Dopaminergic Cell Replacement for Parkinson’s Disease: Addressing the Intracranial Delivery Hurdle
Abstract
Parkinson’s disease (PD) is an increasingly prevalent neurological disorder, affecting more than 8.5 million individuals worldwide. α-Synucleinopathy in PD is considered to cause dopaminergic neuronal loss in the substantia nigra, resulting in characteristic motor dysfunction that is the target for current medical and surgical therapies. Standard treatment for PD has remained unchanged for several decades and does not alter disease progression. Furthermore, symptomatic therapies for PD are limited by issues surrounding long-term efficacy and side effects. Cell replacement therapy (CRT) presents an alternative approach that has the potential to restore striatal dopaminergic input and ameliorate debilitating motor symptoms in PD. Despite promising pre-clinical data, CRT has demonstrated mixed success clinically. Recent advances in graft biology have renewed interest in the field, resulting in several worldwide ongoing clinical trials. However, factors surrounding the effective neurosurgical delivery of cell grafts have remained under-studied, despite their significant potential to influence therapeutic outcomes. Here, we focus on the key neurosurgical factors to consider for the clinical translation of CRT. We review the instruments that have been used for cell graft delivery, highlighting current features and limitations, while discussing how future devices could address these challenges. Finally, we review other novel developments that may enhance graft accessibility, delivery, and efficacy. Challenges surrounding neurosurgical delivery may critically contribute to the success of CRT, so it is crucial that we address these issues to ensure that CRT does not falter at the final hurdle.
INTRODUCTION
Parkinson’s disease (PD) currently affects over 8.5 million individuals worldwide and represents the fastest growing neurological disorder in terms of its prevalence [1–3]. The pathological hallmark of PD is the abnormal aggregation of α-synuclein in Lewy bodies and Lewy neurites. Pathological aggregates are characteristically found in the substantia nigra and several other brain regions but have also been reported in the heart [4], digestive tract [5, 6], and skin [7, 8], implicating PD as a systemic disorder. Aberrant basal ganglia circuit function drives a cardinal triad of motor problems, consisting of bradykinesia, resting tremor and rigidity, while more widespread neurodegeneration of the cerebral cortex and brainstem results in the classical non-motor manifestations of the disease [9, 10]. Specifically, death of A9 dopaminergic neurons has been identified as key to many of the core clinical features of PD [11], providing a discrete target for treating some of the motor features of PD. The anatomical localization of neuronal loss, coupled with current advanced differentiation protocols capable of generating A9-specific dopaminergic neuronal subtypes [12–14], posits PD as a treatable condition via cell replacement therapy (CRT). Current treatment for PD has remained unchanged for over 50 years, focusing on symptomatic relief of motor symptoms using drugs that target the dopaminergic network. More recently, high-level evidence has established deep brain stimulation (DBS) as standard of care for improved motor symptoms and quality of life in a subset of appropriately selected patients. None of these current treatments are considered to be disease-modifying, and limitations exist involving their long-term efficacy and side-effect profiles [15]. CRT involves the introduction of new neurons, in the form of midbrain progenitors, within the diseased host brain, to replenish dopaminergic signaling with a continuous biological therapy. This could present a valuable approach, with an alternative mode of action to overcome several limitations of current treatments, including the need for life-long pharmacological therapy or hardware maintenance.
Preclinical studies between 1979–1985 demonstrated the ability for fetal grafts of ‘dopaminergic neuroblasts’ to survive, integrate and mediate functional recovery when transplanted in 6-hydroxydopamine (6-OHDA)-lesioned rodent models of PD [16–19]. Open-label trials in the late 1980s provided proof of concept that human fetal ventral mesencephalic (hfVM) cells transplanted directly into the striatum could reverse some of the motor deficits in PD patients, allowing patients to be weaned off their medication [20, 21]. A lift on the ban of federally-funded research using human fetal tissue in the United States of America, with the election of Bill Clinton to the White House, led to two NIH-funded double-blind clinical trials evaluating the efficacy of hfVM grafts, published in the early 2000s [22, 23]. Despite some potentially positive outcomes, both trials did not meet their primary endpoint, failing to demonstrate significant benefit. Additionally, they reported for the first time the development of unexpected graft-induced dyskinesias. However, postmortem assessment of grafts and clinical follow up of patients involved in both open-label and NIH trials indicated long-term clinical improvement and graft survival [24–28], sustaining scientific interest within the field of CRT for PD.Several shortcomings in the NIH-funded clinical trials study design have since been identified and extensively reviewed elsewhere, including trial design, patient selection, graft preparation and delivery [29]. Of particular interest is the underexplored role that neurosurgical delivery plays in explaining the translational disparity between animal and human studies, as well as the differing outcomes reported by clinical trials over the past 30 years.
In animal studies, cell grafts are usually injected via a metal cannula, typically attached to a Hamilton syringe. While current neurosurgical delivery systems have proven to be effective for small-animal studies, several technical challenges have been encountered when attempting to scale-up to human trials [30]. As the field progresses, there is growing evidence that our current neurosurgical delivery instruments have significant drawbacks, which in addition to their limited preclinical testing, may contribute to translation failure in the clinical environment [30–33]. Compounding this challenge, many early human trials failed to document their delivery methods in detail, resulting in no universal consensus surrounding the optimal protocol for neurosurgical delivery of neural tissue to the central nervous system (CNS). Trials often differed on several key factors including cell delivery instruments used, graft preparation, neurosurgical approach, number of needle tracts and site of transplantation, making results difficult to interpret. These factors may explain discrepancies in reported outcomes.
Recent advances in stem cell technology and good manufacturing practice [34, 35] have reinvigorated interest from research groups and industry, resulting in global collaborations between academic centers, such as G-Force PD, and in several important clinical trials [34, 36, 37]. As our ability to genetically engineer and differentiate stem cells improves, it is crucial that the field does not overlook the challenges of neurosurgical delivery as these may lead to the failure of both ongoing and future clinical trials. In this article, we will review neurosurgical delivery of CRT for PD, detailing the cell delivery instruments employed and discussing the practical challenges underlying the effective surgical delivery of cell grafts. We also briefly describe some novel biological technologies being developed to enhance graft efficacy.
TECHNICAL CONSIDERATIONS FOR NEUROSURGICAL GRAFT DELIVERY
Clinical efficacy of CRT remains challenging, due in part to the difficulty of delivering grafted cells to target structures within the CNS. Cell delivery is complicated by the presence of the blood-brain barrier (BBB). Intrathecal injection is an established approach to bypass the BBB and novel delivery methods such as intranasal delivery [38] and ultrasound-mediated BBB disruption [39] have been reportedly used to deliver stem cells to the brain. However, the requirement to deposit cells within specific and deep brain structures for PD means the direct intraparenchymal injection of neural tissue remains the only clinically feasible approach for cell transplantation. Early studies used an open neurosurgical approach to implant adrenal medulla tissue, resting directly on top of the targeted striatal complex [40–51]. While demonstrating proof of principle, detailed clinical analysis and postmortem studies showed that this approach did not provide significant clinical benefit and was not associated with cell survival. Interest shifted to the adoption of a cell suspension approach utilizing hfVM grafts [21], consisting of partially dissociated hfVM tissue for stereotactic intracranial delivery. Cell suspensions are currently regarded as the ideal method in which to deliver cells to the CNS, and various neurosurgical delivery instruments have been developed to achieve this aim. We obtained information regarding instruments used in historic and ongoing trials with stem cell-derived grafts, providing valuable insight into some of the practical considerations underpinning effective cell transplantation (see Table 1). A high degree of variability was observed between trials, highlighting the lack of consensus regarding optimal neurosurgical delivery within the field.
Table 1
Characteristics of neurosurgical instrumentation employed in CRT studies utilizing hfVM or stem cell-derived grafts for PD (note that only studies where sufficient information was obtainable are included).
Trial (s) | Patient number (n) | Immunosuppression protocol | Instrument Description | Cell Source | Graft Location | Neurosurgical approach | Outer diameter | Outcome |
Lund series [26, 27, 78, 82, 83, 157–160] | n = 18 | Variable between studies | Rehncrona-Legradi Outer cannula with mandarin tip tightly fitted to inner cannula with adjustable piston rod | hfVM | Putamen +/– caudate | 3–8 implants per side | 1 mm to 2.5 mm (earliest iteration) | Variable outcomes seen, but overall clinical benefit achieved following transplantation |
TRANSEURO (NCT01898390) | n = 21 | Ciclosporin Azathioprine Prednisolone | hfVM | Bilateral putamen | 5 needle tracts per hemisphere placed into putamen | – | Ongoing | |
European STEM-PD (NCT05635409) | n = 8 | Basiliximab Tacrolimus Azathioprine Steroids (not specified) | ESCs | Bilateral putamen | 5 needle tracts per hemisphere placed into putamen (4 or 8 deposits/tract) | 1.2 mm | Ongoing | |
Freed and colleagues [161] | n = 1 | No immunosuppression | 17-gauge outer cannula with removable 19-gauge inner stylet. Stylet removed and replaced with preloaded 19-gauge infusion cannula | hfVM | Unilateral putamen and caudate | 10 needle tracts into putamen (6 tracts) and into caudate (4 tracts) | 1.5 mm | Beneficial effects seen in various motor function tests following transplantation |
Peschanski and colleagues [162] | n = 2 | Cyclosporin Azathioprine Prednisolone | Needle attached to 50μL Hamilton syringe | hfVM | Unilateral putamen +/– caudate | 3 needle tracts into putamen +/–1 needle tract into caudate | 0.8 mm | Benefit in daily activities and motor timed tests associated with increased F-Dopa uptake at graft site following transplantation |
Freeman and colleagues [163] | n = 4 | Cyclosporin | 100μL Hamilton syringe. Outer cannula (diameter 1.5 mm) attached to inner cannula (diameter 1.2 mm, tapering to 0.9 mm) | hfVM | Bilateral putamen | 6–8 needle tracts per side. Bilateral grafts thus between 12–16 total needle tracts | Graduated from 1.2 mm to 0.9 mm | Clinical improvement in UPDRS and Schwab-England disability score associated with increased F-Dopa uptake at graft site following transplantation |
Halifax series [88] | n = 8 | Not available | 50μL Hamilton syringe attached to custom microinjector system with 21-gauge cannula needle | hfVM | Bilateral putamen | 4 needle tracts made unilaterally. Bilateral grafts over 2 operations giving total of 8 needle tracts | 0.8 mm | No procedural related adverse effects seen on postoperative imaging. Increased F-Dopa uptake at graft site following transplantation |
Columbia NIH-funded trial [22] | n = 40 (20 for grafting) | No immunosuppression | Guide cannula containing removable rounded stylet, replaced with preloaded needle | hfVM | Bilateral putamen | 2 needle bilaterally, giving total of 8 needle tracts | Graduated from 1.5 mm to 0.6 mm | No improvement in overall global rating scores following transplantation when compared to sham |
NY STEM-PD (NCT04802733) | n = 12 | Not available | ClearpointSmartFlow Cannula (real-time intraoperative MRI) | ESCs | Bilateral putamen | 3 needle tracts bilaterally, post-commissural putamen | – | Ongoing |
CiRA/Kyoto | n = 7 | Oral Tacrolimus for 1 year then taper to stop over 3 months 5–10 ng/mL target trough value GE180 PET scans used to detect activated microglia before and after tapering tacrolimus | Leksell System Custom syringe, modified version of Hamilton syringe, manual Shortest tract to target 4–5 hours | Allogeneic iPSCs | Bilateral putamen | 3 tracts each side and 4–8 deposits per tract, cell spheres transplanted, 2.5 or 5 million per hemisphere | 1.1 mm | 7 patients transplanted, 2 years follow up results due in 2024 |
Schweitzer and colleagues [121] | n = 1 | No immunosuppression | Star Drive system base with Schweitzer injector attachment allowing mounted Hamilton syringe with a 22-gauge stainless steel cannula | Autologous iPSCs | Bilateral putamen | 3 needle tracts bilaterally, giving total of 6 needle tracts | 0.72 mm | Data suggests potential improvement in motor symptoms and quality of life associated with increased F-Dopa uptake at graft site following transplantation |
CiRA, Center for iPSC Cell Research and Application; ESCs, embryonic stem cells; hfVM, human fetal ventral mesencephalic; iPSCs, induced pluripotent stem cells; UPDRS, Unified Parkinson’s Disease Rating Scale.
