WN5_Ip group (s_veronii, g_Pseudomonas, g_Halomonas, s_perfringes, c_Gammaproteobacteria and p_Proteobacteria). These results suggest that WN5 significantly alters the gut microbiota composition. Most importantly, oral administration of peptide WN5 was associated with a specific gut microbiota composition different from when it was administered by intraperitoneal injection.
Fig. 4
Intragastric administration of WN5 distinctively alters gut microbial structure in APP/PS1 mice. A. Schematic representation of the experimental design. B. Bacteria community plot showing percentages of the major bacteria at the phylum level. C. Alpha-diversity measured by the Shannon index. D. Principle coordinate analysis (PCoA) at OTU level before (mice at 6 months old) and after (mice at 9 months old) treatment based on the Bray-Curtis similarity. Mice were treated for 3 months. E. LEfSe analysis at all levels among the vehicle-treated APP/PS1 (AD) mice, intragastric WN5 treated APP/PS1 (WN5_G) mice and intraperitoneal WN5 treated APP/PS1 (WN5_Ip) mice. F. LEfSe at all levels among the Wild type (WT) group and vehicle-treated APP/PS1 (AD) mice. G. LEfSe at all levels among the intragastric WN5 treated APP/PS1 (WN5_G) mice and intraperitoneal treated APP/PS1 (WN5_Ip) mice. LDA: Least discriminant analysis.
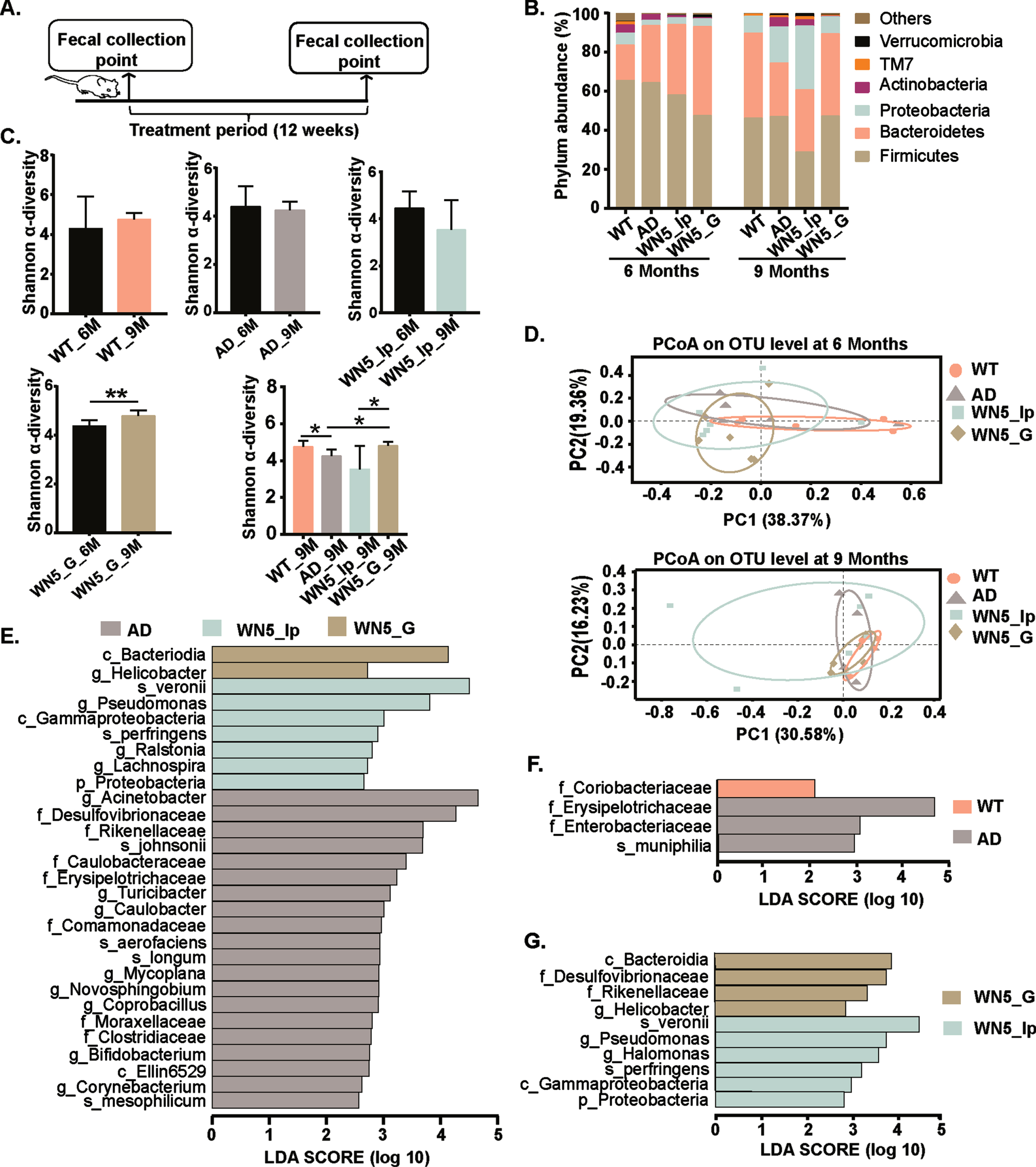
4Discussion
One of the major signature characteristics of AD is Aβ protein aggregation. Consequently, the formation, aggregation, and degradation of Aβ have become major targets in research and development of drugs and therapeutic agents for AD [15, 16]. In this regard, substances with high potency in inhibiting the aggregation or enhancing the degradation of Aβ are considered useful in the search for the prevention and/or treatment of AD [3, 