Establishing Equivalent Aerobic Exercise Parameters Between Early-Stage Parkinson’s Disease and Pink1 Knockout Rats
Abstract
Background:
Rodent Parkinson’s disease (PD) models are valuable to interrogate neurobiological mechanisms of exercise that mitigate motor impairment. Translating these mechanisms to human PD must account for physical capabilities of the patient.
Objective:
To establish cardiovascular parameters as a common metric for cross-species translation of aerobic exercise impact.
Method:
We evaluated aerobic exercise impact on heart rate (HR) in 21 early-stage PD subjects (Hoehn Yahr ≤1.5) exercising in non-contact boxing training for ≥3 months, ≥3x/week. In 4-month-old Pink1 knockout (KO) rats exercising in a progressively-increased treadmill speed regimen, we determined a specific treadmill speed that increased HR to an extent similar in human subjects.
Results:
After completing aerobic exercise for ∼30 min, PD subjects had increased HR∼35% above baseline (∼63% maximum HR). Motor and cognitive test results indicated the exercising subjects completed the timed up and go (TUG) and trail-making test (TMT-A) in significantly less time versus exercise-naïve PD subjects. In KO and age-matched wild-type (WT) rats, treadmill speeds of 8–10 m/min increased HR up to 25% above baseline (∼67% maximum HR), with no further increases up to 16 m/min. Exercised KO, but not WT, rats showed increased locomotor activity compared to an age-matched exercise-naïve cohort at 5 months old.
Conclusion:
These proof-of-concept results indicate HR is a cross-species translation parameter to evaluate aerobic exercise impact on specific motor or cognitive functions in human subjects and rat PD models. Moreover, a moderate intensity exercise regimen is within the physical abilities of early-stage PD patients and is therefore applicable for interrogating neurobiological mechanisms in rat PD models.
INTRODUCTION
Aerobic exercise regimens can mitigate the progression of parkinsonian signs, particularly gait impairment and bradykinesia [1–6] through various aerobic activities such as bicycling [1, 2], treadmill [4, 5], dance [6, 7], or non-contact boxing training [8, 9]. The neurobiological mechanisms underlying the motoric and non-motoric benefits of exercise represent optimal and safe targets for pharmacological or genetic-based therapeutic approaches to mitigate Parkinson’s disease (PD) related impairments. However, the neurobiological and neurophysiological underpinnings of these lifestyle interventions in PD populations remain unclear. Thus, identifying these endogenous exercise-responsive neurobiological mechanisms in PD is an important research priority, particularly since exercise capacity in PD patients diminishes as disease severity increases [10].
Rodent PD models provide opportunities to fully interrogate the neural mechanisms of exercise that also occur in human PD. However, translation of exercise-related mechanisms from rodent PD models to human PD could be compromised if rodent exercise regimens are unrealistically strenuous and thus do not reflect physical limitations of the the PD patient into the experimental design. This issue is particularly more prevalent at advanced stages of PD [11] as patients often encounter obstacles to achieve therapeutically-effective exercise levels [12–14]. Exercise efficacy may also be affected by the timing of its initiation after PD diagnosis. Recent results from aging rats suggest that delaying exercise after parkinsonian signs are first present greatly diminishes its efficacy to alleviate parkinsonian signs [15]. The length of exercise practice is also a critical component of efficacy, as data suggest that PD patients need a minimum of 12-weeks of consistent exercise prior to realizing any physical benefits [16]. Taken together, the neurobiological mechanisms germane to motor recovery found in exercising rodent PD models will be most informative if the regimens are conducted within the limits of physical capabilities of PD patients.
To translate neurobiological mechanisms of exercise from rodent to human PD, the exercise paradigm, benefits and limitations of the rodent model, and timing of exercise requires external validity [17], which is compromised if rodent models do not emulate the limitations and clinical issues relevant to the patient. First, most PD patients in less severe stages can comply to exercise three times per week, ∼30 to 40 minutes per session [1, 2, 4, 5, 8]. While such regimens prove beneficial for mitigating motor impairment, the correlative intensity of exercise required for such benefits has not been fully elucidated in a translational paradigm. Regardless of the source or type of aerobic activity practiced by the PD patient, a unifying metric that reflects the exercise capabilities of the patient would improve the translation of exercise- responsive neurobiological mechanisms from rodent to human PD. Second, emulating the progressive and protracted nature of PD in humans can be challenging in the rodent PD model. Toxin models are well-established, but may have challenges from a translational perspective in terms of their rapid nature of dopamine or tyrosine hydroxylase loss in relation to the onset of motor impairment [18, 19]. These models leave a tight time window to implement and interpret exercise efficacy against nigrostriatal neuron loss and motor decline. Together, these fundamental knowledge gaps minimize translation of neurobiological mechanisms in rodent PD models to human PD.
We began to address these two major knowledge gaps by first determining if cardiovascular metrics associated with exercise intensity could be a common thread to align exercise impact between early-stage human PD and the Pink1 rat genetic PD model. To control variation in exercise modalities potentially confounding the effects of varying intensity on heart rate (HR) in early-stage PD patients, we first determined cardiovascular responses in early-stage PD patients already participating in an established aerobic exercise (non-contact) boxing training regimen [Punching Out Parkinson’s (POPS), University of Hard Knocks, Fort Worth, TX]. Next, we quantified the HR increase in response to incrementally increased treadmill speeds in 4-month-old Pink1 knockout (KO) and wild-type (WT) rats to determine the intensity needed to match the HR increase in the exercising patient.
Pink1 is a ubiquitously-expressed protein that plays a critical role in mitophagy [20, 21] and its loss of function by mutation is associated with young onset PD, as an autosomal recessive mutation [22–25]. Notably, Pink1 KO rats have a protracted rate of motor decline, beginning between 6 to 8 months old [26–32], and may therefore better emulate the progressive nature of nigrostriatal neuron loss and emergence of motor impairment seen in human PD, as compared to more rapid loss in toxin models [17–19, 33, 34]. Our lab has established that a treadmill speed of 10 m/min increases baseline HR ∼25% and alleviates parkinsonian signs in aging [35]. Therefore, our primary goal was to provide a baseline reference of aerobic exercise cardiovascular parameters to use in rodent PD models consistent within the physical capabilities of early-stage PD subjects. Accomplishing this task will increase predictive validity that the neurobiological mechanisms impacted by aerobic exercise in rat models are also occurring in human PD, regardless of the exercise modality employed. A secondary goal was to explore the neuroprotective effects of aerobic exercise in early-stage PD subjects using motor and cognitive outcomes to strengthen the translation of potential neurobiological mechanisms found in rat models of parkinsonism [35]. Thus, we explored the influence of a consistently practiced aerobic exercise regimen (∼30 minutes, 3x/wk, 3-mos) in early-stage PD subjects on quantifiable modalities of motor function and domains of cognitive function as a surrogate neural phenotype.
METHODS
Human subjects
Study design
First, to establish cross-species HR equivalencies in a within-subjects study, we determined pre- and post-exercise cardiovascular parameters in subjects with early-stage PD. Second, we conducted a cross-sectional matched-control pilot study to explore the influence that the aerobic exercise regimen may have had on motor and cognitive outcomes in exercising and non-exercising (exercise-naïve) early-stage PD subjects. In accordance with the Declaration of Helsinki, the study received university Institutional Review Board approval and was conducted in a medical school laboratory setting.
Recruitment, inclusion/exclusion criteria
Using a community-based participatory research approach, subjects with early-stage PD were recruited from local PD non-profit organizations. Those consistently participating in aerobic exercise were recruited through the Paulie Ayala School of Hard Knocks Foundation in association with the Punching Out Parkinson’s (POPs) non-contact boxing regimen. Exercising PD subjects were those participating in aerobic exercise in the POPs program consisting of a minimum of 30-min non-contact boxing regimen delivered ≥3 times/week for ≥3 months. Non-exercise PD subjects were naïve to aerobic activity. Study inclusion criteria were: 1) Men and women of all race/ethnicities, 2) Age 50–90 years, 3) Early-stage PD, (≤1.5 Hoehn and Yahr [36], Movement Disorder Society (MDS) Clinical Diagnostic Criteria with a Unified Parkinson’s Disease Rating Scale section III Motor score of <18) [37], 4) Minimum high school education, 5) Ability to read, write, understand English and provide written informed consent. Volunteers were excluded if they had: 1) Visual, physical, and/or motor impairments that would prevent completing study procedures (i.e., obesity, non-ambulatory, blindness, uncorrected profound hearing loss, etc.), 2) Comorbid mild cognitive impairment or any neurodegenerative disease other than PD (i.e., Alzheimer’s disease, multiple sclerosis, Lewy body dementia, etc.); 3) Current tobacco use; 4) Lifetime history of substance use disorder; 5) Any unstable, moderate, or severe uncontrolled/unremitted/treatment-resistant/treatment non-responsive diseases (i.e., hypertension, cancer, kidney, hepatic, cardiovascular, respiratory, unremitted mental illness, etc.), and/or 6) Traumatic brain injury within the past 10-years. Non-exercising and exercising PD volunteers meeting all study criteria were allowed to enroll in the study after written informed consent was obtained.
Study protocol
Each PD volunteer provided a letter from their personal treating neurologist verifying that they were healthy enough to participate in the POPs program. The study physician (HA), principal investigator (VAN), research assistant (RJ), and doctoral students trained in neurological assessment (IS, KD) provided a consensus regarding PD stage using the Hoehn and Yahr Scale and the Unified Parkinson’s Disease Rating Scale (UPDRS) [36, 37]. To further verify study eligibility, subjects were interviewed to collect demographic, clinical indices, and general health information.
Psychiatric health was evaluated with the Mini Neuropsychological Interview Version 6.0 [38] to exclude those with unremitted mental illness and substance use disorders as per study eligibility criteria. The Geriatric Depression Rating Scale [39] was used to verify subjects mental state was within normal range (0–9). The Mini-Mental State Exam (MMSE; 24 minimum score) was used to exclude subjects with mild cognitive impairment and dementia [40]. All assessments were conducted with subjects in the ON medication state.
Motor and cognitive assessments
Regular exercise has been described as a facilitator of neurogenesis preventing age-related cognitive and motor decline associated with increased volume in orbitofrontal, prefrontal, frontoparietal, temporal cortex, hippocampus, globus pallidus, and striatum [41]. Thus, we further explored the influence a consistent aerobic exercise regimen had on motor and cognitive functioning as a surrogate neural phenotype. Motor performance was measured with well-established assessments used in PD research [Timed Up and Go (TUG) and 6-Minute Walk Test (6MWT)] while Trail–making Test A and B (TMT-A, TMT-B) were chosen to examine executive functioning differences between exercisers and non-exercisers [42–45]. TMT A and B is a widely used standardized tool with normative values used in previous PD research paradigms to assess cognitive flexibility, attention, and inhibitory control [42–45].
Aerobic exercise regimen and cardiovascular data collection
The POPs program was facilitated by a certified trainer and the aerobic exercise protocol began with a 10–15-min stretching and muscle warm-up followed by ∼30 min of supervised continuous rotations alternating through 7 stations (e.g., speed bag, heavy bag, footwork, trainer mit dodging, and bobbing, etc.). Subjects then completed their regimen with 10- to 15-min resistance training with a cool-down phase. Cardiovascular measures were taken twice at the pre- and post-exercise periods with readings taken within the first 2 min after completion of the last component of the aerobic exercise regimen (minimum ∼30 min of aerobic exercise). At both the pre- and post-exercise timepoints, PD subject’s HR and systolic and diastolic BP (SBP and DBP, respectively) were measured twice while seated, and the average HR, SBP, and DBP were calculated and recorded. Other than resting HR, SBP, DBP recorded in non-exercising PD subjects and calculated VO2 max, no other physiological measures were collected from non-exercising PD subjects as we were not employing exercise as an intervention in a clinical trial. To maintain the translatability between rats and humans, and as cardiovascular problems are inherent in some PD patients, we also calculated mean arterial pressure (MAP) to characterize parasympathetic and sympathetic tone, general heart health, cellular oxygenation, and blood flow at rest prior to aerobic activity and again after their exercise regimen in exercising PD subjects. MAP was determined using the formula: MAP = 1/3 * SBP + 2/3 * DBP.