Neurosurgical approach and graft location
The stereotactic principles applied for DBS surgery also apply to CRT, and the aim of surgery is to maximize accuracy and precision with sufficient target coverage and minimum deposition outside that zone, minimize the risk of systematic errors or complications, and achieve consistent and reproducible results for patients. Unlike some DBS or ablative surgeries, CRT patients do not require to be ‘woken up’ from anesthesia during the procedure. However, CRT patients should still be anaesthetized by experienced specialists in neuroanesthesia, and surgeries should be performed in high-volume functional neurosurgery centers to abide by the ‘getting it right first time’ or GIRFT principles [52]. The scope for re-do procedures in the advanced therapies such as CRT may be limited. Choice of anesthetic drugs and the route of administration for these medications can have a significant impact on cerebral physiology during and after surgery and may influence clinical outcomes [53–55]. Administration of prophylactic antibiotics at induction, meticulous skin preparation and surgical technique can minimize risk of infection [56–58]. We advocate a systematic approach to the surgery, such that if an error occurs it can be traced back systematically, audited and corrected. This includes measures to minimize cerebrospinal fluid egress which can cause brain shift and impact on the target accuracy. The surgical protocol should have the minimum number of steps required and avoid complexities that may not directly improve outcome. One such step may be direct intra-operative MRI-assisted visualization of the graft being deposited in the brain [59]. While this has been used for gene therapy approaches in PD, it is not currently known what impact MRI (or other imaging modalities such as CT) would have on cell grafts [60]. Neurosurgical centers delivering CRT should use a stereotactic targeting system that they have sufficient experience with, and we advocate frame-based and (distortion-corrected) MRI-guided surgery. Accuracy and precision should not be compromised in CRT simply because the putamen is a larger structure to target, and mini-frame (often incorrectly referred to as ‘frameless’) solutions should be used only if local surgical protocols for this technique are well established. The same applies for robot-assisted procedures for neurosurgical delivery of CRT.
Historically, cell grafts in PD have been placed over the striatum, especially the posterior putamen, as it is the target for innervation by nigral dopaminergic neurons and the site of greatest DA loss in PD. This was also due to early concerns surrounding the short distances (2–3 mm) that graft-derived neurons extend and reinnervate host striatum. However, studies have shown that grafts transplanted at further distances from the striatum can mediate functional recovery, for example, combined intranigral and intrastriatal grafts led to increased [18F]-DOPA uptake in the putamen and clinical improvement in motor function [61, 62]. Graft location is an important consideration as it can influence the cranial entry point and the number of needle tracts required to deliver cells. For example, neurosurgical access to the striatum can be achieved by either a frontal or parietal approach for grafts to be deposited bilaterally (Fig. 1). There are pros and cons of each, with the former requiring multiple tracts to cover a larger area of the putamen but with minimal risk to visual pathway fibers. Frontal trajectories are planned anterior to the motor area, which is located posterior to the coronal suture. Freed and colleagues allude to a transfrontal approach just above the frontal sinus which is different from the conventional (pre-coronal) frontal approach, and while this approach may reduce the number of needle passes and avoids the motor cortex, it is not the shortest route to the target and may be anatomically more challenging to navigate past the caudate nucleus to reach the posterior putamen [22]. The parietal approach could theoretically cover a larger proportion of the striatum in a single needle tract per hemisphere, but with a risk of injury to visual pathway fibers. A method to circumvent this risk may be to map those visual pathways with pre-operative MRI, prior to using a parietal approach. However, the patient may need to be positioned prone which has other technical challenges and risks including vision loss due to direct pressure on the eyes from positioning in prolonged infusions. Patient positioning and needle angulation are key factors, particularly if operating time is long, as they may lead to cell sedimentation and inconsistent delivery. A single trajectory depositing cells at intervals along the distal tract may not cover the entire target location due to the curved anatomical shape of the posterior putamen and longer trajectories may be more prone to target errors. A parietal approach has been described in gene therapy work in non-human primates [60], but not so far in CRT as recent CRT trials have favored the pre-coronal frontal approach perhaps due to familiarity and safety profile in general neurosurgery. In either approach, the cranial entry burr hole is planned over a gyrus and such that the needle would avoid blood vessels in sulci and avoid transgressing the ventricles (which may impact accuracy and precision). Although the risk of catastrophic hemorrhage is small, this risk increases with multiple needle tracts as well as the risk of infection and other complications. Furthermore, additional tracts can significantly increase the procedure time and the risk of systematic errors with more co-ordinates to process, and so there is a consideration for opportunity cost for PD patients who could otherwise have an established treatment such as DBS, despite its limitations, versus CRT.
Fig. 1
Optimizing neurosurgical delivery in an ideal cell replacement therapy paradigm for Parkinson’s disease.
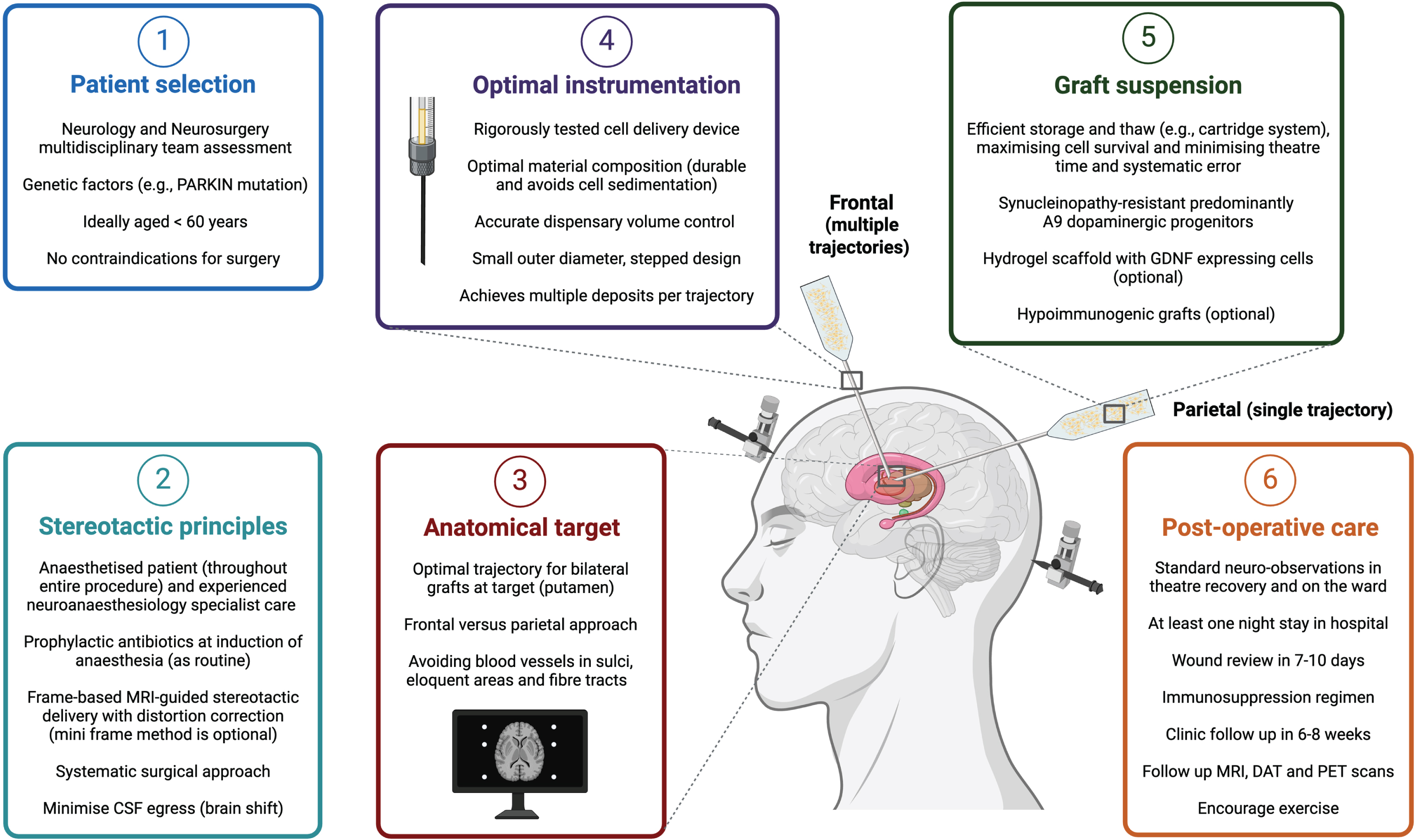
Cell number and injection volume
In preclinical rodent studies, a small single injection is often sufficient for graft delivery capable of mediating therapeutic benefit. As little as 650 surviving TH+ dopaminergic neurons have been shown to demonstrate behavioral and motor improvements in 6-OHDA-lesioned rodents grafted with hfVM tissue [63]. For previously grafted PD patients, postmortem analysis of those experiencing long-term relief of motor symptoms found a range of 43,000–240,000 surviving dopaminergic neurons present in grafts [22, 28, 64, 65], suggesting an approximate range for the number of dopaminergic neurons required for adequate long-term therapeutic striatal innervation. Given that the estimated survival rate for grafted midbrain dopaminergic progenitors is between 5–20% for fetal grafts [66], with a similar range seen in stem cell-derived grafts [35, 67], it can be estimated that somewhere between 200,000 to 5,000,000 cells are required to be grafted per patient per side for efficacious CRT. Beyond cell survival, it is also important to consider how fiber outgrowth and graft-host integration, may play a critical role in establishing the functional benefit of CRT [68].
In a primate model of PD (Macaca fascicularis), 16,000 surviving dopaminergic neurons were sufficient to produce a saturating effect in terms of behavioral recovery, which is in line with results of prior hfVM studies once the volume ratio between human and cynomolgus monkey is considered (equivalent to approximately 100,000 surviving dopaminergic neurons in humans) [69]. Indeed, the TRANSEURO consortium trial aimed to achieve approximately 100,000 surviving cells per graft to maximize chances of success [70]. To achieve this, delivery of a large number of cells is required. While delivering these cells by a single injection is theoretically possible, it would also likely impair graft viability, as has been demonstrated in a myoblast graft study in primates, which showed necrosis in large density grafts, likely due to limitations in oxygen and nutrient distribution in the center of larger grafts [71]. Large injection volumes have also been associated with several drawbacks [31] including greater reflux [72, 73], exposure to increased shear forces [74], damage to host tissue/bleeding [75], risk of embolism [76], and tumorigenesis [77]. Thus, most human studies for CRT in PD have used multiple injections for delivery of their grafts. It is also worth noting that in primates, transplanted grafts gradually increased in size for 6–9 months following transplantation, before plateauing at an average of approximately 40 mm3. This relatively small volume indicates that grafts are unlikely to cause significant mass effects in patients. Furthermore, optimal symptomatic relief from CRT may not be reached until nine months following transplantation, when graft outgrowth is maximal and synaptic connections are fully formed.
Needle diameter and graft size
The diameter of the delivery needle is another key consideration surrounding neurosurgical delivery of grafts, reflected by the optimization of instrumentation across various trials. The first open-label clinical trial utilizing hfVM grafts was performed as part of the Lund series in Sweden, with four advanced PD patients being recruited for CRT. For the initial two patients (patient 1 and 2), neurosurgical delivery of grafts was achieved using a specialized implantation instrument, comprising of two cannulas fitted together, with an outer diameter of 2.5 mm [78]. This is significantly larger than the current standard 1.3 mm DBS lead. The device was patented in 1988 and has since been described as the “Rehncrona-Legradi” instrument. Patients 1 and 2 demonstrated only minor improvement in clinical assessments, which the study authors believe may have been limited by instrumentation-mediated damage of the host striatum [78]. It has previously been shown that hemorrhage surrounding graft tissue during transplantation impairs neural allograft survival in preclinical rodent models [79, 80]. The large diameter (2.5 mm) of the instrument may have led to trauma during graft delivery, with post-surgical CT scans demonstrating a small hemorrhage at the implant site for patient 2. Furthermore, instrument-mediated damage at the time of transplantation may create a transient inflammatory microenvironment accelerating dopaminergic cell death and limiting efficacy of grafted tissue. Interestingly, delaying extrusion of graft tissue by one hour following the intraparenchymal insertion of the delivery needle, has been associated with significantly increased graft survival and functional recovery in a rodent model of PD [81]. However, such a delay may not be practical in the operating theatre. A 60% reduction in outer diameter of the Rehncrona-Legradi instrument to 1 mm was also associated with significant and sustained improvements in motor function for patients 3 and 4 of the series [82, 83], highlighting the importance of careful neurosurgical delivery for CRT success. Indeed, the ongoing TRANSEURO trial has utilized an adapted version of the Rehncrona-Legradi instrument to mediate graft delivery in the UK, while using the Rehncrona-Legradi device in the Swedish transplant arm of TRANSEURO in Lund. Both devices are manufactured in-house, with an adapted version used in the UK as the Swedish device is not currently CE marked (which indicates that a device meets established European Union requirements).