14, 17]. We showed that walnut-derived peptide WN5 could potentially inhibit Aβ42 aggregation at both low (0.05 mM) and high (0.5 mM) concentrations without any obvious cytotoxic effect. This suggests that peptide WN5 could target Aβ aggregation in ameliorating cognitive impairments associated with AD. The use of synthetic Aβ for screening aggregation inhibitors is plagued with issues of high cost and inadequacies in their ability to mimic cellular Aβ aggregation. To overcome this hurdle, an anti-amyloidogenesis screening model was established by Kim et al. [18]. A modified version of the Aβ42-mCherry fluorescent screen in vitro using Flpin-T-Rex HEK293 cell line was developed by Lu et al. [13] to screen intracellular Aβ42 aggregation. This model relies on the fusion of Aβ42 with GFP such that in the absence of inhibitors, the entire fusion protein misfolds as a result of the rapid midfolding and aggregation of Aβ42 which then prevents fluorescence development. On the other hand, the presence of substances capable of inhibiting the aggregation of Aβ42 enables folding of GFP into its native structure thereby producing fluorescence signals which can be identified. This model does not only mimic the rapid and aggressive amyloid fibril formation in cultured cells but also the reporter protein, mCherry, tagged to AB42 as a reporter for fluorescence imaging allows the observation and quantitative study of amyloidogenesis. Thus, it allows for the rapid, efficient and cost effective means of screening compounds that are nontoxic and readily penetrate biological barriers [12]. The use of this model in the current study thus screened peptide WN5 as a potential inhibitor of intracellular Aβ aggregation and could therefore be used for further exploration in in vivo models.