Statistics
Descriptive statistics, frequency distributions, and Chi-square analyses were used to characterize the sample, as appropriate. First, we analyzed HR within exercising PD subjects to discern pre- and post-exercise HR function using a paired samples t-test. A percent change in HR was also calculated. SBP and DBP were used to derive MAP (using the formula described earlier) to ensure that no subjects were outside the normative values for cardiovascular health. As there were more men than women subjects, we used general linear modeling (GLM) univariate analysis to examine sex/gender as an independent variable and again as a covariate in GLM multivariate analyses to identify potential differences in UPDRS motor scores, years of PD, years of exercise (in exercisers), and levodopa dose that may have influenced motor and cognitive outcomes. Exercising and non-exercising PD groups were analyzed using GLM multivariate analysis to explore TUG, 6MWT, TMT A and B outcomes between these groups. All statistics were conducted using a two-tailed probability (p < 0.05) and a 95% Confidence Interval.
Pink1 KO rat study
After approval from the Institutional Animal Care and Use Committee at the University of North Texas Health Science Center was obtained, a 2-month longitudinal study was designed to evaluate cardiovascular function during treadmill exercise. Three-month-old male Long Evans rats, wild type (n = 6) and PINK1 KO (n = 6), were obtained from Horizon Labs (now Envigo) and singly housed in a 12-h reverse light-dark cycle in ambient environment. Food and water was provided ad libitum throughout of the study. Upon arrival, the subjects were acclimated to the colony for one week. Both groups were handled daily (1–3 min) for two weeks prior to the beginning of the exercise regimen, except for a period of three days after the surgical implant of the heart rate monitor. Rats exercised at 4 months old, with locomotor assessment following completion of the entire exercise regimen at three time intervals at 5 months old.
A second cohort of male Long-Evans rats, wild-type (n = 7) and Pink1 KO (n = 7) (also received from Envigo), was evaluated for locomotor activity at monthly intervals from age 3 to 6 months old. This cohort was used as an age-matched, exercise-naïve, cohort to determine if genotype or aging affected locomotor activity at the same age range as the exercise cohort.
Genotype confirmation
Genomic DNA was extracted from cerebellum extracts taken from KO and WT rats to confirm Pink1 gene knockout. The GenElutetrademark Mammalian Genomic DNA Miniprep kit was used with minor changes to the procedure. The tissue was digested for 24 h, only 50μL of elution solution was used, and the elution solution was incubated at room temperature on the column for 10 min. In order to confirm the 26 bp deletion, primers flanking the target sequence were used. The forward primer sequence used was 5′-CCCTGGCTGACTATCCTGAC, and the reverse primer sequence used was 5′-CCACCACCCACTACCACTTACT. The 50μL PCR reaction was comprised of 5μL of 10μM Pink1 forward primer, 5μL of 10μM Pink1 reverse primer, 14μL of nuclease-free water, 1μL genomic DNA, and 25μL of JumpStarttrademark Taq ReadyMixtrademark (Sigma-Aldrich, Cat.No. P2893). A negative control was also run with the same components except for the DNA. Instead, 1μL extra of nuclease-free water was added to the negative control reaction. The PCR cycling conditions consisted of six steps. The initial denaturation step was set as 95°C for 5 min. This was followed by 35 cycles of denaturation at 95°C for 30 s, annealing at 56°C for 30 s, and elongation at 72°C for 40 s. The final elongation step was set as 72°C for 5 min. The final step was a hold at 4°C. The PCR products were run on a 5% TBE polyacrylamide gel in 1x TBE buffer for about 1 h at 100 V. Wild-type bands were 109 bp while knockout bands were 83 bp (Supplementary Figure 1).
Aorta transmitter implant
Cardiovascular parameters were recorded using a Data Sciences International (DSI) radio telemetry system as previously described [35]. Each rat received a transmitter (model S10) and the signals were recorded using DSI telemetry receivers and MX2 matrix with DSI Ponemah Acquisition Software. Transmitter implantations were performed while the rats were anesthetized with 2% Isoflurane and kept warm on a water pad. A midline incision was made on the abdomen and the abdominal aorta and vena cava were isolated. 2-0 silk sutures were positioned above renal artery and right above the iliac bifurcation to temporarily occlude blood flow. A 23-gauge needle was used to introduce the blood pressure telemetry transmitter catcher about 2 cm. Vetbond was used to secure the catheter to the aorta and the transmitter was sutured in the peritoneal cavity on the muscle wall using 4-0 prolene sutures. The incisions were closed using 3-0 vicryl antimicrobial sutures. Animals were allowed to wake under a heat lamp with supervision. They were monitored and recovered for a minimum of 1 week prior to further studies.
Cardiovascular data acquisition
HR and MAP data were collected at multiple time points throughout the entirety of the study; 1) prior to exercise acclimation, 2) after exercise acclimation, 3) 5 min immediately prior to each exercise session, on back-to-back days for each treadmill speed, 4) 5 min immediately after each exercise session, 5) an evaluation of HR between the treadmill without and with incline portions of the study to determine the impact of anticipation to exercise when home cages of rats were transported to the exercise room (without the implementation of treadmill exercise), as previously reported [35]. Collection of HR and MAP data was not possible while the rats were running during the exercise regimen, as the signal strength of the implanted transmitters was not sufficient to be transmitted through the treadmill. Thus, the cardiovascular evaluations took place immediately before and after the exercise sessions in the home cage, wherein the transducer was located underneath the home cage for data collection on a minute-to-minutefrequency.
Treadmill exercise protocol
Treadmill exercise was conducted over a 6-week period during the active (dark) period of the light-dark cycle, and all experiments were concluded before 19:00. Rats were euthanized one month after exercise and completion of locomotor assessment. Euthanasia was by decapitation following very brief isoflurane exposure.
The exercise regimen had three separate phases, following previously described procedures [15, 35, 46]: pre-acclimation, treadmill acclimation, and treadmill exercise with progressive increase in speed (8 to 16 m/min at 2 m/min increments) at no incline, and then, repeating the regimen again at a 5° incline. Prior to beginning the pre-acclimation phase, HR and MAP were measured to establish baseline measures and determine if any genotype differences were present. During pre-acclimation, the rats were placed on a stationary treadmill each day, for three days, for 7 min, 8 min, and 10 min subsequently. During the acclimation phase, the rats were trained on a moving treadmill, starting at 7 m/min for 5 min, then 10 min the following day. This schedule was repeated with incremental increases in speed (2 m/min up) to 13 m/min, and then 14 m/min for 10 min and 15 min for the final two days of acclimation.
The treadmill exercise phase followed acclimation. The HR and MAP were recorded in one min intervals in their home-cage for five min prior to initiating exercise on the treadmill to establish respective pre-exercise HR and MAP. These results were used to evaluate anticipated conditioning effects on baseline HR and MAP as exercise sessions increased. The rats were then placed on the treadmill (no incline) to warm-up for 5 min at a speed 2 m/min slower than the final speed (8, 10, 12, 14, or 16 m/min) for the final 30 min of the session. Immediately after exercise, rats were removed from the treadmill and placed in their home-cage to record HR and MAP for 5 min. The exercise schedule was four times per week, increasing exercise speed by 2 m/min after two back-to- back daily sessions, with one- or two-days rest between increasing speeds, until completion. The regimen was then repeated using the same speeds, starting at 8 m/min, but with a treadmill incline at 5°.
Establishing baseline HR and MAP
In our previous exercise impact study using aged rats, we found that HR or MAP will increase ∼5–15% in response to merely being placed in the exercise environment on a stationary treadmill [35]. Thus, we evaluated whether the change in environment created an anticipatory effect that could potentially confound our evaluation of exercise on baseline HR. We first used linear regression to determine slope deviation in each genotype (previously described under “cardiovascular data acquisition procedure”) by determining if the HR would decrease over time after relocation of rats from their home colony (Supplementary Figure 2). Second, we compared the HR obtained immediately before exercise at each treadmill speed versus the mean HR value obtained from pre- and post-acclimation, and in-between no incline and incline sessions, of which there was no within-genotype difference among these three measures (Supplementary Fig. 3). Treadmill environment increased HR in both evaluations (Supplementary Fig. 4). Therefore, the baseline HR was established taking the mean of three HR measures taken at pre- and post-acclimation, and the interval between the no incline and with incline phases of the study to evaluate exercise impact on HR (as percent of baseline). To evaluate influence of the treadmill environment on MAP, we compared the pre-acclimation MAP result against the pre-exercise results taken 5 min prior to exercise during the progressive treadmill speed regimen.
Exercise compliance assessment
Compliance to exercise was recorded on a scale from 0–4 during each session. A score of 4 indicated exercise for at least 75% of the time without being prompted, as previously described [15, 35, 46]. A score of 0 indicated that the rat was unable to continuously exercise without prompting. Non-compliance was defined as not keeping all four limbs in contact with the treadmill and/or resting against the backstop for ∼5 s. After this interval, the periods of time of non-compliance was noted in 5- and 10-s intervals and then the rat was prompted to continue exercising using the backstop (Supplementary Figure 5A). The total amount of time of non-compliance was added together at the end of the bout of exercise for each rat and subtracted from the total time of exercise to obtain a compliance score.
Assessment of locomotor activity
Spontaneous locomotor activity was assessed in a darkened room while the rats were in their awake cycle, as previously reported [15, 35]. Rats were placed into automated activity chambers with fresh bedding, surrounded by a grid of infrared beams to record activity automatically (VersaMax Animal Activity Monitoring System, Columbus Instruments, Inc., Columbus, OH). Total distance (DT, cm) was evaluated in 1-h sessions in three separate sessions. The average of three 1-h long, once daily, sessions was used to establish locomotor activity levels (as total distance) for each rat. The exercise cohort was evaluated at 5 months old, at three time intervals (3 days, 2 weeks, and 4 weeks) following cessation of all exercise. The exercise-naïve cohort was evaluated at 3, 4, 5, and 6 months old.
Assessment of dopamine and tyrosine hydroxylase
The striatum, SN, nucleus accumbens, and ventral tegmental area were dissected on wet-ice, immediately cooled on dry-ice, and saved for high performance liquid chromatography (HPLC) to analyze dopamine (DA), dihydroxyphenylacetic acid (DOPAC), and further processed for western blot analysis of total tyrosine hydroxylase protein against a calibrated standard curve for quantification against total protein. The methodological guidelines have been extensively published by our lab [19].
Statistics
HR and MAP results were analyzed for genotype differences and exercise impact across session number (session number co-varied with treadmill speed), and if an interaction between genotype and session number/treadmill speed occurred using a repeated measures 2-way ANOVA. Within groups comparisons across exercise session or treadmill speed were evaluated using a Dunnett’s or Tukey’s multiple comparisons test and between groups evaluations used Bonferroni multiple comparison’s test. These analyses were done on raw HR and MAP results, as well as on the calculated percent of baseline.
Locomotor activity was evaluated in repeated measures 2-way ANOVA, matching locomotor results for each rat across multiple time points in the exercise and exercise-naïve cohorts. In the exercise cohort, within genotype evaluations were further analyzed with Tukey’s multiple comparison test. In the exercise-naïve cohort, within genotype evaluations were further analyzed with Dunnett’s Multiple Comparison Test and between genotype evaluations were further analyzed with Bonferronit’s multiple comparison test. The Grubb’s test was used to identify outliers in each set of results with alpha set to 0.05.
RESULTS
Human subjects
Demographic and clinical characteristics
Out of 47 volunteers, we obtained evaluable data on motor and cognitive outcomes on 21 exercising PD subject who met all study criteria (women N = 9; men N = 12) with 19 self-identifying as Non-Hispanic White and 2 as Hispanic White. The mean age was 68.71±7.15 and the mean number of years of education was 16.36±1.80. The mean number of years of having a PD diagnosis was 4.49±4.91 and the levodopa equivalent daily dose was 561.55±425.53 mg. The mean number of years subjects had been regularly exercising was 9.45±14.95. Years of regular exercise were not statistically different between men and women (p = 0.084). Non-exercising PD subjects consisted of 7 women and 17 men (N = 24) who self-identified as Non-Hispanic White (N = 18), Hispanic (N = 2), or Black (N = 4). The mean age was 71.33±8.56 and the mean number of years of education was 15.81±2.14. The mean number of years of having a PD diagnosis was 3.1±3.12 and the levodopa equivalent daily dose was 577.37±435.09 mg.
Between the exercising vs. non-exercising groups, Pearson Chi-square analyses showed no significant group differences in sex/gender [χ2(1, N = 45) = 0.916, p = 0.34] or race/ethnicity [χ2(2, N = 45) = 3.84, p = 0.15]. GLM multivariate analyses showed there were no significant group differences in age (p = 0.28), years of education (p = 0.36), years of PD diagnosis (p = 0.24), levodopa equivalent daily dose (p = 0.90), UPDRS motor score (p = 0.68), or HR (p = 0.11).