The significance of needle diameter has also been demonstrated in preclinical animal models, with the description of a micro-transplantation technique in 1994, which utilized a glass capillary attachment to the traditionally used Hamilton syringe cannula [84, 85]. Due to a reduction in outer diameter, multiple graft deposits are required to deliver the same total volume of grafted tissue. In a rodent model of PD, delivery of 450,000 cells (total volume of 3.6μl) was compared using either 2×1.8μl grafts delivered by a conventional steel cannula (outer diameter 0.5 mm), or 18×0.2μl grafts delivered using a glass capillary (outer diameter 50–70μM). Micro-transplantation demonstrated several advantages including reduced surgical trauma, more precise delivery, and enhanced visualization. Furthermore, the use of numerous micro-grafts improved graft survival and integration, highlighting that graft volume is an important consideration, and that multiple small grafts dispersed over a greater area improves efficacy [84, 85] and may be used to address issues surrounding adequate striatal reinnervation [86]. Interestingly, the direct comparison in 6-OHDA-lesioned rodents has shown that micro-transplantation is likely superior to two established delivery instruments used for human PD clinical trials (including the Rehncrona instrument), reinforcing a translational disparity that still exists between preclinical and clinical devices [87]. However, micro-transplantation uses a glass attachment, which currently has not been utilized in the clinic due to issues including the risk of fragmentation.
Minimizing implantation trauma using multi-graft instrumentation
While factors such as small injection volume, reduced needle diameter and multiple graft deposits are associated with improved graft outcomes, they also require a greater number of transcortical penetrations. With each needle pass through the parenchyma, there is risk of direct trauma or damage to surrounding architecture including blood vessels. To address this conflict, several neurosurgical instruments have been developed and tested to maximize the number of graft deposits, while simultaneously reducing risk of hemorrhagic complications. The only such instrument to have been tested clinically was described by Mendez et al. in 2002 and used in the Halifax Neural Transplantation program for a series of 8 PD patients. This device involved a 2-hole transplantation cannula (outer diameter 0.8 mm) allowing for the injection of two distinct graft deposits within 3 mm of one another, with each brain pass [88]. This distance has been shown to be within the theoretical range to enable inter-graft confluency [89–91]. In addition to promising results in animal studies, the use of the two-hole cannula in PD patients was not associated with any evidence of instrument-mediated hemorrhage or tissue damage for 16 transplantation surgeries totaling 64 trajectories [88]. However, it has been demonstrated that there is significant variation in the number of cells expelled between the first and subsequent aliquots for both horizontally and vertically orientated delivery approaches [92]. Thus, when using multiple cell deposits in a single needle tract, it is important to consider how uneven distribution of cells may impact graft survival andintegration.
Several other multi-graft devices have been developed and tested preclinically with the same principle of minimizing implantation-mediated trauma (see Table 2). One such device allowing for 12 graft deposits to be made through a single cannula injection was described and tested in rodents by Brecknel and Fawcett. They went on to adapt their device for human use, with an outer diameter of 1.2 mm capable of multiple concentric deposits, 10 mm from a single central needle tract. This would theoretically allow coverage of most of the human caudate/putamen with just two vertical injection tracts [86]. Another similar device capable of multiple graft deposits per cannula site was described by Cunningham and colleagues, additionally allowing for precise microvolume injections with electrophysiological recording during implantation. This device, with an outer diameter of 90μm, limits trauma and reactive astrogliosis local to the implant site in rodents [93] and has also been shown to be safe when targeting the substantia nigra of Göttingen minipigs [94]. Lim and colleagues have recently described another approach, involving the radially branched deployment of a delivery catheter, with an outer diameter of 1 mm. Following a single transcortical penetration, the delivery catheter enables real time adjustment of depth, rotation, and radial extension, allowing greater distribution of grafts within even larger target regions [30]. Improving distribution of grafts following delivery may also be explored using technologies such as convection enhanced delivery (CED), which utilizes continuous positive pressure to generate bulk flow distribution of macromolecules [95, 96]. Recently, an in-house CED system consisting of four microcatheters attached to a transcutaneous port was utilized to achieve chronic, non-invasive, and well distributed putamenal delivery of glial cell line-derived neurotrophic factor (GDNF) [97] and cerebral dopamine neurotrophic factor (CDNF) [98]. CED may be of relevance for CRT [30].
Table 2
Neurosurgical delivery devices developed with enhanced graft distribution evaluated in either preclinical or clinical models.
Described by | Instrument description | Largest model tested | Outer Diameter | Additional features | Outcome |
Brecknel and Fawcett [86] | Based on “extruding wire knife” design. Headpiece composed of 18G tube through which Teflon tubing and 22G cannula are threaded | Rodent | 1.2 mm (human prototype) | – | Device capable of implanting multiple cellular deposits through single injection site in rodent brain |
Cunningham and colleagues [93] | Carrier attached to guide cannula encasing a microcannula | Göttingen minipig | 148μm (human prototype) | Accurate microvolume injections Electrophysiological recording capabilities | Safe transplantation when targeting the substantia nigra of Göttingen minipigs |
Lim and colleagues [164] | Integrated catheter-plunger system with modular cannula system composed of outer and inner guide tubes, cell delivery catheter and plunger wire all nested together | Yorkshire swine | 1 mm | Real time adjustment of radial extension Integrated catheter-plunger system | Reduction in cellular reflux in comparison to a standard 20G straight cannula. Successful surgical delivery of multiple radially deployed graft deposits in Yorkshire swine |
Mendez and colleagues [88] | 50μL Hamilton syringe attached to custom microinjector system with 21-gauge cannula needle | Humans | 0.8 mm | – | Successful transplantation of multiple graft deposits into PD patients with no procedural related adverse effects seen on postoperative imaging. Increased F-Dopa uptake at graft site following transplantation |
Gill and colleagues [97] | Skull-mounted transcutaneous port attached to 4 microcatheters | Humans | – | CED system Capable of non-invasive chronic infusions | Device capable of delivery of multiple GDNF infusions directly over extensive putamen target tissue. However, GDNF infusion was not associated with improved clinical outcomes |
Svenningsson and colleagues [98] | Skull-mounted transcutaneous port attached to 4 microcatheters | Humans | – | CED system Capable of non-invasive chronic infusions | Implantation of delivery device was safe and capable of putamenal CDNF delivery. While CDNF delivery was safe and well tolerated, it was not associated with significantly improved clinical outcomes |
CDNF, cerebral dopamine neurotrophic factor; CED, convection enhanced delivery; GDNF, glial cell line-derived neurotrophic factor.
Graft loading
The process of loading graft suspension into a delivery cannula likely significantly influences the variability of CRT, impacting both the practicality and success of the procedure. If loading is to be performed by neurosurgeons in the operating theatre, it is important they possess adequate expertise in cell handling. Manual cell loading will impact graft variability, with both intradepartmental variability between neurosurgeons and interdepartmental variability between neurosurgical sites using different loading approaches. For the Kyoto trial, which utilizes a cell aggregation method, neurosurgeons found that if cells were loaded with a high volume of liquid they were seen to sink in the needle during preparation, resulting in a heterogeneously dense graft. Front-loading (drawing the cell suspension directly into the cannula via the tip prior to injection) may also present issues surrounding consistent delivery which may be minimized by a back-loading approach (priming a cannula with a prefilled syringe with cell suspicion). The development of an off-shelf pre-loaded cell “cartridge”, compatible with delivery devices and capable of being easily stored and transported, presents a potential approach to standardize CRT. Such a cartridge would remove the requirement to manually load cells, reducing theatre time, minimizing variability, and negating the requirement to train neurosurgeons in cell handling. Furthermore, such an approach would enable a means for large-scale quality-control. While such a cartridge has not been reported in the field of CRT, preloaded transport cartridges have been studied for anatomical replacement therapy for the delivery of Descemet membrane endothelial keratoplasty grafts [99].
Factors surrounding successful graft extrusion
Historically, neurosurgical delivery devices comprise of a straight cannula attached to an external syringe in which a plunger controls the rate of cell delivery. Such systems present several challenges that influence graft delivery. The role of mechanical forces on cells during injection has been extensively reviewed elsewhere [31]. It has been shown that an extensional force generated by differential velocities affecting the length of the cell is the most significant contributor to acute cell death during delivery [100]. Cells are particularly vulnerable to this mechanical force at the transition area of the instrumentation, where they travel from a large diameter syringe to a comparatively smaller diameter needle. Decreasing the outer diameter of the delivery cannula and keeping the syringe diameter constant will increase the magnitude of this extensional force, while lengthening the needle also increases the time cells are subject to this force [30]. Several strategies are being explored in response to address this issue, including the development of protective hydrogel cell carriers which have been shown to increase cell viability during the injections [100]. Interestingly, flow rate of the injection may also influence cell delivery, with too high a flow rate associated with greater mechanical damage and reflux [101, 102], and too low flow rates impairing cell delivery and leading to cell death [31, 103, 104].
Several issues also surround accurate volumetric delivery during transplantation. Typically, the use of an external manual syringe and plunger makes the delivery of precise microvolumes of grafts challenging. This may be addressed using pneumatic delivery devices, which allows accurate pulsed delivery of volumes as low as 3μl [105]. The remainder of “dead volume” within the delivery instrumentation and reflux of cell suspension back up the cannula tract can also significantly influence effective delivery and graft variability. While reducing the needle diameter [106] and “steeped” catheters [73, 96, 107] have both been associated with reduced reflux [95], Daniel Lim’s radially branched deployment device has an integrated catheter-plunger system, further eliminating the need for a separate syringe. By doing so, the device demonstrated a significant reduction in cellular reflux in comparison to a standard 20G straight cannula, and minimized dead volume. By designing the catheter-plunger system to be modular and fully removable, they are further able to address issues surrounding graft variability due to sedimentation within the syringe [30]. A novel columnar injection device allowing for separate control of syringe barrel and plunger has also been described by Schweitzer and colleagues, which utilizes the columnar cannula tract as the site of deposition during controlled cannula withdrawal. This technique is thought to reduce tissue resistance to delivered cells and improve graft surface area to volume ratio. When testing their device to transplant dopaminergic progenitors into rodent hosts, columnar injection demonstrated more uniform cellular distribution, fewer acellular areas and increased cell survival when compared with a traditional bolus injection technique [108].
A period of only twenty minutes between cannula filling and injection has been associated with significant cell sedimentation and retention [92], which is of particular relevance for neurosurgical delivery, where there is often a period of delay before the syringe can be attached to the stereotactic frame and cells injected within an identified injection plane [92]. The use of specialized low friction coatings such as silicone, as well as manipulation of factors influencing chemical and charge effects have also been suggested as a method to minimize cell adhesion. Interestingly, the influence of instrument material on cell retention within delivery devices has also been explored, with glass showing more retention than metal [92].
OTHER CONSIDERATIONS
Optimal patient cohort
Thus far, the patient response to grafted tissue in PD trials has returned mixed levels of success, with some patients demonstrating remarkable improvements in functional outcomes, while others showing little response or developing detrimental side-effects such as graft-induced dyskinesia [21, 29, 109]. This raises important questions surrounding the factors that predispose patients for successful CRT, and how to identify the optimal patient cohort for treatment. This prior selection would help support the use of resource-intensive neurosurgical intervention. As CRT is limited to the alleviation of primary motor symptoms caused by nigrostriatal degeneration, PD patients identified with a PARKIN mutation may represent ideal candidates for CRT due to their pathology being confined within the nigrostriatal pathway. Furthermore, these patients often lack an α-synucleinopathy pathology at postmortem, indicating their grafts may have greater protection from long-term α-Synuclein aggregation [110]. The Michael J. Fox Foundation has recently funded a phase 1b clinical trial to evaluate the safety and efficacy of dopamine progenitor cells specifically for treating patients with PARKIN mutations [111].