Consistent with the in vitro results, we found that WN5 could reduce hippocampal Aβ plagues aggregation in APP/PS1 transgenic mice in comparison with the vehicle-treated APP/PS1 model mice. Furthermore, oral administration was associated with improved learning and memory impairments as measured by the Moris water maze and shuttle box tests. These improvements were only mild or absent when administered by intraperitoneal injection suggesting that the route of administration could be a critical determinant to the level of efficacy of WN5. Morris water maze and shuttle box tests are standard tests frequently used for the evaluation of cognitive function in aging and neurological conditions [19, 20]. Intraperitoneal injection introduces substances of interest directly into the bloodstream but the direct introduction of peptide drugs into the bloodstream may pose allergic reaction challenges [10, 21]. Besides, only small peptides (di- and tri-peptides) have been shown to have the ability to cross the blood-brain-barrier (BBB) to exert direct influence [22]. This suggested that the ability of WN5 to ameliorate cognitive impairment might be indirect, independent of its ability to cross the BBB. Interestingly, the most significant difference between oral and intraperitoneal administration is the involvement of the gut microbiota. Oral administration meant that WN5 is ostensibly introduced into the gastrointestinal tract where it may get structurally modified by gut microbial species, induce other agents or modify the gut microbiota structure which might potentially enhance its bioactivity including remote memory regulation [23, 24]. The evidence thus suggests that WN5 requires the transforming action of the gut environment in vivo. This may also explain the inadequacy of the intraperitoneal route that does not transform the peptide nor impact the gut microbiota structure. Interestingly, oral administration of various dietary components has been widely reported to impact both gut microbiota structure and function in diverse conditions [4, 25, 26]. The WN5-transforming action of the gut over time may also lead to significant changes in the structure and function of the microbiota. There is evidence that significant alteration in the gut microbiota strongly impact immunity and affect disease progression including neurological diseases [4, 27, 28]. In a novel idea for AD treatment, Shen et al. suggested that improving the gut microbiota composition in AD patients could further inhibit neuroinflammation and attenuate AD pathologies [29]. Numerous other findings suggest that gut microbiota affects the regulation of the gut-brain-axis through immunological, neuroendocrine, and direct neural mechanisms, implying that the role of the gut microbiota in regulating neuro-immune function beyond the gastrointestinal tract may have a significant effect on the neurodegenerative process especially in AD [4, 30–32]. For example, fecal microbiota transplantation from aged APP/PS1 to young mice led to increased Aβ deposition in the cortex and hippocampus of the young recipient mice [31], and showed that FMT-induced gut microbiota alteration was sufficient to significantly increase Aβ plaque deposition with accompanying changes in astrocytes morphology. In the same study, we showed that alterations in the abundance of bacteria genera such as f_Coriobacteriaceae, g_ Aldercreutzia, o_Erysipelotrichales and g_Clostridium may play significant roles in Aβ pathology in APPSWE/PS1 ΔE9 mice. Park et al. reported Coriobacteriaceae as one of the signature gut microbiota species in Tg-APP/PS1 mice [31, 33, 34]. In another study, we showed that the intake of Bifidobacterium Lactis Probio-M8 acted as a preventive therapy for AD by protecting against gut microbiota dysbiosis [14] Thus aging along with diet could lead to microbiota dysbiosis which contributes to the pathogenesis of AD [12]. APP/PS1 transgenic mouse model is a frequently used model for the study of AD pathogenesis including the gut microbiota [35] and a growing number of research findings have reported a strong linkage between the gut microbiota and brain function through the gut-brain-axis [36, 37]. Our data showed treatment-specific alterations in microbial communities. Both the WN5_Ip and vehicle-treated AD groups showed no significant intra-group variations in microbial community richness and evenness. Besides, not only did the AD mice have a distinct microbial profile from the WN5-treated groups but also WN5_G mice had different microbiota state from the WN5-Ip mice. Indeed at the end of the 12 weeks treatment period (when mice were 9 months old), PCoA results showed that the gut microbiota structure of WN5_G mice most closely resembled the WT status, with WN5_Ip being most distinct. Elevated levels of Firmicutes and Verrucomicrobia have been observed in aged transgenic AD mice. Increases in Proteobacteria, and Erysipelotichaceae, which favors inflammation and Desulfovibrionaceae, found elevated in our AD mice have been reported to influence cognitive function in other studies [37, 38]. Pseudomonas, an opportunistic pathogen was found elevated in the WN5_Ip group whiles Bacteroidia which is negatively correlated with AD [39] was in higher abundance in the WN5_G group. Taking together, our preliminary findings suggest that targeting the gut microbiota, using peptide WN5, could ameliorate cognitive impairments associated with AD through the gut microbiota-brain-axis.