GLM multivariate analyses showed there was a ∼11% increase in the number of meters PD exercisers walked during the 6MWT (M = 319.08±47.81) compared to non-exercisers (M = 287.15±69.37), although this finding was not statistically significant [F(1,42) = 3.05, p = 0.088]. In contrast, when comparing TUG performance between the groups, we found that exercising PD subjects took significantly less time to arise from a seated position and start walking (M = 7.31±1.52) than non-exercisers (M = 9.40±3.44) [F(1,42) = 5.93, p = 0.019]. The normative TUG mean score lower and upper bound range [95% C.I.] is 9.25–10.55 for 50- to 59-year-old healthy individuals. Unexpectedly, we found that the TUG scores for exercising PD subjects (M = 7.31±1.52) indicates that their performance is better than or comparable to 20–29-year old healthy adults whose lower and upper bound range [95% C.I.] is 8.18–8.97 s [47]. Even the non-exercising PD subjects’ TUG scores (M = 9.40±3.44) suggests that they are performing as if they do not have PD and are within the range of subjects who are a decade younger [47]. This result suggests that we indeed assessed PD in the earliest stage of the disease.
Cognitive flexibility, attention, and response inhibition comparing TMT A and B showed that exercising PD subjects took significantly less time to complete TMT-A (M = 36.82±10.35) than non-exercisers (M = 46.23±14.48), [F(1,42) = 5.51, p = 0.024]. Exercising PD subjects completed the more complex TMT-B in less time (M = 99.03,±50.70) than non-exercisers (M = 120.65±61.95) although this result was not statistically significant [F(1,42 = 1.37, p = 0.249].
To eliminate potential bias due to the unequal group distribution of men and women, we analyzed sex/gender as a covariate with GLM and found no influence on TUG (p = 0.50), 6MWT (p = 0.93), TMT-A (p = 0.58), or TMT-B (p = 0.59) scores. Therefore, our results not only indicate that we captured motor functioning in the earliest disease stage, but the data suggest that non-contact boxing aerobic exercise consisting of at least 30 min, 3x/wk, for 3 months may be a minimum threshold for improving or maintaining motor and cognitive functioning that one might expect to find in prodromal PD. Although we cannot explore the human neurobiological mechanisms in this paradigm, the neuroprotective effects of aerobic exercise, as observed in motor and cognitive functioning, in this proof-of-concept study appear evident.
Cardiovascular metrics and aerobic exercise impact in early-stage PD
Prior to motor and cognitive evaluation, volunteers in the exercising PD group (N = 24), the mean pre-exercise HR as 71.1±10.4 beats per min (BPM) and 94.6±16.2 BPM in the post-exercise condition resulting in an overall ∼33% increase in HR [t(23) = 6.71, p < 0.0001] (Fig. 1). The average age of the participants (68.71 years old) was used in an established formula [220 minus age] to calculate the intensity of physical activity in our sample. We found the maximum HR estimate was ∼151 BPM for the sample. With an overall mean post-exercise HR to ∼96 BPM, the intensity of physical activity is estimated to be ∼67% of maximum HR (151 BPM), which qualifies in the range of a moderate-intensity activity level.
Fig. 1
Non-contact boxing-training exercise regimen impact on HR in early-stage human PD subjects. Mean pre-exercise (Pre-Ex) baseline HR in early-stage PD patients immediately prior to participation in the aerobic exercise regimen was 71.1±10.4 beats per min (BPM) and 94.6±16.2 BPM in the post-exercise (Post-Ex) condition resulting in ∼33% increase in HR [t(23) = 6.71, p < 0.0001].
![Non-contact boxing-training exercise regimen impact on HR in early-stage human PD subjects. Mean pre-exercise (Pre-Ex) baseline HR in early-stage PD patients immediately prior to participation in the aerobic exercise regimen was 71.1±10.4 beats per min (BPM) and 94.6±16.2 BPM in the post-exercise (Post-Ex) condition resulting in ∼33% increase in HR [t(23) = 6.71, p < 0.0001].](https://content.iospress.com:443/media/jpd/2022/12-6/jpd-12-6-jpd223157/jpd-12-jpd223157-g001.jpg)
In the exercising PD group, pre-exercise SBP = 144.19 and DBP = 80.76 resulted in MAP = 102 mm Hg. The post-exercise SBP = 132.14 and DBP = 74.60 resulted in MAP = 93 mm Hg. In the non-exercising PD group, basal SBP = 134.27 and DBP = 75.77 resulted in MAP = 96 mm Hg. The pre- and post-exercise MAP results were within desirable normal limits of 90–119 for SBP mm Hg and 60–79 mm Hg for DBP (American Heart Association) for both the exercising and non-exercising groups [48]. Even though MAP was within desirable normal limits, we noted the pre-exercise SBP and DBP seemed higher than we expected especially given the study criteria that strictly excluded untreated, unremitted, uncontrolled hypertension and cardiovascular disease. According to the American College of Physicians, the newest hypertension threshold guidelines categorized by age for initiating pharmacotherapy is SBP >150 in >60 year olds [48]. Given the fact the post-exercise SBP and DBP for 60+ year olds were closer to the threshold recommended by the American College of Cardiology (130/80), we suggest that anticipatory effects were present for the pre-exercise blood pressure measures that began to normalize after moderate intensity aerobic exercise. This result mirrors the anticipatory effects in rodents [35]. The decrease in SBP and DBP in the post-exercise may reflect a positive conditioning effect of consistent participation in the non-contact boxing regimen (>30-min×3/wk/3 months), again, mirroring findings in our study in aged rats [35].
Rat PD model
Pink1 KO and Long-Evans wild-type studies: Exercise compliance
During the first week of exercise acclimation, all rats were compliant to the gradual implementation of treadmill exercise, running at least 75% of the allotted time by the start of the second week of acclimation, with compliance increasing to at least 94% of exercise time by the end of the acclimation period. At the conclusion of the exercise sessions on flat or incline, there was no significant difference in compliance between the genotypes, with 73% of WT and 87% of Pink1 KO rats showing 97% overall compliance to the exercise regimen at all speeds for the 30 min duration after warm-up without incline (t = 1.49, p = 0.15, df = 18; Supplementary Figure 5B). However, at the 14 or 16 m/min speeds on the incline, 50% of the rats maintained >97% compliance, with the remaining half of each group compliant to these speeds 80–90% of the time during the 30-min exercise session. Thus, despite considerable experience with treadmill exercise, the rats began to approach limits to optimal treadmill compliance at the highest intensity levels used.
Pink1 KO and Long-Evans wild-type studies: Exercise impact on HR
The baseline HR of the Pink1 KO was significantly greater (∼15%) as compared with the age-matched WT prior to, and after, acclimation to the exercise regimen (Fig. 2A, Supplementary Figure 3). The mean HR pre- and post-acclimation in the WT was 337 and 354 BPM, and in the Pink1 KO was 395 and 397 BPM, respectively.
Fig. 2
Exercise impact on Pink1 KO and WT rat cardiovascular dynamics: heart rate (as beats per minute (BPM)). A) Genotype difference in heart rate (HR). The Pink1 KO rat had significantly greater HR (as BPM) than the age-matched WT prior to, and after, exercise acclimation. genotype (F(1,10) = 36.5, p = 0.0001); exercise acclimation status (F(1,9) = 1.42, ns); genotype×exercise acclimation status (F(1,9) = 0.79, ns). WT vs. KO, pre-acclimation (t=5.05, ***p = 0.0001); post-acclimation (t = 3.59, **p = 0.004), Bonferroni’s multiple comparison test. B. Progressive treadmill exercise and HR as percent of baseline. Pink1 KO rats had significantly less increase in baseline HR following exercise. Genotype (F(1,10) = 29.37, p = 0.0003). Session number or treadmill speed affected the increase in HR, as percent of baseline (F(4,40) = 11.07, p < 0.0001); genotype×session number F(4,40) = 0.21, ns). Dunnett’s multiple comparison comparing session number against HR at treadmill speed (8 m/min) vs. 10 m/min (WT, q = 0.25, ns, KO q = 1.30, ns); vs. 12 m/min (WT, q = 2.27, p = 0.09; KO q = 2.47, p = 0.06); vs. 14 m/min (WT, q = 3.24, **p = 0.009; KO q = 3.63, **p = 0.003); vs. 16 m/min (WT, q = 3.30, **p = 0.007; KO q = 4.29, ***p = 0.0004). C) Progressive treadmill exercise and HR. The number of exercise sessions had a highly significant effect on HR (as BPM). As the number of sessions increased, there was a smaller increase in BPM detected immediately following exercise (F(4,40) = 10.89, p < 0.0001). There was no difference between genotype (F(1,10) = 1.71, ns); genotype×exercise session F(4,40) = 0.23, ns). Dunnett’s multiple comparison comparing session number against HR at treadmill speed (8 m/min) vs. 10 m/min (WT, q = 0.25, ns, KO q = 1.30, ns); vs. 12 m/min (WT, q = 2.27, p = 0.09; KO q = 2.47, p = 0.06); vs. 14 m/min (WT, q = 3.24, **p = 0.009; KO q = 3.63, **p = 0.003); vs. 16 m/min (WT, q = 3.30, **p = 0.007; KO q = 4.29, ***p = 0.0004). D) Exercise session number impact on pre-exercise HR. As the number of exercise sessions increased, baseline HR (as BPM) captured immediately prior to the exercise session decreased. Exercise session number (F(4,40) = 6.70, p = 0.0003). There was no difference between genotype (F(1,10) = 1.72, ns), or interaction between genotype×exercise session number F(4,40) = 0.67, ns). Dunnett’s multiple comparison comparing mean session numbers, both genotypes; 1,2 vs.. 3,4 (q = 0.27, ns); vs. 5,6 (q = 0.57, ns); vs. 7,8 (q = 0.69, ns); vs. 9,10 (q = 4.24, **p = 0.0005).