It is also well established that a loss of dopaminergic innervation occurs in various other brain structures, and the extent to which other regions are affected will be reflected in patients’ responses to grafting. Therefore, for CRT to be viable for a large population of PD patients, location of graft placement may become standardized and tailored to each individual patient, with graft response guided by data from [18F]-DOPA PET imaging. Furthermore, greater research is required to elucidate how factors such as patient environment, age and disease progression may affect the utility of CRT. Preclinical studies using grafts composed of ventral mesencephalic tissue [112, 113] or induced pluripotent stem cells (iPSC)-derived dopamine progenitor cells [114], have demonstrated that a younger brain likely provides a more receptive environment for successful CRT. Indeed, in one of the NIH-funded hfVM trials based in Columbia [22], potential clinical improvement following transplantation was only achieved in patients aged 60 and below. Younger patients are also more suitable for neurosurgical intervention with less comorbidities and fewer surgical risk factors. On the other hand, owing to ethical considerations, the FDA has historically recommended the recruitment of patients with more advanced stage PD into invasive trials, likely not in keeping with the ideal target population for CRT.
Interestingly it has been proposed that patients undergoing DBS may present an ideal patient population for future clinical trials evaluating CRT. Such a trial design would randomize patients to receive DBS and CRT concurrently, or just DBS alone. This presents several theoretical advantages, including negating the requirement of sham procedures, enhanced patient recruitment, cost reduction and evaluation of the synergistic effects of neuromodulatory and biological therapies [115]. However, such a trial has yet to be performed, likely owing to challenges surrounding complex surgical procedures and difficulties in evaluating the effectiveness of CRT.
Cell source
The use of hfVM grafts raised significant questions surrounding accessibility, as exemplified by the TRANSEURO trial, where only 21 surgeries were possible over a three year period because of inadequate tissue supply [34]. Further practical, ethical, and logistical hurdles including the requirement for stringent maternal screening, lengthy theatre time for harvesting and heterogeneity of grafts led to the pursuit of other cell sources. The ability to grow human embryonic stem cells (hESCs) and iPSCs [116], alongside the recent advancement in differentiation protocols enabling the generation of nigral (predominantly) A9 dopaminergic neurons from human iPSCs [13, 14, 117] has revitalized the field of CRT. It is important that an accessible and reliable tissue source is identified, to reduce the resource-heavy burden of CRT and prevent cancellation of planned neurosurgical procedures.
The various cell types, including stem cells, used to generate cell grafts for PD have been extensively reviewed elsewhere [118]. One advantage of patient-specific iPSCs is the ability to form autologous grafts, expressing the same surface markers as the hosts pre-existing neural circuitry, thus evading immune recognition, and theoretically negating the requirement for immunosuppression. Proof-of-concept was successfully demonstrated in a primate model of PD, where transplantation of autologous iPSC-derived midbrain dopaminergic neurons resulted in striatal reinnervation [119] and functional motor improvement without any immunosuppression [120]. Recently, a case report for the first human to be transplanted with an autologous iPSC-derived cell graft for PD was described by Schweitzer and colleagues. The patient was followed for 2 years after their first surgery, with data suggesting graft survival and potential symptomatic relief. Importantly, no adverse side-effects were reported, with no evidence of graft-induced dyskinesias commonly seen with fetal grafts [121, 122]. While this report is promising, it is important to note that autologous grafts may also have the same genetic risk factors predisposing PD pathology, and current hurdles involving their expense and time-consuming generation present financial and logistical barriers to their widespread adoption [123].
The use of human leukocyte antigen (HLA)-matched allogeneic grafts may represent a more feasible option, with evidence for this approach already having been demonstrated in primate models of PD [124]. These allogeneic grafts could theoretically be generated from “haplobanks” of iPSCs, donated by super donors who are blood type O and homogenous at HLA loci, resulting in immunological compatibility with large numbers of unrelated recipients. This allows for a potential off-shelf solution, substantially reducing associated costs and time for graft generation. The use of quality-controlled master allogenic cell lines would further standardize CRT, negating issues surrounding variability in cell culture and graft generation caused by heterogeneity in autologous source cells. These considerations were made pertinent in a 2015 RIKEN trial which aimed to investigate autologous iPSC-derived grafts to treat age-related macular degeneration. However, RIKEN has since revised their trial protocol to use allogenic grafts, in part due to the discovery of 6 mutations in a patient’s autologous iPSC line, one of which is listed as cancer somatic. These mutations were not detectable in the patients original fibroblasts, and thus may have been generated in culture [125, 126].
It has been estimated that 50 donor iPSCs lines would be adequate to generate HLA-matched tissue for 90.7% of the Japanese population [127] and progress is already underway to establish a global network of iPSCs haplobanks for cell therapies [128]. iPSCs obtained from the Clinical iPS Bank of Kyoto were used to create the allogeneic grafts transplanted by Takahashi and team in the first iPSC-based PD clinical trial launched by the Centre for iPSC Cell Research and Application, based at Kyoto University [129–131]. The use of iPSC haplobanks may provide a more reliable and less resource-intensive source of graft tissue for CRT, facilitating improved neurosurgical intervention.
Genetically engineering grafts
Advances in genetic engineering have provided tools to address some of the potential concerns surrounding the use of cell grafts in PD. It is possible to use CRISPR/Cas9 technology to generate “hypoimmunogenic” iPSCs, which possess deleted MHC class I and II genes and simultaneously over-express the immunoglobulin CD47, enabling them to evade immune destruction and potential graft rejection by natural killer cells. These cells can be differentiated into more mature cell types and successfully grafted in MHC-mismatched rodents without the need for immunosuppression [132]. However, engineering cells to avoid immune detection, while useful for grafting, does raise some safety concerns surrounding long-term use. Decreased HLA expression and overexpression of CD47 are well established methods by which cancerous cells evade the immune system, meaning transplanted cells may be at increased risk for malignant conversion [133]. Indeed, a major concern within the fields of regenerative medicine and cell transplantation is the avoidance of tumor formation long-term. Safety nets for transplanted cells are being investigated, including the genetic incorporation of suicide genes or “kill switches” that can be activated and expressed if the cell turns malignant by administration of drugs such as ganciclovir [134, 135]. Another concern with long-term graft survival is the susceptibility of the graft to develop the α-Synuclein pathology of PD. This has been detailed in the postmortem analysis of hfVM grafts older than 10 years, demonstrating varying degrees of α-Synuclein aggregation [28]. The generation of α-Synucleinopathy-resistant dopaminergic neurons from human iPSCs has been achieved with CRISPR-mediated deletion of the SNCA gene [136], providing another potential basis for enhancing long-term graft efficacy using genetic engineering.
Enhancing graft survival
Low dopaminergic neuronal survival and integration rates in grafts represents a major hurdle for successful CRT and autopsy data highlights the importance of surgical technique as one of the key factors [64]. Several avenues are being explored to optimize the growth capacity, survival, and efficacy of transplanted grafts. Prior to transplantation, treatment of human iPSC-derived dopaminergic progenitors with a modified extracellular matrix (Perlecan-conjugated laminin 511/521-E8 fragments) was shown to enhance maturation and neurite extension of grafted cells in rodents through activation of RAS-ERK1/2 pathway downstream of GDNF- and brain-derived neurotrophic factor-receptors [137]. Injectable biomaterial scaffolds, such as collagen hydrogels, can provide an optimal microenvironment for cell delivery and engraftment, while also enabling protective immune shielding [138, 139]. These scaffolds do not disrupt the local parenchyma and can be loaded with supportive growth factors such as GDNF, facilitating a 5-fold increase in the survival and re-innervation of encapsulated cells [139, 140]. Recent developments describing self-assembling peptide scaffolds and nanoparticle protein delivery vehicles has further advanced delivery of biomaterial scaffolds [141]. Co-transplantation of polymer-encapsulated genetically modified cell lines presents another novel avenue. Grafting modified cells overexpressing GDNF alongside dopaminergic neurons in a rodent model of PD enhances fiber outgrowth in the areas between the capsule and graft [142]. Delivery of either trophic factors such as basic fibroblast growth factor, neurturin and growth differentiation factor 5, or drugs such as lazaroids, caspase inhibitors and calcium antagonists have also been associated with increased graft survival as extensively reviewed by Brundin and colleagues [143]. Interestingly, cell maturity has also been shown to influence graft survival. Midbrain dopaminergic progenitors (day 17) demonstrate improved survival, fiber outgrowth and functional benefits when compared to immature neurons (day 24) and mature neurons (day 37) when engrafted in 6-OHDA rats [144]. Moreover, simple post-transplantation interventions such as exercise can also improve graft survival and integration [145].
Active modulation of grafts following delivery
While imaging modalities such as magnetic resonance imaging (MRI) and positron emission tomography (PET) are available to monitor graft survival and proliferation, it is crucial to explore questions surrounding post-transplantation regulation of grafts in patients and whether it is possible to modulate grafted cells in “real-time”. Can we control the level of dopamine produced by cells once grafted and prevent potential dopamine excess leading to graft-related side-effects? Developments in the novel fields of optogenetics and chemogenetics provide a theoretical method to directly switch grafted cells “on” and “off”, modulating their dopamine production in vivo. Theoptogenetic inhibition of transplanted hESCs-derived cells in a mouse model of PD has already been demonstrated, switching “off” dopaminergic production and causing the return of previously rescued motor deficits [146]. Chemogenetic tools such as DREADDs (designer receptors exclusively activated by designer drugs) could also be used with transplanted cells allowing for functional excitatory and inhibitory “switches”, without the need for specialized lasers and implanted fiber optics [147]. The development of these novel technologies and their implementation with established imaging modalities such as [18F]-DOPA PET, may provide an exciting tool to exogenously and non-invasively modulate graft efficacy and therapeutic outcomes following CRT. Such approaches may limit the requirement for further neurosurgical interventions and provide more long-term therapeutic benefit in traditionally treatment-resistant cohorts without the need for daily medication.
CONCLUSION
CRT has historically been labelled by some clinicians as a ‘failed therapy’ for PD; however, in view of the reasons highlighted above, including advances in cell source and graft biology [12, 148, 149], it may re-emerge as a potential treatment option that overcomes the limitations of current medical and surgical treatments for motor symptoms in PD [15]. Successful global clinical translation will depend on consistent neurosurgical delivery. As highlighted in this review and summarized in Fig. 1, the key factors to consider are patient selection, a systematic surgical method applying principles of stereotactic surgery, the anatomical target and surgical approach, a rigorous delivery system for consistent graft transplantation and survival, as well as meticulous post-operative care. Patient selection is crucial in functional neurosurgery and consideration for CRT will follow a similar multidisciplinary paradigm as currently used for DBS [150]. Over time, as the risk and benefit profiles become apparent for CRT, a broader range of PD patients may be considered for CRT.
An ideal delivery device should be rigorously assessed in large animal models, demonstrating both safety and efficacy. To minimize implantation-related trauma, the instrument should have a small needle diameter and be capable of multiple graft deposits with each brain passage. Furthermore, the device may incorporate a steeped catheter design to minimize reflux and maximize graft distribution. The ideal transplanted graft will be generated according to good manufacturing practice from ethically sourced ESCs or iPSCs, and genetically engineered to minimize immunoreactivity and reduce susceptibility to long-term tumorigenesis and α-Synucleinopathy. Furthermore, grafts may also be capable of post-transplantation modulation via suicide genes and/or optogenetic or chemogenetic modulation to control dopamine release [146, 147]. Grafts may be delivered within hydrogel scaffold microenvironments capable of proving trophic factor support to maximize graft integration and survival [139, 140].
While it is not possible for every neurosurgical center in the world to use the exact same instruments and methods for delivery, the emphasis should be on using a systematic approach that is well-established locally for consistent results. Experienced, high-volume functional neurosurgery centers audit and reaudit practices to refine methodology for consistent results and unlike DBS where switching on the current can give an immediate indication of response, in CRT it would take time for engraftment of cells and the clinical response to occur. Surgical theatre time can be optimized based on the number of planned trajectories, the delivery system, and a pragmatic ‘off the shelf’ intraoperative solution for thawing, loading, and injecting the grafts, with assistance from trained scientific personnel. Postoperative care including blood pressure management, wound care and pain management are also essential.
Several other alternative advanced therapies for PD are currently being explored, including intraparenchymal infusion of trophic factors [151] and gene therapy [152]. Recent clinical trials for these treatments have used novel intraoperative delivery modalities, demonstrating effective neurosurgical delivery. These include the use of a skull-mounted transcutaneous port with implanted catheters to facilitate long-term intermittent infusions of GDNF [97] and CDNF [98], as well as the combination of a steeped catheter, CED and real-time MRI imaging for delivery of AADC gene therapy [153]. However, these approaches are still far from incorporation into standard clinical practice and some aspects such as CED and real-time MRI imaging be less useful for CRT. A future approach for PD will likely use relevant multiple modalities to maximize success. Proof-of-concept of combination therapies was demonstrated by promising preclinical results of a recent study investigating GDNF infusions in conjunction with homotopic cell grafts [154, 155]. The use of “hybrid” stereotaxic surgery, merging DBS with stem cell implantation to harness a synergistic approach, has also been proposed [156].