In conclusion, we showed that walnut-derived pentapeptide WN5 showed potential for attenuating Aβ aggregation in vitro. In addition, intragastric WN5 administration was associated with a gut microbiota highly distinct from both intraperitoneal-administered and AD model mice; and this microbiota state was associated with reduced hippocampal Aβ aggregation and improved cognitive performance in APP/PS1transgenic mice. It could, therefore, be inferred that peptide WN5 can target the gut microbiota to indirectly ameliorate learning and memory impairments associated with AD through the gut-brain-axis, suggesting that the effect of WN5 is depended on the administration route. It is worth noting that the interpretation of the results is limited to compositional changes of the gut microbiota and falls short of functional metabolic readouts. Consequently, further studies would be needed to elucidate precise mechanisms of action of the impact of WN5 including metabolomics and/or proteomics as well as in vitro exploration of the biodegradtion of WN5 among others.
Acknowledgments
We acknowledge Prof. Alan Tunnacliffe (Department of Chemical Engineering and Biotechnology, University of Cambridge, Cambridge, UK) for gracefully providing the cell line used in this study.
Funding
Thiss project was supported by the Guangdong Province International Science and Technology Project (2022A0505050012), the Belt and Road, Foreign Experts Innovation and Talent Exchange Project (DL2022163004L), the Sino-Singapore International Joint Research Institute Project Phase II (201-A022002), the Industrialization Project of the Sino-Singapore International Joint Research Institute (201-A017001C) and the Young Foreign Talent Program (QN2022163004L).
The funding agencies had no role in the design; the collection, analysis and interpretation of data of the study as well as in the writing of the report; and in the decision to submit the paper for publication.
Conflict of interest
The authors have no conflict of interest to declare that are relevant to the content of this article.
Data availability
Data available upon request through the corresponding author.
References
[1] | Rogers G , Keating D , Young R , Wong M , Licinio J , Wesselingh S . From gut dysbiosis to altered brain function and mental illness: mechanisms and pathways. Molecular Psychiatry. (2016) ;21: (6):738–48. |
[2] | Shen L , Ji HF . Associations Between Gut Microbiota and Alzheimer’s Disease: Current Evidences and Future Therapeutic and Diagnostic Perspectives. J Alzheimers Dis. (2019) ;68: (1):25–31. |
[3] | Sharma S , Nehru B , Saini A . Inhibition of Alzheimer’s amyloid-beta aggregation in-vitro by carbenoxolone: Insight into mechanism of action. Neurochemistry International. (2017) ;108: :481–93. |
[4] | Bianchi VE , Herrera PF , Laura R . Effect of nutrition on neurodegenerative diseases. A systematic review. Nutr Neurosci. 2019: :1–25. |
[5] | Li C-H , Yang J-L . Wolfberry extracts inhibit Aβ1-42 aggregation and rescue memory loss of AD Drosophila. Food Science and Human Wellness. (2020) ;9: (1):64–70. |
[6] | Morley JE . Peptides and aging: Their role in anorexia and memory. Peptides. (2015) ;72: :112–8. |
[7] | Liu Y , Lin X , Li Q , Wang M , Zhou M , Wang Z , et al. Identification of two novel peptides with antioxidant activity and their potential in inhibiting amyloid-beta aggregation in vitro. Food Funct. (2019) ;10: (2):1191–202. |
