The Accuracy of Imaging Guided Targeting with Microelectrode Recoding in Subthalamic Nucleus for Parkinson’s Disease: A Single-Center Experience
Abstract
Background:
Accurate electrode targeting was essential for the efficacy of deep brain stimulation (DBS). There is ongoing debate about the necessary of microelectrode recording (MER) in subthalamic nucleus (STN)-DBS surgery for accurate targeting.
Objective:
This study aimed to analyze the accuracy of imaging-guided awake DBS with MER in STN for Parkinson’s disease in a single center.
Methods:
The authors performed a retrospective analysis of 161 Parkinson’s disease patients undergoing STN-DBS at our center from March 2013 to June 2021. The implantation was performed by preoperative magnetic resonance imaging (MRI)-based direct targeting with intraoperative MER and macrostimulation testing. 285 electrode tracks with preoperative and postoperative coordinates were included to calculate the placement error in STN targeting.
Results:
85.9% of electrodes guided by preoperative MRI were implanted without intraoperative adjustment. 31 (10.2%) and 12 (3.9%) electrodes underwent intraoperative adjustment due to MER and intraoperative testing, respectively. We found 86.2% (245/285) of electrodes with trajectory error ≤2 mm. The MER physiological signals length < 4 mm and ≥4 mm group showed trajectory error > 2 mm in 38.0% and 8.8% of electrodes, respectively. Compared to non-adjustment electrodes, the final positioning of MER-adjusted electrodes deviated from the center of STN.
Conclusion:
The preoperative MRI guided STN targeting results in approximately 14% cases that require electrode repositioning. MER physiological signals length < 4 mm at first penetration implied deviation off planned target. MER combined with intraoperative awake testing served to rescue such deviation based on MRI alone.
INTRODUCTION
Subthalamic nucleus (STN) deep brain stimulation (DBS) is a well-established neuromodulation treatment for Parkinson’s disease (PD) worldwide. So far, there are several electrodes implantation methods, including preoperative imaging-guided, microelectrode recordings (MER) assisted with intraoperative test, and intraoperative computerized tomography (CT) or magnetic resonance imaging (MRI)-verified approach. MER in DBS surgery is recognized as an efficient method for targeting STN region in previous studies [1, 2]. In recent decades, it has been proposed that the MER is not mandatory for STN-DBS as imaging-guided DBS surgery experienced significant growth [3, 4]. Imaging-guided targeting has also sparked the possibility of performing DBS during asleep procedure [5]. Considering the level of stress and discomfort, PD patients prefer asleep over awake surgery [6]. However, intraoperative CT or MRI facilities to confirm electrode position are not widely available, especially in developing countries. Therefore, it is critical to obtain an estimate rate of success of STN targeting by preoperative-MRI alone.
The targeting accuracy is highly associated with the outcome of STN-DBS for symptomatic improvement [7]. Different implantation methods from various medical centers have reported different level of targeting accuracy [3, 4, 8–10]. Some factors are known to have certain influence on targeting accuracy, such as stereotactic system used [11], target [12], and brain shift due to cerebrospinal fluid loss and intracranial air [13]. Here we propose that MER can serve as a predictive factor for targeting accuracy during STN-DBS.
In this study, the authors retrospectively analyzed single-center data of patients undergoing awake STN-DBS. The authors investigated the rate of success of implantation based on preoperative-imaging alone, and the targeting accuracy by preoperative-imaging alone vs. MER-guided procedures.
MATERIALS AND METHODS
Patients
We retrospectively analyzed 161 PD patients undergoing STN-DBS at our center from March 2013 to June 2021. Seven patients were excluded for anesthetic influence on MER. Three patients had unilateral implantation and 151 patients had bilateral implantation. MER was recorded in 305 hemispheres (153 left hemispheres, and 152 right hemispheres). The postoperative-electrodes coordinates were available in 285 hemispheres (Fig. 1). The study was conducted with the approval by the ethics committee of Zhejiang University School of Medicine Second Affiliated Hospital, approval number 2021-0925.
Fig. 1
The patients screening procedure. PD, Parkinson’s disease; MER, microelectrodes recording; STN, subthalamic nucleus.