Results from current phase I stem cell-derived dopaminergic cell replacement trials in PD will be crucial in determining the future of CRT. If the surgeries are expensive (personalized versus off-shelf), time consuming, error prone and return variable results, the opportunity cost of CRT in comparison to current well-established neurosurgical modalities such as DBS may be brought into question. In this case, it would be challenging to ethically design a CRT versus DBS clinical trial to confirm long-standing benefit relative to the current gold-standard surgical care. Thus, we must not falter at the final hurdle which is the non-trivial neurosurgical delivery of CRT.
ACKNOWLEDGMENTS
The authors would like to thank Professor Roger Barker, Professor Anders Björklund, Professor Tilo Kunath, Professor Thomas Foltynie and Professor Robert Brownstone for their critical comments on the manuscript.
FUNDING
A.N. is funded by the NIHR. S.K.K. is supported by the R.R Tasker Chair in Stereotaxic and Functional Neurosurgery.
CONFLICT OF INTEREST
S.K.K. declares honoraria and/or consulting with Abbott, Boston, Medtronic and Novo Nordisk. S.K.K. is a University of Toronto site co-investigator for the Bluerock Therapeutics trial (MSK-DA01). S.K.K. is an Editorial Board Member of this journal but was not involved in the peer-review process nor had any access to any information regarding its peer-review.
REFERENCES
[1] | GBD 2016 Parkinson’s Disease Collaborators ((2018) ) Global, regional, and national burden of Parkinson’s disease, 1990– 2016: A systematic analysis for the Global Burden of Disease Study 2016. Lancet Neurol 17: , 939–953. |
[2] | Ou Z , Pan J , Tang S , Duan D , Yu D , Nong H , Wang Z ((2021) ) Global trends in the incidence, prevalence, and years lived with disability of Parkinson’s disease in 204 countries/territories from 1990 to 2019. Front Public Health 9: , 776847. |
[3] | Dorsey ER , Sherer T , Okun MS , Bloem BR ((2018) ) The emerging evidence of the Parkinson pandemic. J Parkinsons Dis 8: , S3–S8. |
[4] | Iwanaga K , Wakabayashi K , Yoshimoto M , Tomita I , Satoh H , Takashima H , Satoh A , Seto M , Tsujihata M , Takahashi H ((1999) ) Lewy body-type degeneration in cardiac plexus in Parkinson’s and incidental Lewy body diseases. Neurology 52: , 1269–1271. |
[5] | Wakabayashi K , Takahashi H , Takeda S , Ohama E , Ikuta F ((1988) ) Parkinson’s disease: The presence of Lewy bodies in Auerbach’s and Meissner’s plexuses. Acta Neuropathol 76: , 217–221. |
[6] | Yang R , Gao G , Yang H ((2022) ) The pathological mechanism between the intestine and brain in the early stage of Parkinson’s disease. Front Aging Neurosci 14: , 861035. |
[7] | Rodríguez-Leyva I , Calderón-Garcidueñas AL , Jiménez-Capdeville ME , Rentería-Palomo AA , Hernandez-Rodriguez HG , Valdés-Rodríguez R , Fuentes-Ahumada C , Torres-Álvarez B , Sepúlveda-Saavedra J , Soto-Domínguez A , Santoyo ME , Rodriguez-Moreno JI , Castanedo-Cázares JP ((2014) ) α-Synuclein inclusions in the skin of Parkinson’s disease and parkinsonism. Ann Clin Transl Neurol 1: , 471–478. |
[8] | Ikemura M , Saito Y , Sengoku R , Sakiyama Y , Hatsuta H , Kanemaru K , Sawabe M , Arai T , Ito G , Iwatsubo T , Fukayama M , Murayama S ((2008) ) Lewy body pathology involves cutaneous nerves. J Neuropathol Exp Neurol 67: , 945–953. |
[9] | Kalia LV , Lang AE ((2015) ) Parkinson’s disease. Lancet 386: , 896–912. |
[10] | Dickson DW ((2012) ) Parkinson’s disease and parkinsonism: Neuropathology. Cold Spring Harb Perspect Med 2: , a009258. |
[11] | Dauer W , Przedborski S ((2003) ) Parkinson’s disease: Mechanisms and models. Neuron 39: , 889–909. |
[12] | O’Keeffe FE , Scott SA , Tyers P , O’Keeffe GW , Dalley JW , Zufferey R , Caldwell MA ((2008) ) Induction of A9 dopaminergic neurons from neural stem cells improves motor function in an animal model of Parkinson’s disease. Brain 131: , 630–641. |
[13] | Piao J , Zabierowski S , Dubose BN , Hill EJ , Navare M , Claros N , Rosen S , Ramnarine K , Horn C , Fredrickson C , Wong K , Safford B , Kriks S , El Maarouf A , Rutishauser U , Henchcliffe C , Wang Y , Riviere I , Mann S , Bermudez V , Irion S , Studer L , Tomishima M , Tabar V ((2021) ) Preclinical efficacy and safety of a human embryonic stem cell-derived midbrain dopamine progenitor product, MSK-DA01. Cell Stem Cell 28: , 217–229.e7. |
[14] | Doi D, Magotani H, Kikuchi T, Ikeda M, Hiramatsu S, Yoshida K, Amano N, Nomura M, Umekage M, Morizane A, Takahashi J ((2020) ) Pre-clinical study of induced pluripotent stem cell-derived dopaminergic progenitor cells for Parkinson’s disease. Nat Commun 11: , 3369. |
[15] | Jankovic J , Aguilar LG ((2008) ) Current approaches to the treatment of Parkinson’s disease. Neuropsychiatr Dis Treat 4: , 743–757. |
[16] | Björklund A , Stenevi U ((1979) ) Reconstruction of the nigrostriatal dopamine pathway by intracerebral nigral transplants. Brain Res 177: , 555–560. |
[17] | Björklund A , Dunnett SB , Stenevi U , Lewis ME , Iversen SD ((1980) ) Reinnervation of the denervated striatum by substantia nigra transplants: Functional consequences as revealed by pharmacological and sensorimotor testing. Brain Res 199: , 307–333. |
[18] | Björklund A , Stenevi U , Dunnett SB , Iversen SD ((1981) ) Functional reactivation of the deafferented neostriatum by nigral transplants. Nature 289: , 497–499. |
[19] | Brundin P , Nilsson OG , Gage FH , Björklund A ((1985) ) Cyclosporin A increases survival of cross-species intrastriatal grafts of embryonic dopamine-containing neurons. Exp Brain Res 60: , 204–208. |
[20] | Parmar M , Torper O , Drouin-Ouellet J ((2019) ) Cell-based therapy for Parkinson’s disease: A journey through decades toward the light side of the Force. Eur J Neurosci 49: , 463–471. |
[21] | Barker RA , Drouin-Ouellet J , Parmar M ((2015) ) Cell-based therapies for Parkinson disease—past insights and future potential. Nat Rev Neurol 11: , 492–503. |
[22] | Freed CR , Greene PE , Breeze RE , Tsai WY , DuMouchel W , Kao R , Dillon S , Winfield H , Culver S , Trojanowski JQ , Eidelberg D , Fahn S ((2001) ) Transplantation of embryonic dopamine neurons for severe Parkinson’s disease. N Engl J Med 344: , 710–719. |
[23] | Olanow CW , Goetz CG , Kordower JH , Stoessl AJ , Sossi V , Brin MF , Shannon KM , Nauert GM , Perl DP , Godbold J , Freeman TB ((2003) ) A double-blind controlled trial of bilateral fetal nigral transplantation in Parkinson’s disease. Ann Neurol 54: , 403–414. |
[24] | Ma Y , Tang C , Chaly T , Greene P , Breeze R , Fahn S , Freed C , Dhawan V , Eidelberg D ((2010) ) Dopamine cell implantation in Parkinson’s disease: Long-term clinical and (18)F-FDOPA PET outcomes. J Nucl Med 51: , 7–15. |
[25] | Politis M , Oertel WH , Wu K , Quinn NP , Pogarell O , Brooks DJ , Bjorklund A , Lindvall O , Piccini P ((2011) ) Graft-induced dyskinesias in Parkinson’s disease: High striatal serotonin/dopamine transporter ratio. Mov Disord 26: , 1997–2003. |
[26] | Kefalopoulou Z , Politis M , Piccini P , Mencacci N , Bhatia K , Jahanshahi M , Widner H , Rehncrona S , Brundin P , Björklund A , Lindvall O , Limousin P , Quinn N , Foltynie T ((2014) ) Long-term clinical outcome of fetal cell transplantation for Parkinson disease: Two case reports. JAMA Neurol 71: , 83–87. |
[27] | Piccini P , Brooks DJ , Björklund A , Gunn RN , Grasby PM , Rimoldi O , Brundin P , Hagell P , Rehncrona S , Widner H , Lindvall O ((1999) ) Dopamine release from nigral transplants visualized in vivo in a Parkinson’s patient. Nat Neurosci 2: , 1137–1140. |
[28] | Li J-Y , Li W ((2021) ) Postmortem studies of fetal grafts in Parkinson’s disease: What lessons have we learned? Front Cell Dev Biol 9: , 666675. |
[29] | Barker RA , Barrett J , Mason SL , Björklund A ((2013) ) Fetal dopaminergic transplantation trials and the future of neural grafting in Parkinson’s disease. Lancet Neurol 12: , 84–91. |
[30] | Potts MB , Silvestrini MT , Lim DA ((2013) ) Devices for cell transplantation into the central nervous system: Design considerations and emerging technologies. Surg Neurol Int 4: , S22–S30. |
[31] | Amer MH , Rose FRAJ , Shakesheff KM , Modo M , White LJ ((2017) ) Translational considerations in injectable cell-based therapeutics for neurological applications: Concepts, progress and challenges. NPJ Regen Med 2: , 23. |
[32] | Kondziolka D , Gobbel GT , Fellows-Mayle W , Chang Y-F , Uram M ((2011) ) Injection parameters affect cell viability and implant volumes in automated cell delivery for the brain. Cell Transplant 20: , 1901–1906. |
[33] | Gobbel GT , Kondziolka D , Fellows-Mayle W , Uram M ((2010) ) Manual vs automated delivery of cells for transplantation: Accuracy, reproducibility, and impact on viability. Neurosurgery 67: , 1662–1668; discussion 1668. |
[34] | Barker RA , Parmar M , Studer L , Takahashi J ((2017) ) Human Trials of Stem Cell-Derived Dopamine Neurons for Parkinson’s Disease: Dawn of a New Era. Cell Stem Cell 21: , 569–573. |
[35] | Kirkeby A , Nolbrant S , Tiklova K , Heuer A , Kee N , Cardoso T , Ottosson DR , Lelos MJ , Rifes P , Dunnett SB , Grealish S , Perlmann T , Parmar M ((2017) ) Predictive markers guide differentiation to improve graft outcome in clinical translation of hESC-based therapy for Parkinson’s disease. Cell Stem Cell 20: , 135–148. |
[36] | Barker RA , Studer L , Cattaneo E , Takahashi J , G-Force PD consortium ((2015) ) G-Force PD: A global initiative in coordinating stem cell-based dopamine treatments for Parkinson’s disease. NPJ Parkinsons Dis 1: , 15017. |
[37] | Kirkeby A , Nelander J , Hoban DB , Rogelius N , Bjartmarz H , Novo Nordisk Cell Therapy R&D, Storm P , Fiorenzano A , Adler AF , Vale S , Mudannayake J , Zhang Y , Cardoso T , Mattsson B , Landau AM , Glud AN , Sørensen JC , Lillethorup TP , Lowdell M , Carvalho C , Bain O , van Vliet T , Lindvall O , Björklund A , Harry B , Cutting E , Widner H , Paul G , Barker RA , Parmar M ((2023) ) Preclinical quality, safety, and efficacy of a human embryonic stem cell-derived product for the treatment of Parkinson’s disease, STEM-PD. Cell Stem Cell 30: , 1299–1314.e9. |
[38] | Li Y , Wu H , Jiang X , Dong Y , Zheng J , Gao J ((2022) ) New idea to promote the clinical applications of stem cells or their extracellular vesicles in central nervous system disorders: Combining with intranasal delivery. Acta Pharm Sin B 12: , 3215–3232. |