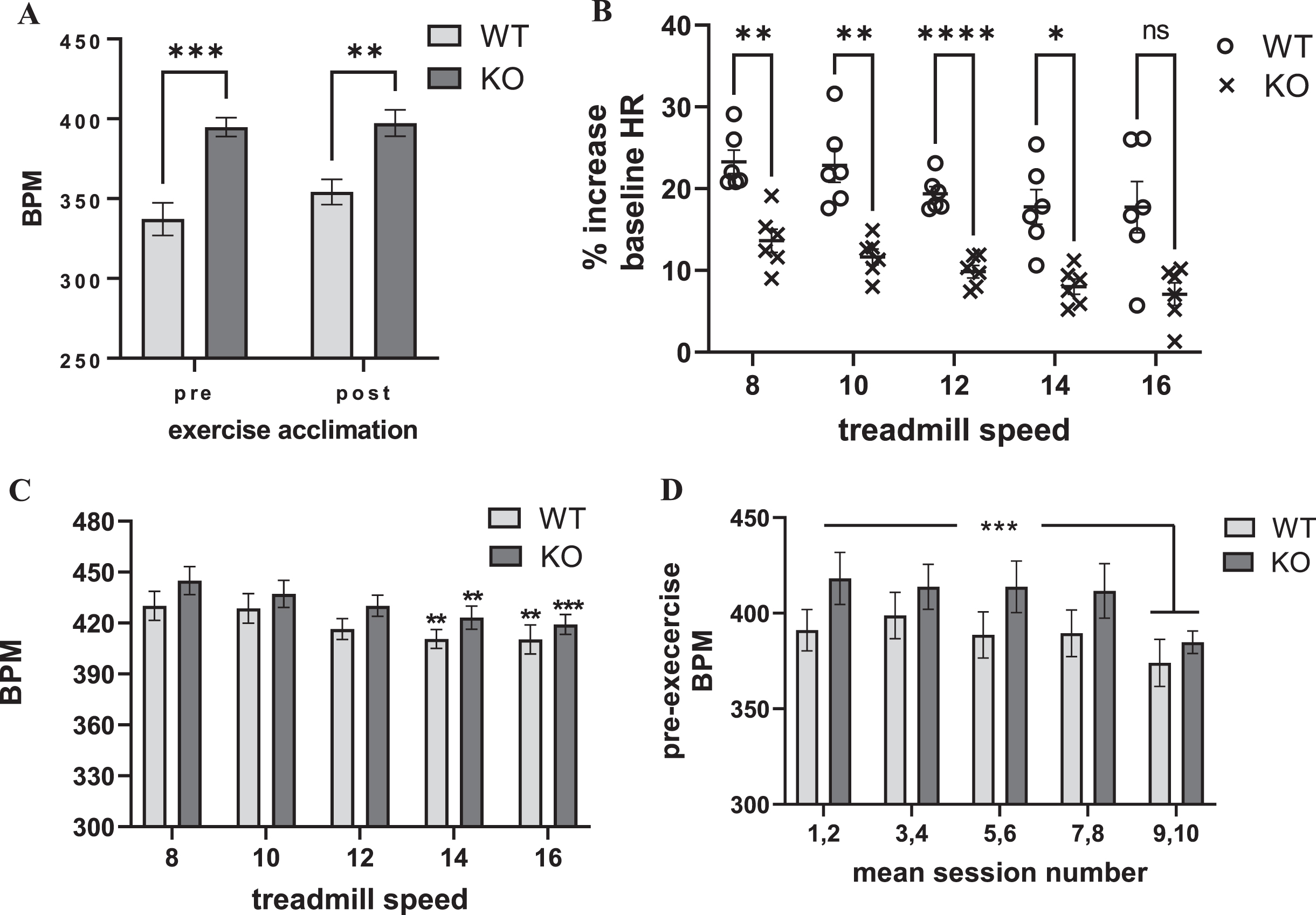
Within the first two min immediately after exercise, HR increased above baseline levels between 10–25% at each treadmill speed (Fig. 2B). There was a highly significant difference in the HR responses to exercise between the Pink1 KO and WT (Fig. 2B). Compared to the WT, the Pink1 KO had significantly attenuated responses to exercise relative to baseline HR. This difference was likely due to the higher baseline HRs observed in the Pink1 KO (Fig 2A). This possibility was supported by the observation that the exercise HR increased to the same absolute values in both genotypes at any treadmill speed (Fig. 2C). The exercise regimen did produce a highly-significant increase in HR within the first 2 min after treadmill exercise completion, with no effect of treadmill speed (Supplementary Fig. 6). The progressive increases in treadmill speed, unexpectedly, did not produce correlative increases in HR, as measured by percentage changes from baseline (Fig. 2B), or actual BPM (Fig. 2C, Supplementary Figure 6). Instead as treadmill speed increased, the increase in HR following exercise steadily declined (Fig. 2B, C).
Maximal heart rate in rats is estimated to be ∼650 BPM [49]. Therefore, we estimate that the rats achieved between 60–70% of their maximum HR at the lowest treadmill speed of 8 m/min during the 30 min regimen. The unexpected decrease in the exercise-related HR response associated with increasing either numbers of exercise sessions or the treadmill speed was likely due to, in part, conditioning from the exercise regimen as exercise progressed. As the number of exercise sessions increased, there was a significant decrease in the pre-exercise baseline HR, in both genotypes, evident by the 9th and 10th session of exercise, prior to running at the 16 m/min speed (Fig. 2D).
The additional exertion required from running on the 5° incline did not further increase HR after exercise (Supplementary Figure 7). Moreover, there was also no further decrease in the HR that was observed at the end of the exercise session conducted with no incline. Therefore, the conditioning produced by the exercise sessions indicates maximal impact of exercise on HR may be achieved at the lowest treadmill speeds employed (8 to 10 m/min) and this change is well-within the HR increase of early- stage PD patients and is in the moderate intensity range in both species.
Pink1 KO and Long-Evans wild-type studies: Exercise impact on MAP
Prior to exercise acclimation, similar to HR, MAP was slightly, but, significantly greater in the Pink1 KO (Fig. 3A). Immediately prior to exercise, there was an overall difference in MAP as compared to MAP taken at the pre-acclimation phase (Supplementary Figure 8). Therefore, we evaluated exercise effects on MAP as a percent of baseline using the pre-acclimation results, similar to the approach used to determine HR as percent of baseline.
Fig. 3
Exercise impact on Pink1 KO and WT rat cardiovascular dynamics: blood pressure (as mean arterial pressure (MAP)). A) Genotype difference in MAP. MAP was significantly greater in the KO (t = 2.58, p < 0.05). B) Progressive treadmill exercise and MAP as percent of baseline. MAP increased 5–15% following exercise across all treadmill speeds, without any significant difference between genotype (F(1,9) = 1.72, ns), session number (F(4,36) = 0.74, ns), or interaction between genotype×session number F(4,36) = 1.02, ns). C) Progressive treadmill exercise and MAP. MAP increased to 120 –135 following exercise across all treadmill speeds, without any significant difference between genotype (F(1,9) = 1.27, ns), session number (F(4,36) = 1.60, ns), or interaction between genotype×session number F(4,36) = 0.43, ns). D) Exercise session number impact on pre-exercise MAP. Baseline (immediately prior to exercise (Pre-EX) MAP was unaffected by number of exercise sessions. Exercise session number (F(4,36) = 0.76, ns). There was no difference between genotype (F(1,9) = 1.12, ns), or interaction between genotype×exercise session number F(4,36) = 2.45, p = 0.06).
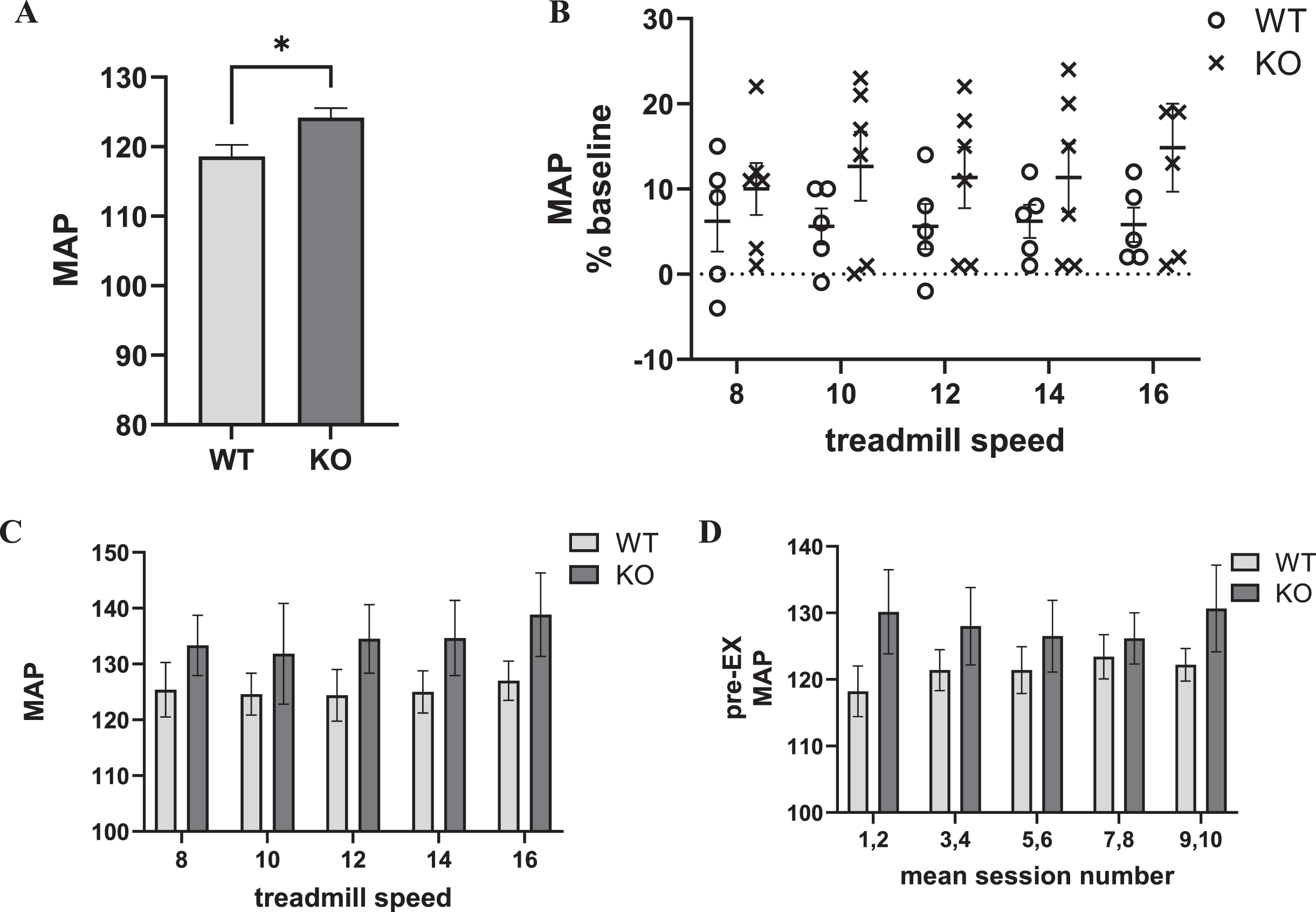
Within the first two min immediately after exercise, MAP increased above baseline levels between 5–15% at each treadmill speed, but without significant difference between genotypes or influence of exercise session number/treadmill speed (Fig. 3B). No genotype difference was observed in MAP following exercise (Fig. 3C) and no evidence of a conditioning effect of exercise on the MAP, unlike HR (Fig. 3D).
Locomotor activity
Decreased locomotor activity, reflecting parkinsonian signs, occur between 6- to 8-month-old in the Pink1 KO, as suggested by previous studies [26, 32]. Although the primary goal of the study was to determine the treadmill speed in a rat PD model that produced a similar increase in HR in an exercising early-stage PD patient, we also evaluated locomotor activity in the exercise cohort, after completing the entire regimen, and compared results to an exercise-naïve cohort at the same age (5 months old) in the lifespan. In the exercise-naïve cohort, there was a significant effect of age, and an interaction between age and genotype (Fig. 4A). However, locomotor decline occurred in the KO at 6, but not 5, months old (Fig. 4 A, B). Moreover, locomotor activity decline in the KO was highly variable, ranging from 39 to 100% of the baseline locomotor activity level taken at 3 months old (Fig. 4B).
Fig. 4
Locomotor activity in exercise-naïve cohort. A) Distance travelled. In an exercise-naïve cohort, there was a significant effect of age and an interaction between age and genotype on locomotor activity. Age (F(3,36) = 4.62, p = 0.008), age×genotype (F(3,36) = 5.24, p = 0.004), genotype (F(1,12) = 0.64, ns). Within group post-hoc. WT, all comparisons vs. 3 months, p > 0.05. KO, 3 vs. 4 months (q = 0.44, ns), 3 vs. 5 months (q = 0.1.14, ns), 3 vs. 6 months (q = 4.79, ****p < 0.0001). Dunnett’s Multiple Comparison Test. B) Locomotor Activity as percent of baseline. There was a highly significant effect of age and interaction between age and genotype on locomotor activity as a percentage of levels at 3 months old. Age (F(2,24) = 7.68, p = 0.003), age×genotype (F(2,24) = 5.65, p = 0.010), genotype (F(1,12) = 4.28, p = 0.061). Between group (WT vs. KO) post-hoc; 4 months (t = 1.13, ns), 5 months (t = 1.12, ns), 6 months (t = 3.33, **p = 0.006). Bonferroni’s multiple comparison test.