[8] | Yu Z , Wu S , Zhao W , Ding L , Shiuan D , Zheng F , et al. Biological evaluation and interaction mechanism of beta-site APP cleaving enzyme 1 inhibitory pentapeptide from egg albumin. Food Science and Human Wellness. (2020) ;9: (2):162–7. |
[9] | Li Q , Kang X , Shi C , Li Y , Majumder K , Ning Z , et al. Moderation of hyperuricemia in rats via consuming walnut protein hydrolysate diet and identification of new antihyperuricemic peptides. Food Funct. (2018) ;9: (1):107–16. |
[10] | Permanne B , Adessi C , Saborio GP , Fraga S , Frossard MJ , Van Dorpe J , et al. Reduction of amyloid load and cerebral damage in transgenic mouse model of Alzheimer’s disease by treatment with a β-sheet breaker peptide. The FASEB Journal. (2002) ;16: (8):860–2. |
[11] | Ribarič S . Peptides as potential therapeutics for Alzheimer’s disease. Molecules. (2018) ;23: (2):283. |
[12] | Wang M , Amakye WK , Guo L , Gong C , Zhao Y , Yao M , et al. Walnut-derived peptide PW5 ameliorates cognitive impairments and alters gut microbiota in APP/PS1 transgenic mice. Molecular Nutrition & Food Research. (2019) ;63: (18):1900326. |
[13] | Lu M , Williamson N , Mishra A , Michel CH , Kaminski CF , Tunnacliffe A , et al. Structural progression of amyloid-β Arctic mutant aggregation in cells revealed by multiparametric imaging. Journal of Biological Chemistry. (2019) ;294: (5):1478–87. |
[14] | Cao J , Amakye WK , Qi C , Liu X , Ma J , Ren J . Bifidobacterium Lactis Probio-M8 regulates gut microbiota to alleviate Alzheimer’s disease in the APP/PS1 mouse model. European Journal of Nutrition. 2021: :1–13. |
[15] | Anand R , Gill KD , Mahdi AA . Therapeutics of Alzheimer’s disease: Past, present and future. Neuropharmacology. (2014) ;76: (Part A):27–50. |
[16] | Schartmann E , Schemmert S , Niemietz N , Honold D , Ziehm T , Tusche M , et al. In vitro potency and preclinical pharmacokinetic comparison of all-D-enantiomeric peptides developed for the treatment of Alzheimer’s Disease. Journal of Alzheimer’s Disease. (2018) ;64: (3):859–73. |
[17] | Lemere CA , Maier M , Jiang L , Peng Y , Seabrook TJ . Amyloid-betaimmunotherapy for the prevention and treatment of Alzheimer disease:lessons from mice, monkeys, and humans. Rejuvenation Research. (2006) ;9: (1):77–84. |
[18] | Kim W , Kim Y , Min J , Kim DJ , Chang Y-T , Hecht MH . A high-throughput screen for compounds that inhibit aggregation of the Alzheimer’s peptide. ACS Chemical Biology. (2006) ;1: (7):461–9. |
[19] | Sathyamoorthy Y , Kaliappan K , Nambi P , Radhakrishnan R . Glycyrrhizic acid renders robust neuroprotection in rodent model of vascular dementia by controlling oxidative stress and curtailing cytochrome-c release. Nutr Neurosci. (2020) ;23: (12):955–70. |
[20] | Cai H , Cai T , Zheng H , Liu L , Zhou L , Pang X , et al. The Neuroprotective effects of Danggui-Shaoyao San on vascular cognitive impairment: Involvement of the role of the low-density lipoprotein receptor-related protein. Rejuvenation Research. (2020) ;23: (5):420–33. |
[21] | Ribaric S . Peptides as Potential Therapeutics for Alzheimer’s Disease. Molecules. (2018) ;23: (2). |
[22] | Miner-Williams WM , Stevens BR , Moughan PJ . Are intact peptides absorbed from the healthy gut in the adult human? Nutrition Research Reviews. (2014) ;27: (2):308–29. |
[23] | Mandal A , Prabhavalkar KS , Bhatt LK . Gastrointestinal hormones inregulation of memory. Peptides. (2018) ;102: :16–25. |