[39] | Burgess A , Ayala-Grosso CA , Ganguly M , Jordão JF , Aubert I , Hynynen K ((2011) ) Targeted delivery of neural stem cells to the brain using MRI-guided focused ultrasound to disrupt the blood-brain barrier. PLoS One 6: , e27877. |
[40] | Backlund EO , Granberg PO , Hamberger B , Knutsson E , Mårtensson A , Sedvall G , Seiger A , Olson L ((1985) ) Transplantation of adrenal medullary tissue to striatum in parkinsonism. First clinical trials. J Neurosurg 62: , 169–173. |
[41] | Madrazo I , Drucker-Colín R , Díaz V , Martínez-Mata J , Torres C , Becerril JJ ((1987) ) Open microsurgical autograft of adrenal medulla to the right caudate nucleus in two patients with intractable Parkinson’s disease. N Engl J Med 316: , 831–834. |
[42] | Lindvall O , Backlund EO , Farde L , Sedvall G , Freedman R , Hoffer B , Nobin A , Seiger A , Olson L ((1987) ) Transplantation in Parkinson’s disease: Two cases of adrenal medullary grafts to the putamen. Ann Neurol 22: , 457–468. |
[43] | Allen GS , Burns RS , Tulipan NB , Parker RA ((1989) ) Adrenal medullary transplantation to the caudate nucleus in Parkinson’s disease. Initial clinical results in 18 patients. Arch Neurol 46: , 487–491. |
[44] | Drucker-Colín R , Madrazo I , Ostrosky-Solís F , Shkurovich M , Franco R , Torres C ((1988) ) Adrenal medullary tissue transplants in the caudate nucleus of Parkinson’s patients. Prog Brain Res 78: , 567–574. |
[45] | Goetz CG , Olanow CW , Koller WC , Penn RD , Cahill D , Morantz R , Stebbins G , Tanner CM , Klawans HL , Shannon KM ((1989) ) Multicenter study of autologous adrenal medullary transplantation to the corpus striatum in patients with advanced Parkinson’s disease. N Engl J Med 320: , 337–341. |
[46] | Goetz CG , Tanner CM , Penn RD , Stebbins GT , Gilley DW , Shannon KM , Klawans HL , Comella CL , Wilson RS , Witt T ((1990) ) Adrenal medullary transplant to the striatum of patients with advanced Parkinson’s disease: 1-year motor and psychomotor data. Neurology 40: , 273–276. |
[47] | Jankovic J , Grossman R , Goodman C , Pirozzolo F , Schneider L , Zhu Z , Scardino P , Garber AJ , Jhingran SG , Martin S ((1989) ) Clinical, biochemical, and neuropathologic findings following transplantation of adrenal medulla to the caudate nucleus for treatment of Parkinson’s disease. Neurology 39: , 1227–1234. |
[48] | Jiao SS , Zhang WC , Cao JK , Zhang ZM , Wang H , Ding MC , Zhang Z , Sun JB , Sun YC , Shi MT ((1988) ) Study of adrenal medullary tissue transplantation to striatum in parkinsonism. Prog Brain Res 78: , 575–580. |
[49] | ((1989) ) Adrenal medullary autografts in patients with Parkinson’s disease. N Engl J Med 321: , 324–327. |
[50] | Kelly PJ , Ahlskog JE , van Heerden JA , Carmichael SW , Stoddard SL , Bell GN ((1989) ) Adrenal medullary autograft transplantation into the striatum of patients with Parkinson’s disease. Mayo Clin Proc 64: , 282–290. |
[51] | Ostrosky-Solís F , Quintanar L , Madrazo I , Drucker-Colín R , Franco-Bourland R , Leon-Meza V ((1988) ) Neuropsychological effects of brain autograft of adrenal medullary tissue for the treatment of Parkinson’s disease. Neurology 38: , 1442–1450. |
[52] | Phillips N ((2018) ) Cranial Neurosurgery: GIRFT Programme National Specialty Report, https://gettingitrightfirsttime.co.uk/wp-content/uploads/2018/07/CranialNeurosurgeryJune18-L.pdf |
[53] | Slupe AM , Kirsch JR ((2018) ) Effects of anesthesia on cerebral blood flow, metabolism, and neuroprotection. J Cereb Blood Flow Metab 38: , 2192–2208. |
[54] | Dinsmore J ((2007) ) Anaesthesia for elective neurosurgery. Br J Anaesth 99: , 68–74. |
[55] | Flexman AM , Meng L , Gelb AW ((2016) ) Outcomes in neuroanesthesia: What matters most? Can J Anaesth 63: , 205–211. |
[56] | Barker FG ((1994) ) Efficacy of prophylactic antibiotics for craniotomy: A meta-analysis. Neurosurgery 35: , 484–490; discussion 491. |
[57] | Cao Y , Pu K , Li G , Yan X , Ma Y , Xue K , Sun Z , Li Q ((2017) ) The role of antibiotic prophylaxis in clean neurosurgery. World Neurosurg 100: , 305–310. |
[58] | Maragkos GA , McNeill IT , Kessler R , Xie M , Schaefer S , Patel G , Bederson JB , Shrivastava RK ((2021) ) Letter: Comprehensive neurosurgery infection prevention and control practice in the COVID-19 “return to operate” era. J Sci Innov Med 4: , 33. |
[59] | Vermilyea SC , Lu J , Olsen M , Guthrie S , Tao Y , Fekete EM , Riedel MK , Brunner K , Boettcher C , Bondarenko V , Brodsky E , Block WF , Alexander A , Zhang S-C , Emborg ME ((2017) ) Real-time intraoperative MRI intracerebral delivery of induced pluripotent stem cell-derived neurons. Cell Transplant 26: , 613–624. |
[60] | Richardson RM , Bankiewicz KS , Christine CW , Van Laar AD , Gross RE , Lonser R , Factor SA , Kostyk SK , Kells AP , Ravina B , Larson PS ((2020) ) Data-driven evolution of neurosurgical gene therapy delivery in Parkinson’s disease. J Neurol Neurosurg Psychiatr 91: , 1210–1218. |
[61] | Mendez I , Dagher A , Hong M , Gaudet P , Weerasinghe S , McAlister V , King D , Desrosiers J , Darvesh S , Acorn T , Robertson H ((2002) ) Simultaneous intrastriatal and intranigral fetal dopaminergic grafts in patients with Parkinson disease: A pilot study. Report of three cases. J Neurosurg 96: , 589–596. |
[62] | Xiong M , Tao Y , Gao Q , Feng B , Yan W , Zhou Y , Kotsonis TA , Yuan T , You Z , Wu Z , Xi J , Haberman A , Graham J , Block J , Zhou W , Chen Y , Zhang S-C ((2021) ) Human stem cell-derived neurons repair circuits and restore neural function. Cell Stem Cell 28: , 112–126.e6. |
[63] | Rath A , Klein A , Papazoglou A , Pruszak J , Garcia J , Krause M , Maciaczyk J , Dunnett SB , Nikkhah G ((2013) ) Survival and functional restoration of human fetal ventral mesencephalon following transplantation in a rat model of Parkinson’s disease. Cell Transplant 22: , 1281–1293. |
[64] | Kurowska Z , Englund E , Widner H , Lindvall O , Li J-Y , Brundin P ((2011) ) Signs of degeneration in 12-22-year old grafts of mesencephalic dopamine neurons in patients with Parkinson’s disease. J Parkinsons Dis 1: , 83–92. |
[65] | Li W , Englund E , Widner H , Mattsson B , van Westen D , Lätt J , Rehncrona S , Brundin P , Björklund A , Lindvall O , Li J-Y ((2016) ) Extensive graft-derived dopaminergic innervation is maintained 24 years after transplantation in the degenerating parkinsonian brain. Proc Natl Acad Sci U S A 113: , 6544–6549. |
[66] | Castilho RF , Hansson O , Brundin P ((2000) ) Improving the survival of grafted embryonic dopamine neurons in rodent models of Parkinson’s disease. Prog Brain Res 127: , 203–231. |
[67] | Niclis JC , Gantner CW , Hunt CPJ , Kauhausen JA , Durnall JC , Haynes JM , Pouton CW , Parish CL , Thompson LH ((2017) ) A PITX3-EGFP reporter line reveals connectivity of dopamine and non-dopamine neuronal subtypes in grafts generated from human embryonic stem cells. Stem Cell Reports 9: , 868–882. |
[68] | Kordower JH , Goetz CG , Chu Y , Halliday GM , Nicholson DA , Musial TF , Marmion DJ , Stoessl AJ , Sossi V , Freeman TB , Olanow CW ((2017) ) Robust graft survival and normalized dopaminergic innervation do not obligate recovery in a Parkinson disease patient. Ann Neurol 81: , 46–57. |
[69] | Kikuchi T, Morizane A, Doi D, Magotani H, Onoe H, Hayashi T, Mizuma H, Takara S, Takahashi R, Inoue H, Morita S, Yamamoto M, Okita K, Nakagawa M, Parmar M, Takahashi J ((2017) ) Human iPS cell-derived dopaminergic neurons function in a primate Parkinson’s disease model. Nature 548: , 592–596. |
[70] | Barker RA , TRANSEURO consortium ((2019) ) Designing stem-cell-based dopamine cell replacement trials for Parkinson’s disease. Nat Med 25: , 1045–1053. |
[71] | Skuk D , Paradis M , Goulet M , Tremblay JP ((2007) ) Ischemic central necrosis in pockets of transplanted myoblasts in nonhuman primates: Implications for cell-transplantation strategies. Transplantation 84: , 1307–1315. |
[72] | Varenika V , Kells AP , Valles F , Hadaczek P , Forsayeth J , Bankiewicz KS ((2009) ) Controlled dissemination of AAV vectors in the primate brain. Prog Brain Res 175: , 163–172. |
[73] | Yin D , Forsayeth J , Bankiewicz KS ((2010) ) Optimized cannula design and placement for convection-enhanced delivery in rat striatum. J Neurosci Methods 187: , 46–51. |
[74] | Walker PA , Jimenez F , Gerber MH , Aroom KR , Shah SK , Harting MT , Gill BS , Savitz SI , Cox CS ((2010) ) Effect of needle diameter and flow rate on rat and human mesenchymal stromal cell characterization and viability. Tissue Eng Part C Methods 16: , 989–997. |
[75] | Perry VH , Andersson PB , Gordon S ((1993) ) Macrophages and inflammation in the central nervous system. Trends Neurosci 16: , 268–273. |
[76] | Borlongan CV , Weiss MD ((2011) ) Baby STEPS: A giant leap for cell therapy in neonatal brain injury. Pediatr Res 70: , 3–9. |
[77] | Sunness JS ((2015) ) Stem cells in age-related macular degeneration and Stargardt’s macular dystrophy. Lancet 386: , 29. |
[78] | Lindvall O , Rehncrona S , Brundin P , Gustavii B , Astedt B , Widner H , Lindholm T , Björklund A , Leenders KL , Rothwell JC , Frackowiak R , Marsden D , Johnels B , Steg G , Freedman R , Hoffer BJ , Seiger A , Bygdeman M , Strömberg I , Olson L ((1989) ) Human fetal dopamine neurons grafted into the striatum in two patients with severe Parkinson’s disease. A detailed account of methodology and a 6-month follow-up. Arch Neurol 46: , 615–631. |
[79] | Stenevi U , Björklund A , Svendgaard NA ((1976) ) Transplantation of central and peripheral monoamine neurons to the adult rat brain: Techniques and conditions for survival. Brain Res 114: , 1–20. |
[80] | Stenevi U , Björklund A , Dunnett SB ((1980) ) Functional reinnervation of the denervated neostriatum by nigral transplants. Peptides 1: , 111–116. |
[81] | Sinclair SR , Fawcett JW , Dunnett SB ((1999) ) Delayed implantation of nigral grafts improves survival of dopamine neurones and rate of functional recovery. Neuroreport 10: , 1263–1267. |
[82] | Lindvall O , Widner H , Rehncrona S , Brundin P , Odin P , Gustavii B , Frackowiak R , Leenders KL , Sawle G , Rothwell JC ((1992) ) Transplantation of fetal dopamine neurons in Parkinson’s disease: One-year clinical and neurophysiological observations in two patients with putaminal implants. Ann Neurol 31: , 155–165. |
[83] | Lindvall O , Sawle G , Widner H , Rothwell JC , Björklund A , Brooks D , Brundin P , Frackowiak R , Marsden CD , Odin P ((1994) ) Evidence for long-term survival and function of dopaminergic grafts in progressive Parkinson’s disease. Ann Neurol 35: , 172–180. |