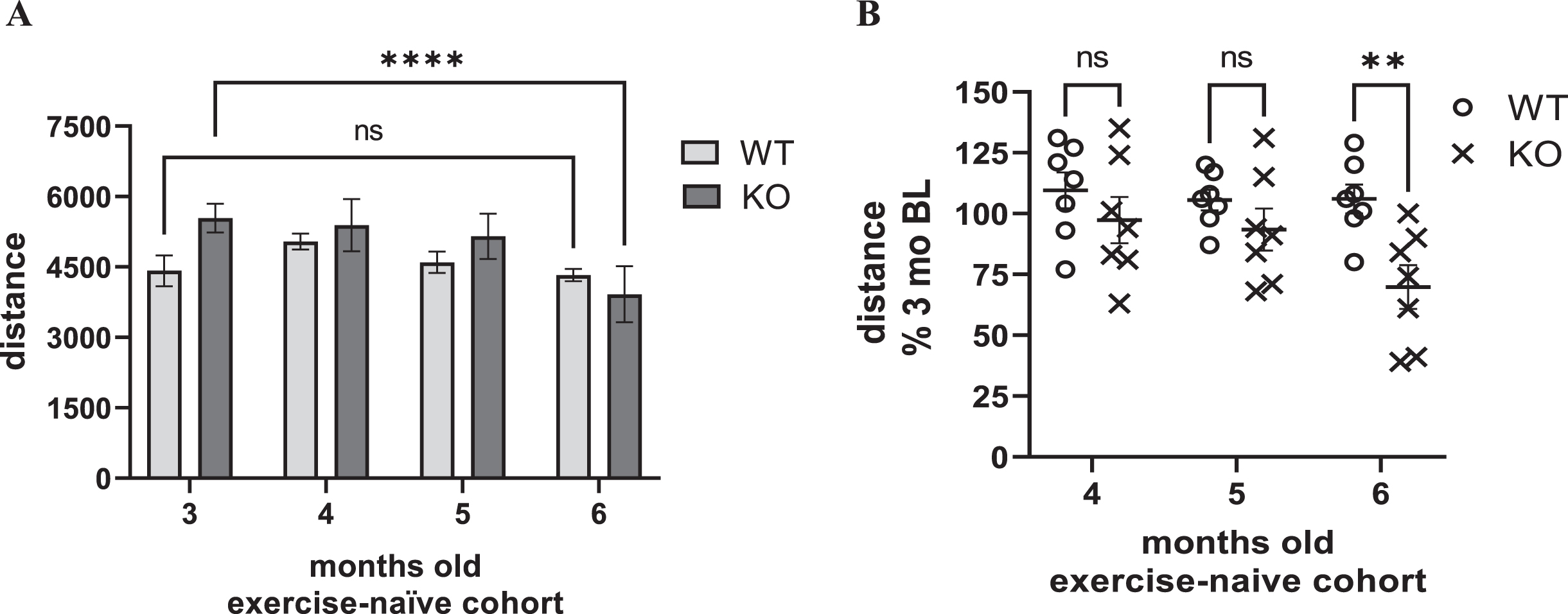
In the exercised cohort, there was a highly significant genotype difference in locomotor activity between the KO and WT, and an influence of length of time following completion of exercise (Fig. 5A). These effects were solely attributable to the KO, with significantly greater locomotor activity compared to the WT at 3 days and peaking at 2 weeks after exercise, but no difference after 4 weeks, suggesting a possible exercise effect in the KO that decreased as time following exercise increased (Fig. 5A). This difference was also evident within the KO, by greater locomotor activity at 2 weeks post-exercise, compared to 3 days or 4 weeks after exercise (Fig. 5A). The exercised KO also had significantly greater locomotor activity compared to the exercise-naïve KO at the same age of 5 months old, whereas this exercise effect was not seen in the WT (Fig. 5B). With the caveat that treadmill speed was not confined to one speed (as per the experimental design), it appears that exercise did increase locomotor activity in the Pink1 KO at an age just prior to the onset of locomotor decline shown in the exercise-naïve cohort.
Fig. 5
Locomotor activity in Pink1 KO vs. WT at 5 months-old, exercised and exercise-naïve vs. exercised. A) Exercised. After completing 20 sessions of 30 min exercise with progressively-increased treadmill speed, KO and WT rats were evaluated at three time points for open-field locomotor activity at 5 months old. Locomotor activity was significantly greater in KO vs. WT rats across multiple time points after cessation of the exercise regimen. Genotype (F(1,10) = 10.83, p = 0.008), time after final exercise session (F(2,20) = 7.96, p = 0.003), genotype×time after final exercise (F(2,20) = 0.90, ns). Within-group post-hoc results. WT, all comparisons p > 0.05. KO, 3 days vs. 2 wks (q = 4.34, *p = 0.016), 3 days vs. 4 wks (q = 0.17, ns), 2 wks vs. 4 wks (q = 4.17, *p = 0.021). Tukey’s multiple comparison test. Between-group, WT vs. KO, post-hoc results. 3 days (t = 3.14, #p = 0.035), 2 wks (t = 3.60, #p = 0.015), 4 wks (t = 2.11, ns). B) Exercise-naïve vs. exercised. At 5 months old, locomotor activity was not significantly different between the KO and WT in the exercise-naïve cohort, whereas locomotor activity 2 weeks after completion of the treadmill regimen in the exercised KO was significantly greater than the exercise-naïve KO and the exercised WT. One-way ANOVA between groups (F3,22) = 6.21, p = 0.003). Between group comparisons; EX-naïve WT vs. EX-naïve KO (t = 1.08, ns), EX-naïve WT vs. EX WT (t = 0.44, ns), EX-naïve KO vs. EX KO (t = 2.95, *p = 0.03), EX WT vs. EX KO (t = 3.42, **p = 0.001). Bonferroni’s multiple comparison test.
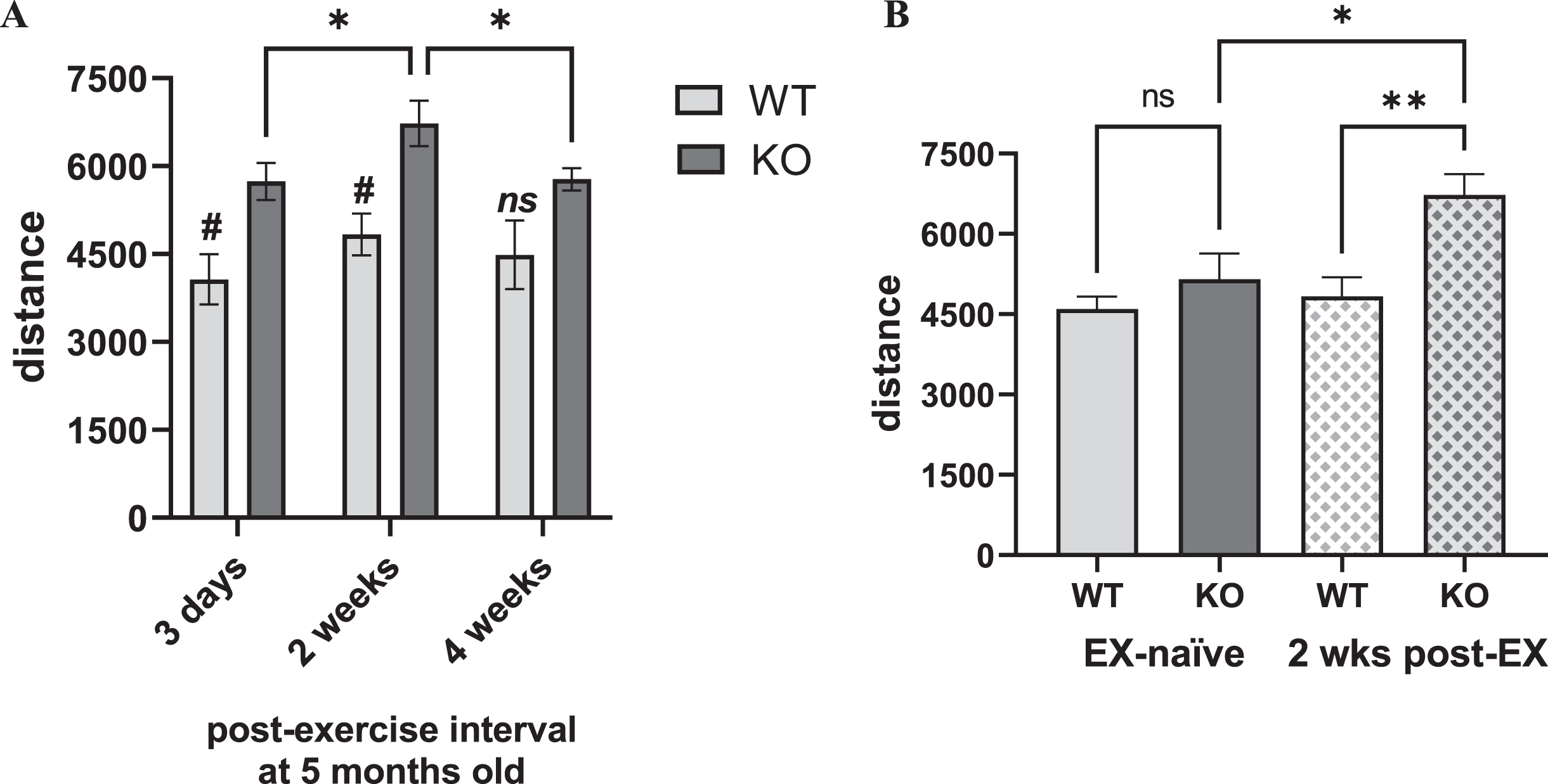
Markers of nigrostriatal dopamine-regulation
Following completion of locomotor assessments, we assessed DA tissue content, DA turnover and TH protein expression. There was no difference in TH protein expression in the striatum (Fig. 6A, C). Moreover, there was no difference in the SN as well (Fig. 6B, D). As others have reported TH protein loss specific to the SN in the Pink1 KO rat [26], we speculate the exercise experience may have initiated a protection against such loss.
Fig. 6
Genotype differences in tyrosine hydroxylase (TH) and dopamine (DA)-regulation 1 month after completing exercise. A-D) Tyrosine hydroxylase (TH) (as ng/μg total protein) in the striatum and SN. E, F) Dopamine (DA) tissue content. G, H) DA turnover (as DOPAC (ng/mg protein) per DA (ng/mg protein). Total TH. A) Striatum. TH levels were not significantly different between genotypes (t = 0.06, ns, df = 10). B) SN. In the same rats, TH levels were not significantly different between genotypes (t = 1.15, ns, df = 10). C) Representative TH quantification in striatum. Standard curve used to quantify total TH from 0.5 –3.0 ng total TH with representative pairs of WT and KO rat. D) Representative TH quantification in SN. Standard curve used to quantify total TH from 0.2 –1.5 ng total TH with representative pairs of WT and KO rat. [Note: blots were processed and imaged in different assays, and image development time varies according to average signal intensity.] DA tissue content: E) Striatum. DA levels were significantly less in the KO rat (t = 3.34, *p = 0.010, df = 8). F) SN. In the same rats, DA levels not significantly different between genotypes (t = 1.61, p = 0.14, df = 8). DA turnover: G) Striatum. DA turnover was significantly greater in the KO rat (t = 3.34, *p = 0.010, df = 8). H) SN. In the same rats, DA turnover was not significantly different between genotypes (t = 2.06, p = 0.07 (ns), df = 10).
![Genotype differences in tyrosine hydroxylase (TH) and dopamine (DA)-regulation 1 month after completing exercise. A-D) Tyrosine hydroxylase (TH) (as ng/μg total protein) in the striatum and SN. E, F) Dopamine (DA) tissue content. G, H) DA turnover (as DOPAC (ng/mg protein) per DA (ng/mg protein). Total TH. A) Striatum. TH levels were not significantly different between genotypes (t = 0.06, ns, df = 10). B) SN. In the same rats, TH levels were not significantly different between genotypes (t = 1.15, ns, df = 10). C) Representative TH quantification in striatum. Standard curve used to quantify total TH from 0.5 –3.0 ng total TH with representative pairs of WT and KO rat. D) Representative TH quantification in SN. Standard curve used to quantify total TH from 0.2 –1.5 ng total TH with representative pairs of WT and KO rat. [Note: blots were processed and imaged in different assays, and image development time varies according to average signal intensity.] DA tissue content: E) Striatum. DA levels were significantly less in the KO rat (t = 3.34, *p = 0.010, df = 8). F) SN. In the same rats, DA levels not significantly different between genotypes (t = 1.61, p = 0.14, df = 8). DA turnover: G) Striatum. DA turnover was significantly greater in the KO rat (t = 3.34, *p = 0.010, df = 8). H) SN. In the same rats, DA turnover was not significantly different between genotypes (t = 2.06, p = 0.07 (ns), df = 10).](https://content.iospress.com:443/media/jpd/2022/12-6/jpd-12-6-jpd223157/jpd-12-jpd223157-g006.jpg)
There was a significant decrease in striatal DA tissue content in the KO ∼43% (Fig. 6E), whereas in the SN, there was no significant difference in DA tissue content (Fig. 6F). There was also a significant increase in DA turnover of nearly 50% in the striatum (Fig. 6G), whereas a small, but insignificant increase (14%) was observed in the SN (Fig. 6H).
Interestingly, we also found a reduction in DA tissue content in the nucleus accumbens (Supplementary Figure 9) similar to that seen in the striatum, and no reduction in the ventral tegmental area, similar to that seen in the SN.