[24] | Wang X , Zheng M , Liu J , Huang Z , Bai Y , Ren Z , et al. Differences of first-pass effect in the liver and intestine contribute to the stereoselective pharmacokinetics of rhynchophylline and isorhynchophylline epimers in rats. Journal of Ethnopharmacology. (2017) ;209: :175–83. |
[25] | Marungruang N , Kovalenko T , Osadchenko I , Voss U , Huang F , Burleigh S , et al. Lingonberries and their two separated fractions differently alter the gut microbiota, improve metabolic functions, reduce gut inflammatory properties, and improve brain function in ApoE-/- mice fed high-fat diet. Nutr Neurosci. (2020) ;23: (8):600–12. |
[26] | Miller AW , Orr T , Dearing D , Monga M . Loss of function dysbiosis associated with antibiotics and high fat, high sugar diet. The ISME Journal. (2019) ;13: (6):1379–90. |
[27] | Rajoka MSR , Shi J , Mehwish HM , Zhu J , Li Q , Shao D , et al. Interaction between diet composition and gut microbiota and its impact on gastrointestinal tract health. Food Science and Human Wellness. (2017) ;6: (3):121–30. |
[28] | Fröhlich EE , Farzi A , Mayerhofer R , Reichmann F , Jacan A , Wagner B , et al. Cognitive impairment by antibiotic-induced gut dysbiosis: analysis of gut microbiota-brain communication. Brain, Behavior, and Immunity. (2016) ;56: :140–55. |
[29] | Shen H , Guan Q , Zhang X , Yuan C , Tan Z , Zhai L , et al. New mechanism of neuroinflammation in Alzheimer’s disease: The activation of NLRP3 inflammasome mediated by gut microbiota. Prog Neuropsychopharmacol Biol Psychiatry. (2020) ;100: :109884. |
[30] | Giau VV , Wu SY , Jamerlan A , An SSA , Kim S , Hulme J . Gut microbiota and their neuroinflammatory implications in Alzheimer’s disease. Nutrients. (2018) ;10: (11):1765. |
[31] | Wang M , Cao J , Gong C , Amakye WK , Yao M , Ren J . Exploring the microbiota-Alzheimer’s disease linkage using short-term antibiotic treatment followed by fecal microbiota transplantation. Brain, Behavior, and Immunity. (2021) ;96: :227–38. |
[32] | Nagpal R , Mainali R , Ahmadi S , Wang S , Singh R , Kavanagh K , et al. Gut microbiome and aging: Physiological and mechanistic insights. Nutrition and Healthy Aging. (2018) ;4: (4):267–85. |
[33] | Park J-Y , Choi J , Lee Y , Lee J-E , Lee E-H , Kwon H-J , et al. Metagenome analysis of bodily microbiota in amouse model of Alzheimer disease using bacteria-derived membranevesicles in blood. Experimental Neurobiology. (2017) ;26: (6):369. |
[34] | Lin L , Zheng LJ , Zhang LJ . Neuroinflammation, gut microbiome, and Alzheimer’s disease. Molecular Neurobiology. (2018) ;55: (11):8243–50. |
[35] | Pascoe EL , Hauffe HC , Marchesi JR , Perkins SE . Network analysis of gut microbiota literature: an overview of the research landscape in non-human animal studies. ISME J. (2017) ;11: (12):2644–51. |
[36] | Zhang L , Wang Y , Xiayu X , Shi C , Chen W , Song N , et al. Altered gut microbiota in a mouse model of Alzheimer’s disease. Journal of Alzheimer’s Disease. (2017) ;60: (4):1241–57. |
[37] | Shen L , Ji H-F . Associations between gut microbiota and Alzheimer’s disease: current evidences and future therapeutic and diagnostic perspectives. Journal of Alzheimer’s Disease. (2019) ;68: (1):25–31. |
[38] | Bäuerl C , Collado MC , Diaz Cuevas A , Viña J , Pérez Martínez G . Shifts in gut microbiota composition in anAPP/PSS 1 transgenic mouse model of Alzheimer’s disease during lifespan. Letters in Applied Microbiology. (2018) ;66: (6):464–71. |
[39] | Zhuang Z-Q , Shen L-L , Li W-W , Fu X , Zeng F , Gui L , et al. Gutmicrobiota is altered in patients with Alzheimer’s disease. Journalof Alzheimer’s Disease. (2018) ;63: (4):1337–46. |