[84] | Nikkhah G , Cunningham MG , Jödicke A , Knappe U , Björklund A ((1994) ) Improved graft survival and striatal reinnervation by microtransplantation of fetal nigral cell suspensions in the rat Parkinson model. Brain Res 633: , 133–143. |
[85] | Nikkhah G , Olsson M , Eberhard J , Bentlage C , Cunningham MG , Björklund A ((1994) ) A microtransplantation approach for cell suspension grafting in the rat Parkinson model: A detailed account of the methodology. Neuroscience 63: , 57–72. |
[86] | Brecknell JE , Fawcett JW ((1996) ) A device for the implantation of multiple cellular deposits into a large volume of brain from a single cannula site. Exp Neurol 138: , 338–344. |
[87] | Wiedenmann von CA ((2018) ) Grafting instrument comparison in a rodent model of Parkinson’ s disease. Thesis. |
[88] | Mendez I , Hong M , Smith S , Dagher A , Desrosiers J ((2000) ) Neural transplantation cannula and microinjector system: Experimental and clinical experience. Technical note. J Neurosurg 92: , 493–499. |
[89] | Kordower JH , Freeman TB , Snow BJ , Vingerhoets FJ , Mufson EJ , Sanberg PR , Hauser RA , Smith DA , Nauert GM , Perl DP ((1995) ) Neuropathological evidence of graft survival and striatal reinnervation after the transplantation of fetal mesencephalic tissue in a patient with Parkinson’s disease. N Engl J Med 332: , 1118–1124. |
[90] | Kordower JH , Rosenstein JM , Collier TJ , Burke MA , Chen EY , Li JM , Martel L , Levey AE , Mufson EJ , Freeman TB , Olanow CW ((1996) ) Functional fetal nigral grafts in a patient with Parkinson’s disease: Chemoanatomic, ultrastructural, and metabolic studies. J Comp Neurol 370: , 203–230. |
[91] | Hauser RA , Freeman TB , Snow BJ , Nauert M , Gauger L , Kordower JH , Olanow CW ((1999) ) Long-term evaluation of bilateral fetal nigral transplantation in Parkinson disease. Arch Neurol 56: , 179–187. |
[92] | Torres EM , Trigano M , Dunnett SB ((2015) ) Translation of cell therapies to the clinic: Characteristics of cell suspensions in large-diameter injection cannulae. Cell Transplant 24: , 737–749. |
[93] | Cunningham MG , Bolay H , Scouten CW , Moore C , Jacoby D , Moskowitz M , Sorensen JC ((2004) ) Preclinical evaluation of a novel intracerebral microinjection instrument permitting electrophysiologically guided delivery of therapeutics. Neurosurgery 54: , 1497–1507; discussion 1507. |
[94] | Bjarkam CR , Glud AN , Margolin L , Reinhart K , Franklin R , Deding D , Ettrup KS , Fitting LM , Nielsen MS , Sørensen J-CH , Cunningham MG ((2010) ) Safety and function of a new clinical intracerebral microinjection instrument for stem cells and therapeutics examined in the Göttingen minipig. Stereotact Funct Neurosurg 88: , 56–63. |
[95] | Barua NU , Gill SS , Love S ((2014) ) Convection-enhanced drug delivery to the brain: Therapeutic potential and neuropathological considerations. Brain Pathol 24: , 117–127. |
[96] | Lewis O , Woolley M , Johnson DE , Fletcher J , Fenech J , Pietrzyk MW , Barua NU , Bienemann AS , Singleton W , Evans SL , Gill SS ((2018) ) Maximising coverage of brain structures using controlled reflux, convection-enhanced delivery and the recessed step catheter. J Neurosci Methods 308: , 337–345. |
[97] | Whone A , Luz M , Boca M , Woolley M , Mooney L , Dharia S , Broadfoot J , Cronin D , Schroers C , Barua NU , Longpre L , Barclay CL , Boiko C , Johnson GA , Fibiger HC , Harrison R , Lewis O , Pritchard G , Howell M , Irving C , Johnson D , Kinch S , Marshall C , Lawrence AD , Blinder S , Sossi V , Stoessl AJ , Skinner P , Mohr E , Gill SS ((2019) ) Randomized trial of intermittent intraputamenal glial cell line-derived neurotrophic factor in Parkinson’s disease. Brain 142: , 512–525. |
[98] | Huttunen HJ , Booms S , Sjögren M , Kerstens V , Johansson J , Holmnäs R , Koskinen J , Kulesskaya N , Fazio P , Woolley M , Brady A , Williams J , Johnson D , Dailami N , Gray W , Levo R , Saarma M , Halldin C , Marjamaa J , Resendiz-Nieves J , Grubor I , Lind G , Eerola-Rautio J , Mertsalmi T , Andréasson M , Paul G , Rinne J , Kivisaari R , Bjartmarz H , Almqvist P , Varrone A , Scheperjans F , Widner H , Svenningsson P ((2023) ) Intraputamenal cerebral dopamine neurotrophic factor in Parkinson’s disease: A randomized, double-blind, multicenter phase 1 trial. Mov Disord 138: , 1209–1222. |
[99] | Rickmann A , Wahl S , Hofmann N , Knakowski J , Haus A , Börgel M , Szurman P ((2020) ) Comparison of preloaded grafts for Descemet membrane endothelial keratoplasty (DMEK) in a novel preloaded transport cartridge compared to conventional precut grafts. Cell Tissue Bank 21: , 205–213. |
[100] | Aguado BA , Mulyasasmita W , Su J , Lampe KJ , Heilshorn SC ((2012) ) Improving viability of stem cells during syringe needle flow through the design of hydrogel cell carriers. Tissue Eng Part A 18: , 806–815. |
[101] | Peterson SL ((1998) ) Drug microinjection in discrete brain regions. KOPF Carrier 50: , 1–6. |
[102] | Morrison PF , Chen MY , Chadwick RS , Lonser RR , Oldfield EH ((1999) ) Focal delivery during direct infusion to brain: Role of flow rate, catheter diameter, and tissue mechanics. Am J Physiol 277: , R1218–R1229. |
[103] | Amer MH , White LJ , Shakesheff KM ((2015) ) The effect of injection using narrow-bore needles on mammalian cells: Administration and formulation considerations for cell therapies. J Pharm Pharmacol 67: , 640–650. |
[104] | Amer MH , Rose FRAJ , White LJ , Shakesheff KM ((2016) ) A detailed assessment of varying ejection rate on delivery efficiency of mesenchymal stem cells using narrow-bore needles. Stem Cells Transl Med 5: , 366–378. |
[105] | Heaton JT , Kobler JB , Ottensmeyer MP , Lopez-Guerra G , Karajanagi SS , Burns JA , Zeitels SM ((2012) ) Modification and testing of a pneumatic dispensing device for controlled delivery of injectable materials. Laryngoscope 122: , 2023–2028. |
[106] | Chen MY , Lonser RR , Morrison PF , Governale LS , Oldfield EH ((1999) ) Variables affecting convection-enhanced delivery to the striatum: A systematic examination of rate of infusion, cannula size, infusate concentration, and tissue-cannula sealing time. J Neurosurg 90: , 315–320. |
[107] | Krauze MT , Saito R , Noble C , Tamas M , Bringas J , Park JW , Berger MS , Bankiewicz K ((2005) ) Reflux-free cannula for convection-enhanced high-speed delivery of therapeutic agents. J Neurosurg 103: , 923–929. |
[108] | Schweitzer JS , Song B , Leblanc PR , Feitosa M , Carter BS , Kim K-S ((2020) ) Columnar injection for intracerebral cell therapy. Oper Neurosurg (Hagerstown) 18: , 321–328. |
[109] | Hagell P , Piccini P , Björklund A , Brundin P , Rehncrona S , Widner H , Crabb L , Pavese N , Oertel WH , Quinn N , Brooks DJ , Lindvall O ((2002) ) Dyskinesias following neural transplantation in Parkinson’s disease. Nat Neurosci 5: , 627–628. |
[110] | Kunath T , Natalwala A , Chan C , Chen Y , Stecher B , Taylor M , Khan S , Muqit MMK ((2019) ) Are PARKIN patients ideal candidates for dopaminergic cell replacement therapies? Eur J Neurosci 49: , 453–462. |
[111] | The Michael J. Fox Foundation (2022) Phase 1b Trial to Assess the Safety and Efficacy of Dopamine Cell Transplants in Parkinson’s Disease Linked to Mutations in Parkin. Available at: https://www.michaeljfox.org/grant/phase-1b-trial-assess-safety-and-efficacy-dopamine-cell-transplants-parkinsons-disease-linked |
[112] | Collier TJ , Sortwell CE , Daley BF ((1999) ) Diminished viability, growth, and behavioral efficacy of fetal dopamine neuron grafts in aging rats with long-term dopamine depletion: An argument for neurotrophic supplementation. J Neurosci 19: , 5563–5573. |
[113] | Collier TJ , O’Malley J , Rademacher DJ , Stancati JA , Sisson KA , Sortwell CE , Paumier KL , Gebremedhin KG , Steece-Collier K ((2015) ) Interrogating the aged striatum: Robust survival of grafted dopamine neurons in aging rats produces inferior behavioral recovery and evidence of impaired integration. Neurobiol Dis 77: , 191–203. |
[114] | Grinand L , Takahashi J ((2022) ) Automated measurement of fluorescence signals reveals a significant increase of the graft-derived neurite extension in neonates compared to aged rats. Regen Ther 19: , 97–106. |
[115] | Rowland NC , Starr PA , Larson PS , Ostrem JL , Marks WJ , Lim DA ((2015) ) Combining cell transplants or gene therapy with deep brain stimulation for Parkinson’s disease. Mov Disord 30: , 190–195. |
[116] | Takahashi K , Yamanaka S ((2006) ) Induction of pluripotent stem cells from mouse embryonic and adult fibroblast cultures by defined factors. Cell 126: , 663–676. |
[117] | Li H , Jiang H , Li H , Li L , Yan Z , Feng J ((2022) ) Generation of human A9 dopaminergic pacemakers from induced pluripotent stem cells. Mol Psychiatry 27: , 4407–4418. |
[118] | Stoker TB ((2018) ) Stem cell treatments for Parkinson’s disease. In Parkinson’s Disease: Pathogenesis and Clinical Aspects, Stoker TB, Greenland JC, eds. Codon Publications, Brisbane (AU). |
[119] | Morizane A, Doi D, Kikuchi T, Okita K, Hotta A, Kawasaki T, Hayashi T, Onoe H, Shiina T, Yamanaka S, Takahashi J ((2013) ) Direct comparison of autologous and allogeneic transplantation of iPSC-derived neural cells in the brain of a non-human primate. Stem Cell Reports 1: , 283–292. |
[120] | Hallett PJ , Deleidi M , Astradsson A , Smith GA , Cooper O , Osborn TM , Sundberg M , Moore MA , Perez-Torres E , Brownell A-L , Schumacher JM , Spealman RD , Isacson O ((2015) ) Successful function of autologous iPSC-derived dopamine neurons following transplantation in a non-human primate model of Parkinson’s disease. Cell Stem Cell 16: , 269–274. |
[121] | Schweitzer JS , Song B , Herrington TM , Park T-Y , Lee N , Ko S , Jeon J , Cha Y , Kim K , Li Q , Henchcliffe C , Kaplitt M , Neff C , Rapalino O , Seo H , Lee I-H , Kim J , Kim T , Petsko GA , Ritz J , Cohen BM , Kong S-W , Leblanc P , Carter BS , Kim K-S ((2020) ) Personalized iPSC-derived dopamine progenitor cells for Parkinson’s disease. N Engl J Med 382: , 1926–1932. |
[122] | Parmar M , Björklund A ((2020) ) From skin to brain: A Parkinson’s disease patient transplanted with his own cells. Cell Stem Cell 27: , 8–10. |
[123] | Fan Y , Winanto , Ng S-Y ((2020) ) Replacing what’s lost: A new era of stem cell therapy for Parkinson’s disease. Transl Neurodegener 9: , 2. |
[124] | Morizane A, Kikuchi T, Hayashi T, Mizuma H, Takara S, Doi H, Mawatari A, Glasser MF, Shiina T, Ishigaki H, Itoh Y, Okita K, Yamasaki E, Doi D, Onoe H, Ogasawara K, Yamanaka S, Takahashi J ((2017) ) MHC matching improves engraftment of iPSC-derived neurons in non-human primates. Nat Commun 8: , 385. |