DISCUSSION
Regardless of the aerobic exercise modality, cardiovascular responses can be easily obtained to identify the intensity needed to gauge exercise efficacy. Establishing cardiovascular metrics associated with exercise intensity can serve as a corollary of cross-species neurobiological mechanisms in translational investigations. In this proof-of-concept study using early-stage PD patients and the Pink1 KO rat model, we have established translatable cardiovascular parameters and exercise intensity equivalence within the range of moderate intensity.
Importantly, we found maximal cardiovascular impact of exercise using an 8 to 10 m/min treadmill speed in the Pink1 KO rat model and age-matched WT (both reaching 66–68% maximum HR) that were in the range of that produced by an aerobic exercise regimen in early-stage PD subjects (∼63% maximum HR). The maximum HR percentage in our PD subjects meets a recently defined universal prescription of aerobic exercise for PD populations of 3x/week, 30–40 min, with a 60–80% HR reserve and only a slightly lower HR max than the 70–85% reported by Alberts and Rosenfeldt [58].We anticipate that the neurobiological mechanisms activated with aerobic exercise at an intensity of 8–10 m/min in a Pink1 rat PD model would also manifest in human PD, given equal exercise duration (∼30–40 min) and frequency (3 sessions per week). Such translation is vital to identify and define the key neurobiological mechanisms engaged by exercise that could alleviate disease progression, particularly as the ability to consistently exercise may decrease with disease severity or encountering barriers to exercise [10–14]. With this knowledge we can identify the endogenous neurobiological mechanisms engaged by an effective aerobic exercise regimen to reveal the best molecular targets for developing pharmacological or genetic therapies to improve motor or cognitive function, possibly at any stage of PD.
The protracted nature of PD progression requires a longitudinal evaluation of aerobic exercise to reveal the timing and duration of its efficacy [9, 15, 17, 35]. Given the slower rate of motor decline reported in the Pink1 KO rat [26, 32] and as shown in our study, a longitudinal evaluation of exercise impact in this model would reveal the extent to which exercise could delay onset of motor decline, or reverse decline if already established. To that end, our data from the 6-month-old exercise-naïve cohort indicate that the 4-month-old KO rats that exercised on the treadmill did so prior to onset of locomotor decline. As such, the current dataset only allows for us to speculate if the aerobic exercise experience could prove neuroprotective, as we did not observe any difference in TH expression in the SN, unlike another study which reported loss beginning at ∼6 months old [26], and an increase in locomotor activity in the exercised KO. However, in our previous longitudinal study, we found that exercise prevented parkinsonian symptom onset in aged rats commensurate with a HR increase of 15–30% above baseline [35]. Thus, as PD is an aging-related disease, we expect this regimen in aging Pink1 KO rats would be comparably effective, even though these rats were chronologically young. Notwithstanding the caveats imposed by the varying treadmill speed in the experimental design, we found evidence that the exercise regimen increased motor activity in the KO rats, being significantly more active than their exercise-naïve KO counterparts. We also may have found a time limit to exercise efficacy in the KO, as the increase in locomotor activity lasted 2 weeks, but not 4 weeks, after completing a 30-days exercise regimen, and notable the CNS tissue was taken at that time point. Taken together, our data indicate that consistent aerobic exercise may condition the KO rat to forestall the onset of eventual locomotor decline, as evidenced in the exercise-naïve cohort.
We are only beginning to scratch the surface of understanding the neurobiological mechanisms activated or modified by exercise in the PD patient [50–52]. This is primarily due to limited resources available to image the brain with great enough detail to discern the impact exercise may have to increase dopamine signaling [50, 51, 53]. However, by standardizing parameters of cardiovascular functioning in response to aerobic exercise, rat PD models can play a major role to reveal dopaminergic and other neurobiological mechanisms [54–57] that may extend to the exercising PD patient, particularly in the context of evaluating the long-term effects of aerobic exercise against the natural progression of PD [10, 58]. Consistent with these priorities, we met our goal in bridging a mechanistic gap between the Pink1 rat model and early-stage PD subjects.
A variety of aerobic-based exercise therapies (e.g., dancing, non-contact boxing, cycling, etc.) exist for the PD patient [4, 5]. The early-stage PD subjects in our study met the criterion of a moderate intensity level of 63% maximum HR (previously mentioned 220 BPM –mean age metric provided by the CDC), with a ∼35% increase above baseline (pre-exercise) HR. With a similar duration of exercise (30 min), the rats HR was maximally increased above baseline HR at ∼25% in the WT, and ∼10–15% in the KO) at the 8 to 10 m/min treadmill speed. The difference in HR increase was attributable to a higher baseline HR in the KO, and earlier work showing that young rats have a maximum rat HR of ∼650 BPM [49] indicates that the HR of the WT rats (∼430 BPM) and Pink1 KO rats (∼445 BPM) represents 66–68% maximum HR. This is in range of the 63% maximum HR in our PD subjects, and from the perspective of cross-species translation, meets a recently defined universal prescription of aerobic exercise for PD populations of 3x/week, 30–40 min, with a 60–80% HR reserve and only a slightly lower HR max than the 70–85% reported by Alberts and Rosenfeldt (2020) [58]. Although, the progressive increase in treadmill speed or incline was expected to produce a commensurate increase in HR, we did not see any further increase in HR in either genotype presumably due to a conditioning effect, as evidenced by the decrease in maximal HR following exercise at the highest treadmill speeds. This conditioning effect of HR with exercise has been reported by other groups [49, 59, 60] and also observed in humans at moderate intensity levels [61]. Therefore, given that aerobic-based exercise programs like non-contact boxing can provide a satisfying and motivating experience to continue exercise, we expect long-term practice should result in improved quality of life [9], motor and cognitive function [9, 16, 62]. Moderate intensity exercise is clinically relevant for PD patients as Eijsvogels et al. (2016) reported higher intensity levels can be detrimental to the point of sudden death even in physically fit athletes and has reduced rehabilitation effects in cardiovascular disease patients [63].
Using the Pink1 KO model was important for a number of reasons. First, Pink1, along with Parkin has been identified as an autosomal recessive mutation [22–24] that plays a major role in mitophagy [20, 21, 64] influencing downstream homeostasis of dopamine, glutamate, calcium, and antioxidants [65–70] as well as normal cardiac tissue homeostasis [71, 72]. Pink1 KO mice develop cardiac hypertrophy and left ventricular dysfunction [71]. It is possible that the parameters of the exercise paradigm used in this study were not rigorous enough to exacerbate similar cardiovascular changes in Pink1 KO rats. There also is evidence that augmented mitochondrial function plays a role in exercise-related changes in mitophagy in cardiac tissue [73, 74], which posits the Pink1 KO as a valuable model to determine the extent to which mitophagy affects exercise-related recovery of motor or cognitive functions. This includes the counteractive mechanisms of exercise-related increases in inflammatory processes, as proposed by Sliter and colleagues [75]. This possibility is further supported by accumulating evidence that enhanced mitophagy or other critical mitochondrial functions play a role in exercise-related recovery in CNS tissue and neurodegenerative disease models [76–81]. Thus, the Pink1 rat model provides a logical approach for future investigations into the role of mitophagy may play in exercise-related recovery of motor function in PD models in humans and in rats without strenuous and potentially detrimental over-exercising. To our knowledge, this is the first evaluation of exercise impact in Pink1 KO rats, and despite indications for cardiovascular differences in the absence of Pink1, these rats will exercise thus enabling the likelihood of evaluating exercise impact longitudinally [13, 16, 17, 35].
Study strengths and limitations
The primary strength in this study is that we have established translatable cardiovascular parameters to match aerobic exercise intensity equivalence in a rat PD model and human PD with behavioral impact at moderate intensity. Thus we propose these parameters may be applicable to identify the neurobiological mechanisms of aerobic exercise efficacy underlying motor and cognitive improvements in PD. Other strengths include the addition of early-stage PD subjects participating in a well-controlled exploratory study comparing motor and cognitive function in PD exercisers and non-exercisers. The study was well-controlled in that all subjects were tested in the “ON” medication state, restricted to only early-stage, and demographic variables were statistically controlled to eliminate confounds that may have influenced motor and cognitive outcomes. Here, we found that with consistent moderate intensity aerobic exercise (non-contact boxing), exercising PD subjects were quicker to rise and walk (TUG), took more steps during 6-minutes (6MWT), and were quicker to make accurate decisions (TMT-A, B) than non-exercising PD subjects.
Nevertheless, although we have established several strengths, some study limitations are nevertheless noted. As our sample of PD subjects were highly functioning, determining whether our cardiovascular results could be applicable in more advanced stages of PD needs further examination. Thus, any cardiovascular issues that may arise during future stages of PD progression [82] cannot yet be observed with the post-exercise HR we reported. However, in this proof-of-concept design, we were more concerned about reducing possible confounds so that we could readily identify translatable cross-species cardiovascular parameters as a starting point, prior to examining cardiovascular indices in more advanced stages of PD. From the rat model perspective, the Pink1 KO exercise-naïve rat did not exhibit motor decline until 6 months old, consistent with previous studies [26, 32]. However, this too can be considered a strength in that this is new knowledge for future investigations to consider in longitudinal study designs. As such, it is plausible that application of this treadmill exercise regimen in Pink1 KO rats could delay decreased locomotor activity if initiated before 6 months old, or reverse the decrease if initiated after 6 months old, given the increase in locomotor activity in the exercised Pink1 KO rat versus the exercise-naïve KO rat and the outcomes found in the exercising PD subjects.
It is not clear if cardiovascular responses seen between the human and Pink1 model would continue to be applicable at more severe stages, preventing firm establishment of external validity in the advanced stages of the disease. Yet to our knowledge, no studies exist reporting atypical cardiovascular symptoms, or on the effects of aerobic activity on Pink1-PD human subjects that may be used to compare Pink1 cardiovascular indices to idiopathic PD. From reported clinical information, PD arising from a Pink1 mutation is indistinguishable from sporadic PD, aside from average age of onset [83]. Therefore at this time, we can infer that cardiovascular differences in the Pink1 KO rat model are applicable to sporadic PD cases, such as our study population.
Finally, the applicability of the Pink1 KO results to the more widely-used toxin-based models is yet unknown. However, changes in cardiovascular indices have been examined in rat PD toxin-based models [84] and presumably the 8 to 10 m/min treadmill would be comparably effective to stall motor decline or promote recovery, as shown in aging rats [15, 35]. It should be noted that the rapid nature of nigrostriatal neuron loss in a toxin rat model could limit interpretation of exercise efficacy against such loss and motor decline compared to a protracted longitudinal study.
Summary
We have established cardiovascular metrics related to aerobic-based exercise in both early-stage PD patients and a rat genetic PD model to create a launch point of equivalency in exercise intensity and duration to apply in translation research paradigms. The rationale for our study was fueled by the premise that the neurobiological mechanisms to be unveiled in the Pink1 rat PD model has the greatest translation when the exercise parameters applied adhere to the physical capabilities of the PD patient. We have recently substantiated a relationship for aerobic exercise intensity, as measured by HR, with mitigation of parkinsonian signs in aging rats [15, 35]. Therefore, these studies establish a platform upon which to optimize mechanistic findings in Pink1 rat PD models for translation to the human PD patient. This would include the feasibility of initiating exercise when subclinical non-motor signs of PD may be present [85–89]. Finally, the Pink1 KO rat exhibits near 100% compliance at exercise intensities in the moderate range. As such, this genetic rat PD model could be suitable for longitudinal evaluations to determine exercise efficacy and corollary neurobiological mechanisms to align with the PD patient.
ACKNOWLEDGMENTS
The work was funded in part by the Department of Defense Parkinson’s Disease Research Program (Award W81XWH-19-1-0757) to MFS, The Institute for Healthy Aging at University of North Texas Intramural Award to MFS, VAN, and JTC. IS is supported by the National Institute on Aging Training Grant T32 AG 020494. We also recognize Mr.Chris McElroy for technical support.
CONFLICT OF INTEREST
The authors have no conflict of interest to report.
SUPPLEMENTARY MATERIAL
[1] The supplementary material is available in the electronic version of this article: https://dx.doi.org/10.3233/JPD-223157.