[125] | Mandai M , Watanabe A , Kurimoto Y , Hirami Y , Morinaga C , Daimon T , Fujihara M , Akimaru H , Sakai N , Shibata Y , Terada M , Nomiya Y , Tanishima S , Nakamura M , Kamao H , Sugita S , Onishi A , Ito T , Fujita K , Kawamata S , Go MJ , Shinohara C , Hata K-I , Sawada M , Yamamoto M , Ohta S , Ohara Y , Yoshida K , Kuwahara J , Kitano Y , Amano N , Umekage M , Kitaoka F , Tanaka A , Okada C , Takasu N , Ogawa S , Yamanaka S , Takahashi M ((2017) ) autologous induced stem-cell-derived retinal cells for macular degeneration. N Engl J Med 376: , 1038–1046. |
[126] | Garber K ((2015) ) RIKEN suspends first clinical trial involving induced pluripotent stem cells. Nat Biotechnol 33: , 890–891. |
[127] | Nakatsuji N , Nakajima F , Tokunaga K ((2008) ) HLA-haplotype banking and iPS cells. Nat Biotechnol 26: , 739–740. |
[128] | Wilmut I , Leslie S , Martin NG , Peschanski M , Rao M , Trounson A , Turner D , Turner ML , Yamanaka S , Taylor CJ ((2015) ) Development of a global network of induced pluripotent stem cell haplobanks. Regen Med 10: , 235–238. |
[129] | Saito MK , Matsunaga A , Takasu N , Yamanaka S ((2014) ) Donor recruitment and eligibility criteria for HLA-homozygous iPS cell bank in Japan. In Stem Cell Banking, Ilic D, ed. Springer New York, New York, pp. 67–76. |
[130] | Takahashi J ((2017) ) Strategies for bringing stem cell-derived dopamine neurons to the clinic: The Kyoto trial. Prog Brain Res 230: , 213–226. |
[131] | Takahashi J ((2020) ) iPS cell-based therapy for Parkinson’s disease: A Kyoto trial. Regen Ther 13: , 18–22. |
[132] | Deuse T , Hu X , Gravina A , Wang D , Tediashvili G , De C , Thayer WO , Wahl A , Garcia JV , Reichenspurner H , Davis MM , Lanier LL , Schrepfer S ((2019) ) Hypoimmunogenic derivatives of induced pluripotent stem cells evade immune rejection in fully immunocompetent allogeneic recipients. Nat Biotechnol 37: , 252–258. |
[133] | Chao MP , Weissman IL , Majeti R ((2012) ) The CD47-SIRPα pathway in cancer immune evasion and potential therapeutic implications. Curr Opin Immunol 24: , 225–232. |
[134] | Greco R , Oliveira G , Stanghellini MTL , Vago L , Bondanza A , Peccatori J , Cieri N , Marktel S , Mastaglio S , Bordignon C , Bonini C , Ciceri F ((2015) ) Improving the safety of cell therapy with the TK-suicide gene. Front Pharmacol 6: , 95. |
[135] | He J , Rong Z , Fu X , Xu Y ((2017) ) A safety checkpoint to eliminate cancer risk of the immune evasive cells derived from human embryonic stem cells. Stem Cells 35: , 1154–1161. |
[136] | Chen Y , Dolt KS , Kriek M , Baker T , Downey P , Drummond NJ , Canham MA , Natalwala A , Rosser S , Kunath T ((2019) ) Engineering synucleinopathy-resistant human dopaminergic neurons by CRISPR-mediated deletion of the SNCA gene. Eur J Neurosci 49: , 510–524. |
[137] | Adachi H , Morizane A , Torikoshi S , Raudzus F , Taniguchi Y , Miyamoto S , Sekiguchi K , Takahashi J ((2022) ) Pretreatment with perlecan-conjugated laminin-E8 fragment enhances maturation of grafted dopaminergic progenitors in Parkinson’s disease model. Stem Cells Transl Med 11: , 767–777. |
[138] | Moriarty N , Parish CL , Dowd E ((2019) ) Primary tissue for cellular brain repair in Parkinson’s disease: Promise, problems and the potential of biomaterials. Eur J Neurosci 49: , 472–486. |
[139] | Moriarty N , Cabré S , Alamilla V , Pandit A , Dowd E ((2019) ) Encapsulation of young donor age dopaminergic grafts in a GDNF-loaded collagen hydrogel further increases their survival, reinnervation, and functional efficacy after intrastriatal transplantation in hemi-Parkinsonian rats. Eur J Neurosci 49: , 487–496. |
[140] | Moriarty N , Pandit A , Dowd E ((2017) ) Encapsulation of primary dopaminergic neurons in a GDNF-loaded collagen hydrogel increases their survival, re-innervation and function after intra-striatal transplantation. Sci Rep 7: , 16033. |
[141] | Bruggeman KF , Moriarty N , Dowd E , Nisbet DR , Parish CL ((2019) ) Harnessing stem cells and biomaterials to promote neural repair. Br J Pharmacol 176: , 355–368. |
[142] | Ahn Y-H , Bensadoun J-C , Aebischer P , Zurn AD , Seiger A , Björklund A , Lindvall O , Wahlberg L , Brundin P , Kaminski Schierle GS ((2005) ) Increased fiber outgrowth from xeno-transplanted human embryonic dopaminergic neurons with co-implants of polymer-encapsulated genetically modified cells releasing glial cell line-derived neurotrophic factor. Brain Res Bull 66: , 135–142. |
[143] | Brundin P , Karlsson J , Emgård M , Schierle GS , Hansson O , Petersén A , Castilho RF ((2000) ) Improving the survival of grafted dopaminergic neurons: A review over current approaches. Cell Transplant 9: , 179–195. |
[144] | Hiller BM , Marmion DJ , Thompson CA , Elliott NA , Federoff H , Brundin P , Mattis VB , McMahon CW , Kordower JH ((2022) ) Optimizing maturity and dose of iPSC-derived dopamine progenitor cell therapy for Parkinson’s disease. NPJ Regen Med 7: , 24. |
[145] | Torikoshi S , Morizane A , Shimogawa T , Samata B , Miyamoto S , Takahashi J ((2020) ) Exercise promotes neurite extensions from grafted dopaminergic neurons in the direction of the dorsolateral striatum in Parkinson’s disease model rats. J Parkinsons Dis 10: , 511–521. |
[146] | Steinbeck JA , Choi SJ , Mrejeru A , Ganat Y , Deisseroth K , Sulzer D , Mosharov EV , Studer L ((2015) ) Optogenetics enables functional analysis of human embryonic stem cell-derived grafts in a Parkinson’s disease model. Nat Biotechnol 33: , 204–209. |
[147] | Chen Y , Xiong M , Dong Y , Haberman A , Cao J , Liu H , Zhou W , Zhang S-C ((2016) ) Chemical control of grafted human PSC-derived neurons in a mouse model of Parkinson’s disease. Cell Stem Cell 18: , 817–826. |
[148] | Björklund A , Dunnett SB , Brundin P , Stoessl AJ , Freed CR , Breeze RE , Levivier M , Peschanski M , Studer L , Barker R ((2003) ) Neural transplantation for the treatment of Parkinson’s disease. Lancet Neurol 2: , 437–445. |
[149] | Kim TW , Koo SY , Studer L ((2020) ) Pluripotent stem cell therapies for Parkinson disease: Present challenges and future opportunities. Front Cell Dev Biol 8: , 729. |
[150] | Defer GL , Widner H , Marié RM , Rémy P , Levivier M ((1999) ) Core assessment program for surgical interventional therapies in Parkinson’s disease (CAPSIT-PD). Mov Disord 14: , 572–584. |
[151] | Barker RA , Björklund A , Gash DM , Whone A , Van Laar A , Kordower JH , Bankiewicz K , Kieburtz K , Saarma M , Booms S , Huttunen HJ , Kells AP , Fiandaca MS , Stoessl AJ , Eidelberg D , Federoff H , Voutilainen MH , Dexter DT , Eberling J , Brundin P , Isaacs L , Mursaleen L , Bresolin E , Carroll C , Coles A , Fiske B , Matthews H , Lungu C , Wyse RK , Stott S , Lang AE ((2020) ) GDNF and Parkinson’s disease: Where next? A summary from a recent workshop. J Parkinsons Dis 10: , 875–891. |
[152] | Axelsen TM , Woldbye DPD ((2018) ) Gene therapy for Parkinson’s disease, an update. J Parkinsons Dis 8: , 195–215. |
[153] | Christine CW , Bankiewicz KS , Van Laar AD , Richardson RM , Ravina B , Kells AP , Boot B , Martin AJ , Nutt J , Thompson ME , Larson PS ((2019) ) Magnetic resonance imaging-guided phase 1 trial of putaminal AADC gene therapy for Parkinson’s disease. Ann Neurol 85: , 704–714. |
[154] | Moriarty N , Gantner CW , Hunt CPJ , Ermine CM , Frausin S , Viventi S , Ovchinnikov DA , Kirik D , Parish CL , Thompson LH ((2022) ) A combined cell and gene therapy approach for homotopic reconstruction of midbrain dopamine pathways using human pluripotent stem cells. Cell Stem Cell 29: , 434–448.e5. |
[155] | Xiao B , Zhang S-C , Tan E-K ((2022) ) Combination therapy using GDNF and cell transplant in Parkinson’s disease. Mol Neurodegener 17: , 49. |
[156] | Rowland NC , Kalia SK , Kalia LV , Larson PS , Lim DA , Bankiewicz KS ((2016) ) Merging DBS with viral vector or stem cell implantation: “hybrid” stereotactic surgery as an evolution in the surgical treatment of Parkinson’s disease. Mol Ther Methods Clin Dev 3: , 15051. |
[157] | Lindvall O , Brundin P , Widner H , Rehncrona S , Gustavii B , Frackowiak R , Leenders KL , Sawle G , Rothwell JC , Marsden CD ((1990) ) Grafts of fetal dopamine neurons survive and improve motor function in Parkinson’s disease. Science 247: , 574–577. |
[158] | Widner H , Tetrud J , Rehncrona S , Snow B , Brundin P , Gustavii B , Björklund A , Lindvall O , Langston JW ((1992) ) Bilateral fetal mesencephalic grafting in two patients with parkinsonism induced by 1-methyl-4-phenyl-1,2,3,6-tetrahydropyridine (MPTP). N Engl J Med 327: , 1556–1563. |
[159] | Wenning GK , Odin P , Morrish P , Rehncrona S , Widner H , Brundin P , Rothwell JC , Brown R , Gustavii B , Hagell P , Jahanshahi M , Sawle G , Björklund A , Brooks DJ , Marsden CD , Quinn NP , Lindvall O ((1997) ) Short- and long-term survival and function of unilateral intrastriatal dopaminergic grafts in Parkinson’s disease. Ann Neurol 42: , 95–107. |
[160] | Piccini P , Lindvall O , Björklund A , Brundin P , Hagell P , Ceravolo R , Oertel W , Quinn N , Samuel M , Rehncrona S , Widner H , Brooks DJ ((2000) ) Delayed recovery ofmovement-related cortical function in Parkinson’s disease after striatal dopaminergic grafts. Ann Neurol 48: , 689–695. |
[161] | Freed CR , Breeze RE , Rosenberg NL , Schneck SA , Wells TH , Barrett JN , Grafton ST , Huang SC , Eidelberg D , Rottenberg DA ((1990) ) Transplantation of human fetal dopamine cells for Parkinson’s disease. Results at 1 year. Arch Neurol 47: , 505–512. |
[162] | Peschanski M , Defer G , N’Guyen JP , Ricolfi F , Monfort JC , Remy P , Geny C , Samson Y , Hantraye P , Jeny R ((1994) ) Bilateral motor improvement and alteration of L-dopa effect in two patients with Parkinson’s disease following intrastriatal transplantation of foetal ventral mesencephalon. Brain 117: (Pt 3), 487–499. |
[163] | Freeman TB , Olanow CW , Hauser RA , Nauert GM , Smith DA , Borlongan CV , Sanberg PR , Holt DA , Kordower JH , Vingerhoets FJ ((1995) ) Bilateral fetal nigral transplantation into the postcommissural putamen in Parkinson’s disease. Ann Neurol 38: , 379–388. |
[164] | Silvestrini MT , Yin D , Coppes VG , Mann P , Martin AJ , Larson PS , Starr PA , Gupta N , Panter SS , Desai TA , Lim DA ((2013) ) Radially branched deployment for more efficient cell transplantation at the scale of the human brain. Stereotact Funct Neurosurg 91: , 92–103. |