REFERENCES
[1] | Ridgel AL , Vitek JL , Alberts JL ((2009) ) Forced, not voluntary, exercise improves motor function in Parkinson’s disease patients. Neurorehabiol Neural Repair 23: , 600–608. |
[2] | Ridgel AL , Ault DL ((2019) ) High-cadence cycling promotes sustained improvement in bradykinesia, rigidity, and mobility in individuals with mild-moderate Parkinson’s disease. Parkinsons Dis 2019: , 4076862. |
[3] | Tiihonen M , Westner BU , Butz M , Dalal SS ((2021) ) Parkinson’s disease patients benefit from bicycling–a systematic review and meta-analysis. NPJ Parkinsons Dis 7: , 86. |
[4] | Schenkman M , Moore CG , Kohrt WM , Hall DA , Delitto A , Comella CL , Josbeno DA , Christiansen CL , Berman BD , Kluger BM , Melanson EL , Jain S , Robichaus JA , Poon C , Corcos DM ((2018) ) Effect of high- intensity treadmill exercise on motor symptoms in patients with de novo Parkinson’s disease: A phase II randomized clinical trial. JAMA Neurol 75: , 219–226. |
[5] | Shulman LM , Katzel LI , Ivey FM , Sorkin JD , Favors K , Anderson KE , Smith Bam Reich SG , Wiener WJ , Macko RF ((2013) ) Randomized clinical trial of 3 types of physical exercise for patients with Parkinson disease. JAMA Neurol 70: , 183–190. |
[6] | Bearss KA , DeSouza JF ((2021) ) Parkinson’s disease motor symptom progression slowed with multisensory dance learning over 3-years: A preliminary longitudinal investigation. Brain Sci 11: , 895. |
[7] | Hasan SM , Alshafie S , Hasabo EA , Saleh M , Elnaiem W , Qasem A , Alzu’bi YO , Khlaed A , Zaazouee MS , Ragab KM , Nourelden AZ , Doheim MF ((2021) ) Efficacy of dance for Parkinson’s disease: A pooled analysis of 372 patients. J Neurol 269: , 1195–1208. |
[8] | Combs SA , Diehl MD , Staples WH , Conn L , Davis K , Lewis N , Schaneman K ((2011) ) Boxing training for patients with Parkinson disease: A case series. Phys Ther 91: , 132–142. |
[9] | Horbinski C , Zumpf KB , McCortney K , Eoannou D ((2021) ) Longitudinal observational study of boxing therapy in Parkinson’s disease, including adverse impacts of the COVID-19 lockdown systematic review and meta-analysis. BMC Neurol 21: , 326. |
[10] | Kanegusuku H , Ritti-Dias RM , Barbosa P , das Neves Guelfi ET , Okamoto E , Miranda CS , de Paula Oliveira T , Piemonte M ((2021) ) Influence of motor impairment on exercise capacity and quality of life in patients with Parkinson disease. J Exerc Rehabil 17: , 241–246. |
[11] | Ellis T , Rochester L ((2018) ) Mobilizing Parkinson’s disease: The future of exercise. J Parkinsons Dis 8: , S95–S100. |
[12] | Afshari M , Kianirad Y , Bega D ((2016) ) Motivators and barriers to participation in exercise in Parkinson’s disease. J Parkinsons Dis 7: , 703–711. |
[13] | Ellis T , Boudreau JK , DaAngelis TR , Brown LE , Cavanaugh JT , Earhart GM , Ford MP , Foreman KB , Dibble LE ((2013) ) Barriers to exercise in people with Parkinson disease. Phys Ther 93: , 628–636. |
[14] | Schootemeijer S , van der Kolk NM , Ellis T , Mirelman A , Nieuwboer A , Nieuwhof F , Schwarzschild MA , de Vries NM , Bloem BR ((2020) ) Barriers and motivators to engage in exercise for persons with Parkinson’s disease. J Parkinsons Dis 10: , 1293–1299. |
[15] | Arnold JC , Cantu MA , Kasanga EA , Nejtek VA , Papa EV , Bugnariu N , Salvatore MF ((2017) ) Aging- related limit of exercise efficacy on motor decline. PLoS One 12: , e0188538. |
[16] | Chen K , Tan Y , Lu Y , We J , Liu X , Zhao Y ((2020) ) Effect of exercise on quality of life in Parkinson’s Disease: A systematic review and meta-analysis. Parkinsons Dis 2020: , 3257623. |
[17] | Zeiss CJ , Allore HG , Beck AP ((2017) ) Established patterns of animal study de sign undermine translation of disease-modifying therapies for Parkinson’s disease. PLoS One 12: , e0171790. |
[18] | Barker RA , Björklund A ((2020) ) Animal models of Parkinson’s disease: Are they useful or not? . J Parkinsons Dis 10: , 1335–1342. |
[19] | Salvatore MF ((2014) ) Ser31 tyrosine hydroxylase phosphorylation parallels differences in dopamine recovery in nigrostriatal pathway following 6-OHDA lesion. J Neurochem 129: , 548–558. |
[20] | Youle RJ , Narendra DP ((2011) ) Mechanisms of mitophagy. Nat Rev Mol Cell Biol 12: , 9–14. |
[21] | Pickrell AM , Youle RJ ((2015) ) The roles of PINK1, parkin, and mitochondrial fidelity in Parkinson’s disease. Neuron 85: , 257–273. |
[22] | Valente EM , Abou-Sleiman PM , Caputo V , Muqit MM , Harvey K , Gispert S , Ali Z , Del Turco D , Bentivoglio AR , Healy DG , Albanese A , NussbaumR , González-Maldonado R , Deller T , Salvi S , Cortelli P , Gilks WP , Latchman DS , Harvey RJ , Dallapiccola B , Auburger G , Wood NW ((2004) ) Hereditary early-onset Parkinson’s disease caused by mutations in PINK1. Science 304: , 1158–1160. |
[23] | Bentivoglio AR , Cortelli P , Valente EM , Ialongo T , Ferraris A , Elia A , Montagna P , Albanese A ((2001) ) Phenotypic characterization of autosomal recessive PARK6-linked parkinsonism in three unrelated Italian families. Mov Disord 16: , 999–1006. |
[24] | Ricciardi L , Petrucci S , Guidubaldi A , Ialongo T , Serra L , Ferraris A , Spano B , Bozzali M , Valente EM , Bentivoglio AR ((2014) ) Phenotypic variability of Pink1 expression: 12 years’ clinical follow-up of two Italian families. Mov Disord 29: , 1561–1566. |
[25] | Hedrich K , Hagenah J , Djarmati A , Hiller A , Lohnau T , Lasek K , Grünewald A , Hilker R , Steinlechner S , Boston H , Kock N , Schneider-Gold C , Kress W , Siebner H , Binkofski F , Lencer R , Münchau A , Klein C ((2006) ) Clinical spectrum of homozygous and heterozygous Pink1 mutations in a large German family with Parkinson disease. Arch Neurol 63: , 833–838. |
[26] | Dave KD , De Silva S , Sheth NP , Ramboz S , Beck MJ , Quang C , Switzer RC , Ahmad SO , Sunkin SM , Walker D , Cui X , Fisher DA , McCoy AM , Gamber K , Ding X , Goldberg MS , Benkovic SA , Haupt M , Baptista MA , Fiske BK , Sherer TB , Frasier MA ((2014) ) Phenotypic characterization of recessive gene knockout rat models of Parkinson’s disease. Neurobiol Dis 70: , 190–203. |
[27] | Kelm-Nelson CA , Lechner SA , Lettenberger SE , Kaldenberg T , Pahapill NK , Regenbaum A , Ciucci MR , ((2021) ) Pink1-/- rats are a useful tool to study early Parkinson disease. Brain Commun 3: , fcab077. |
[28] | Creed RB , Menalled L , Casey B , Dave KD , Janssens HB , Veinbergs I , van der Hart M , Rassoulpoor A , Goldberg MS ((2019) ) Basal and evoked neurotransmitter levels in Parkin, DJ-1, PINK1, and LRRK2 knockout rat striatum. Neurosci 409: , 169–179. |
[29] | Ren X , Butterfiled DA ((2021) ) Fidelity of the Pink1 knockout rat to oxidative stress and other characteristics of Parkinson disease. Free Radic Biol Med 163: , 88–101. |
[30] | Grigoruta M , Martinez-Martinez A , Dagda RY , Dagda RK ((2020) ) Psychological stress phenocopies brain mitochondrial dysfunction and motor deficits as observed in a parkinsonian rat model. Mol Neurobiol 57: , 1781–1798. |
[31] | Zhi L , Qin Q , Muqeem T , Seifert EL , Liu W , Zheng S , Li C , Zhang H ((2019) ) Loss of Pink1 causes age-dependent decrease of dopamine release and mitochondrial dysfunction. Neurobiol Aging 75: , 1–10. |
[32] | de Hass R , Heltzel LCMW , Tax D , van den Broek P , Steenbreker H , Verheij MMM , Russel FGM , Orr AL , Nakamura K , Smeitink JAM ((2019) ) To be or not to be pink(1): Contradictory findings in an animal model for Parkinson’s disease. Brain Commun 1: , fcz016. |
[33] | Francardo V ((2018) ) Modeling Parkinson’s disease and treatment complications in rodents: Potentials and pitfalls of the current options . . Behav Brain Res 352: , 142–150. |
[34] | Cenci MA , Sgambato V (2021) Toxin-based rodent models of Parkinson’s disease. In Clinical Trials in Parkinson’s Disease Neuromethods, vol. 160, Perez- Lloret S, eds. Humana, New York, NY. |
[35] | Kasanga EA , Little J , McInnis TR , Bugnariu N , Cunningham JT , Salvatore MF ((2021) ) Cardiovascular metrics associated with preventing aging-related parkinsonian signs following exercise intervention in sedentary older rats. }. Front Aging Neurosci 13: , 775355. |
[36] | Hoehn MM , Yahr MD ((1967) ) Parkinsonism: Onset, progression and mortality. Neurology 17: , 427–442. |
[37] | Movement Disorder Society Task Force on Rating Scales for Parkinson’s Disease ((2003) ) The Unified Parkinson’s Disease Rating Scale (UPDRS): Status and recommendations. Mov Disord 18: , 738–750. |
[38] | Sheehan DV , Lecrubier Y , Sheehan KH , Amorim P , Janavs J , Weiller E , Hergueta T , Baker R , Dunbar GC ((1998) ) The Mini-International Neuropsychiatric Interview (M.I.N.I.): The development and validation of a structured diagnostic psychiatric interview for DSM-IV and ICD-10. Clin Psychiatry 59: (Suppl 20), 22–57. |
[39] | Yesavage JA , Brink TL , Rose TL , Lum O , Huang V , Adey M , Leirer VO ((1982) ) Development and validation of a geriatric depression screening scale: A preliminary report. J Psychiatr Res 17: , 37–49. |
[40] | Crum RM , Anthony JC , Bassett SS , Folstein MF ((1993) ) Population-based norms for the Mini-Mental State Examination by age and educational level. JAMA 269: , 2386–2391. |
[41] | Maugeri G , D’Agata V , Trovato B , Roggio F , Castorina A , Vecchio M , Di Rosa M , Musumeci G ((2021) ) The role of exercise on peripheral nerve regeneration: From animal model to clinical application. Heliyon 7: , e08281. |
[42] | Cruise KE , Bucks RS , Loftus AM , Newton RU , Pegoraro R , Thomas MG ((2011) ) Exercise and Parkinson’s: Benefits for cognition and quality of life. Acta Neurol Scand 123: , 13–19. |
[43] | Ridgel AL , Kim CH , Fickes EJ , Muller MD , Alberts JL ((2011) ) Changes in executive function after acute bouts of passive cycling in Parkinson’s disease. J Aging Phys Act 19: , 87–98. |
[44] | Duchesne C , Lungu O , Nadeau A , Robillard ME , Bore A , Bobeuf F , Lafontaine AL , Gheysen F , Bherer L , Doyon J ((2015) ) . Enhancing both motor and cognitive functioning in Parkinson’s disease: Aerobic exercise as a rehabilitative intervention. Brain Cogn 99: , 68–77. |
[45] | Picelli A , Varalta V , Melotti C , Zatezalo V , Fonte C , Amato S , Saltuari L , Santamato A , Fiore P , Smania N ((2016) ) Effects of treadmill training on cognitive and motor features of patients with mild to moderate Parkinson’s disease: A pilot, single-blind, randomized controlled trial. Funct Neurol 31: , 25–31. |
[46] | Arnold JC , Salvatore MF ((2014) ) Getting to compliance in forced exercise in rodents: A critical standard to evaluate exercise impact in aging-related disorders and disease. Journal of visualized experiments. JoVE (90) 51827. |
[47] | Kear BM , Guck TP , McGaha AL ((2017) ) Timed Up and Go (TUG) test: Normative reference values for ages 20 to 59 years and relationships with physical and mental health risk factors. J Prim Care Community Health 8: , 9–13. |
[48] | Qaseem A , Wilt TJ , Rich R , Humphrey LL , Frost J , Forciea MA , Fitterman N , Barry MJ , Horwitch CA , Iorio A , McLean RM ((2017) ) Pharmacologic treatment of hypertension in adults aged 60 years or older to higher versus lower blood pressure targets: A clinical practice guideline from the American College of Physicians and the American Academy of Family Physicians. Ann Intern Med 166: , 430–437. |
[49] | Wisløff U , Helgerud J , Kemi OJ , Ellingsen O ((2001) ) Intensity- controlled treadmill running in rats: VO2max and cardiac hypertrophy. Am J Physiol Heart Circ Physiol 280: , H11–H1310. |
[50] | Sacheli MA , Neva JL , Lakhani B , Murray DK , Vafai N , Shahinfard E , English C , McCormick S , Dinelle K , Neilson N , McKenzie J , Schulzer M , McKenzie DC , Appel-Cresswell S , McKeown MJ , Boyd LA , Sossi V , Stoessl AJ ((2019) ) Exercise increases caudate dopamine release and ventral striatal activation in Parkinson’s disease. Mov Disord 34: , 1891–1900. |
[51] | Fisher BE , Li Q , Nacca A , Salem GJ , Song J , Yip J , Hui JS , Jakowec MW , Petzinger GM ((2013) ) Treadmill exercise elevates striatal dopamine D2 receptor binding potential in patients with early Parkinson’s disease. Neuroreport 24: , 509–514. |
[52] | Silva-Batista C , de Lima-Pardini AC , Nucci MP , Coelho DB , Batista A , Silva-Batista C , de Lima-Pardini AC , Nucci MP , Coelho DB , Batista A , Piemonte MEP , Barbosa ER , Teixeira LA , Corcos DM , Amaro E Jr , Horak FB , Ugrinowitsch C ((2020) ) A randomized, controlled trial of exercise for parkinsonian individuals with freezing of gait. Mov Disord 35: , 1607–1617. |
[53] | Earhart GM , Duncan RP , Huang JL , Perlmutter JS , Pickett KA ((2015) ) Comparing interventions and exploring neural mechanisms of exercise in Parkinson disease: A study protocol for a randomized controlled trial. BMC Neurol 15: , 9. |
[54] | Arnold JC , Salvatore MF ((2016) ) Exercise-mediated increase in nigral tyrosine hydroxylase is accompanied by increased nigral GFR-α1 and EAAC1 expression in aging rats. ACS Chem Neurosci 7: , 227–239. |
[55] | Smith BA , Goldberg NR , Meshul CK ((2011) ) Effects of treadmill exercise on behavioral recovery and neural changes in the substantia nigra and striatum of the 1-methyl-1,2,3,6-tetrahydropyridine- lesioned mouse. Brain Res 1386: , 70–80. |
[56] | Yoon MC , Shin MS , Kim TS , Kim BK , Ko IG , Sung YH , Kim SE , Lee HH , Kim YP , Kim CJ ((2007) ) Treadmill exercise suppresses nigrostriatal dopaminergic neuronal loss in 6-hydroxydopamine- induced Parkinson’s rats. Neurosci Lett 423: , 12–17. |
[57] | Gorton LM , Vuckovic MG , Vertelkina N , Petzinger GM , Jakowec MW , Wood RI ((2010) ) Exercise effects on motor and affective behavior and catecholamine neurochemistry in the MPTP-lesioned mouse. Behav Brain Res 213: , 253–262. |
[58] | Alberts JL , Rosenfeldt AB ((2020) ) The universal prescription for Parkinson’s disease: Exercise. J Parkinsons Dis 10: (1) S21–S27. |
[59] | Musch TI , Moore RL , Smaldone PG , Riedy M , Zelis R ((1989) ) Cardiac adaptations to endurance training in rats with a chronic myocardial infarction. J Appl Physiol 66: , 712–719. |
[60] | Høydal MA , Wisløff U , Kemi OJ , Ellingsen O ((2007) ) Running speed and maximal oxygen uptake in rats and mice: Practical implications for exercise training. Eur J Cardiovasc Prev Rehabil 14: , 753–760. |
[61] | Dawes TJ , Corden B , Cotter S , de Marvao A , Walsh R , Ware JS , Cook SA , O’Regan DP ((2016) ) Moderate physical activity in healthy adults is associated with cardiac remodeling. Circ Cardiovasc Imaging 9: , e004712. |
[62] | Larson D , Yeh C , Rafferty M , Bega D (2021) High satisfaction and improved quality of life with Rock Steady Boxing in Parkinson’s disease: Results of a large-scale survey. Disabil Rehabil. doi: 10.1080/09638288.2021.1963854. |
[63] | Eijsvogels TM , Molossi S , Lee DC , Emery MS , Thompson PD ((2016) ) Exercise at the extremes: The amount of exercise to reduce cardiovascular events. J Am Coll Cardiol 67: , 316–329. |
[64] | Chen G , Kroemer G , Kepp O ((2020) ) Mitophagy: An emerging role in aging and age-associated diseases. Front Cell Dev Biol 8: , 200. |
[65] | Creed RB , Roberts RC , Farmer CB , McMahon LL , Goldberg M ((2021) ) Increased glutamate transmission onto dorsal striatum spiny projection neurons in Pink1 knockout rats. Neurobiol Dis 150: , 105246. |
[66] | Gandhi S , Wood-Kaczmar A , Yao Z , Plun-Favreau H , Deas E , Klupsch K , Downward J , Latchman DS , Tabrizi SJ , Wood NW , Duchen MR , Abramov AY ((2009) ) PINK1-associated Parkinson’s disease is caused by neuronal vulnerability to calcium-induced cell death. Mol Cell 33: , 627–638. |
[67] | DeAngelo VM , Hilliard JD , McConnell GC ((2022) ) Dopaminergic but not cholinergic neurodegeneration is correlated with gait disturbances in Pink 1 knockout rats. Behav Brain Res 417: , 113575. |
[68] | Kelm-Nelson CA , Trevino MA , Ciucci MR ((2018) ) Quantitative analysis of catecholamines in the Pink1 -/- rat model of early-inset Parkinson’s disease. Neuroscience 379: , 126–141. |
[69] | Maynard ME , Redell JB , Kobori N , Underwood EL , Fischer TD , Hood KN , LaRoche V , Waxham MN , Moore AN , Dash PK ((2020) ) Loss of PTEN- induced kinase 1 (Pink1) reduces hippocampal tyrosine hydroxylase and impairs learning and memory. Exp Neurol 323: , 113081. |
[70] | Ren X , Hinchie A , Swomley A , Powell DK , Butterfield DA ((2019) ) Profiles of brain oxidative damage, ventricular alterations, and neurochemical metabolites in the striatum of Pink1 knockout rats as functions of age and gender: Relevance to Parkinson disease. Free Radic Biol Med 143: , 146–152. |
[71] | Billia F , Hauck L , Konecny F , Rao V , Shen J , Mak TW ((2011) ) PTEN-inducible kinase 1 (PINK1)/Park6 is indispensable for normal heart function. Proc Natl Acad Sci U S A 108: , 9572–9577. |
[72] | Siddall HK , Yellon DM , Ong SB , Mukherjee UA , Burke N , Hall AR , Angelova PR , Ludtmann MH , Deas E , Davidson SM , Mocanu MM , Hausenloy DJ ((2013) ) Loss of PINK1 increases the heart’s vulnerability to ischemia-reperfusion injury. PloS One 8: , e62400. |
[73] | Li H , Qin S , Liang Q , Xi Y , Bo W , Cai M , Tian Z ((2021) ) Exercise training enhances myocardial mitophagy and improves cardiac function via irisin/FNDC5-PINK1/parkin pathway in MI mice. Biomedicines 9: , 701. |
[74] | Zhao Y , Zhu Q , Song W , Gao B ((2018) ) Exercise training and dietary restriction affect PINK1/Parkin and Bnip3/Nix-mediated cardiac mitophagy in mice. Gen Physiol Biophys 37: , 657–666. |
[75] | Sliter DA , Martinez J , Hao L , Chen X , Sun N , Fischer TD. , Burman JL , Li Y , Zhang Z , Narendra DP , Cai H , Borsche M , Klein C , Youle RJ ((2018) ) Parkin and PINK1 mitigate STING-induced inflammation. Nature 561: , 258–262. |
[76] | Koo JH , Cho JY , Lee UB ((2017) ) Treadmill exercise alleviates motor deficits and improves mitochondrial import machinery in an MPTP-induced mouse model of Parkinson’s disease. Exp Gerontol 89: , 20–29. |
[77] | Zhao N , Yan QW , Xia J , Zhang XL , Li BX , Yin LY , Xu B ((2020) ) Treadmill exercise attenuates Aβ-induced mitochondrial dysfunction and enhances mitophagy activity in APP/PS1 transgenic mice. Neurochem Res 45: , 1202–1214. |
[78] | Ferreira AFF , Binda KH , Singulani MP , Pereira CPM , Ferrari GD , Alberici LC , Real CC , Britto LR ((2020) ) Physical exercise protects against mitochondria alterations in the 6-hidroxydopamine rat model of Parkinson’s disease. Behav Brain Res 387: , 112607. |
[79] | Kwon I , Jang Y , Lee Y ((2021) ) Endurance exercise-induced autophagy/mitophagy coincides with a reinforced anabolic state and increased mitochondrial turnover in the cortex of young male mouse brain. J Mol Neurosci 1: , 42–54. |
[80] | Silva AS , Ariza D , Dias DPM , Crestani CC , Martins-Pinge MC ((2015) ) Cardiovascular and autonomic alterations in rats with Parkinsonism induced by 6-OHDA and treated with L-DOPA. Life Sci 127: , 82–89. |
[81] | Raefsky SM , Mattson MP ((2017) ) Adaptive responses of neuronal mitochondria to bioenergetic challenges: Roles in neuroplasticity and disease resistance. Free Radic Biol Med 102: , 203–216. |
[82] | Salvatore MF , Disbrow EA , Emborg ME ((2014) ) Peripheral and cognitive signs: Delineating the significance of impaired catecholamine metabolism in Parkinson’s disease progression. J Neurochem 131: , 129–133. |
[83] | Ibáñez P , Lesage S , Lohmann E , Thobois S , De Michele G , Borg M , Agid Y , Dürr A , Brice A , French Parkinson’s Disease Genetics Study Group ((2006) ) Mutational analysis of the PINK1 gene in early-onset parkinsonism in Europe and North Africa. Brain 129: (Pt 3) 686–694. |
[84] | Silva AS , Ariza D , Dias DP , Crestani CC , Martins-Pinge MC ((2015) ) Cardiovascular and autonomic alterations in rats with Parkinsonism induced by 6-OHDA and treated with L-DOPA. Life Sci 127: , 82–89. |
[85] | Nejtek VA , James RN , Salvatore M , Alphonso HM , Boehm GW ((2021) ) Premature cognitive decline in specific domains found in young veterans with mTBI coincide with elder normative scores and advanced-age subjects with early-stage Parkinson’s disease. PloS One 16: , e0258851. |
[86] | da Silva FC , Iop RR , de Oliveira LC , Boll AM , de Alvarenga JGS , Gutierres Filho PJB , de Melo LMAB , Xavier AJ , da Silva R ((2018) ) Effects of physical exercise programs on cognitive function in Parkinson’s disease patients: A systematic review of randomized controlled trials of the last 10 years. PLoS One 13: , e0193113. |
[87] | Gonzalez-Latapi P , Bayram E , Litvan I , Marras C ((2021) ) Cognitive impairment in Parkinson’s disease: Epidemiology, clinical profile, protective and risk factors. Behav Sci 11: , 74. |
[88] | Salvatore MF , Soto I , Alphonso H , Cunningham R , James R , Nejtek VA ((2021) ) Is there a neurobiological rationale for the utility of the Iowa Gambling Task in Parkinson’s disease? . J Parkinsons Dis 11: , 405–419. |
[89] | Oliveira de Carvalho A , Filho A , Murillo-Rodriguez E , Rocha NB , Carta MG , Machado S ((2018) ) Physical exercise for Parkinson’s disease: Clinical and experimental evidence. Clin Pract Epidemiol Ment Health 14: , 89–98. |