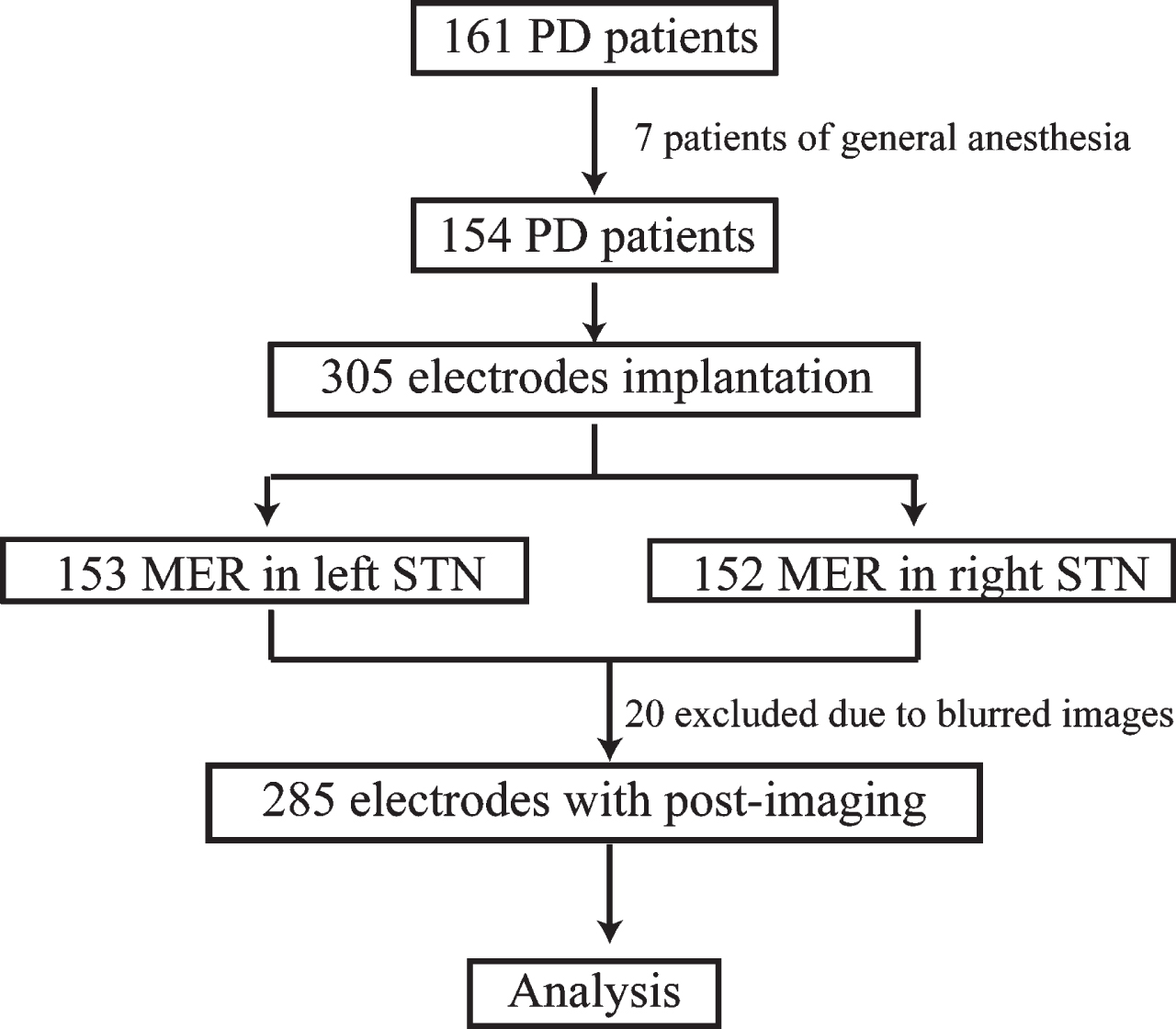
Preparation and target formulation
The implantation was planned by preoperative MRI-based (contrast enhanced 1.5T T1-weight MRI, and 3.0 T T2-weight axial MRI sequence) direct targeting. The patients were installed with Leksell frame under local anesthesia and performed three-dimensional CT scan subsequently. The images were ported to workstation (StealthStation, Medtronic or SinoPlan, Sinovation) for target planning. The neurosurgeon (ZZ) planned STN target trajectories via direct visualization at the maximum diameter slice of the red nucleus relative to the anterior-posterior commissures (AC-PC) line as previously described [14].
Surgery and electrode adjustment
Surgery was performed under local anesthesia. If bilateral implantation was performed, we first operated on the hemisphere contralateral to the side with more severe symptoms. Burr holes of 1.4 cm were placed by an arc incision through two ways: 1) simultaneous bilateral; 2) staged bilateral. Single-track microelectrodes were inserted through guide tube in a planned trajectory for recording electrophysiological signals. The recordings started from 10 mm above planned target with a microdrive of 0.5 mm steps until substantia nigra activity appeared, or until STN activity disappeared [15]. Trajectories with STN MER physiological signals length ≥4 mm were considered ideal [16]; otherwise, microelectrode was adjusted to a new trajectory and repeated recording until MER physiological signals length ≥4 mm were achieved (maximum 5 penetrations). The final placement of DBS electrodes was validated by intraoperative test for acceptable threshold for adverse effects with satisfactory improvement of symptom. The patients then underwent general anesthesia for implanting extension cables and implantable pulse generator.
Postoperative electrode localization
Permanent electrodes (four contacts with 1.5 mm length, 0.5 mm interval, Medtronic model 3389 or Pins model L301S) were used in this study. Postoperative CT images were obtained and imported to workstation (SinoPlan, Sinovation) to fuse preoperative MRI to visualize the final placement and coordinates in stereotactic space. The final placement coordinates were defined by identifying the center of the artifact perpendicular to planned target [17]. The trajectory error was defined as the perpendicular deviation from the final electrode to the planned trajectory. Postoperative-CT was obtained during postoperative one week. The electrode positions in STN region were defined as central, medial margin, medial outside, lateral margin, and lateral outside at the plane 4 mm below the AC-PC line (Fig. 2).
Fig. 2
The example of classification in postoperative electrodes position in STN region. A) Central in bilateral STN. B) Lateral margin in bilateral STN. C) Medial outside in right STN. D) Medial margin in right STN and central in left STN.
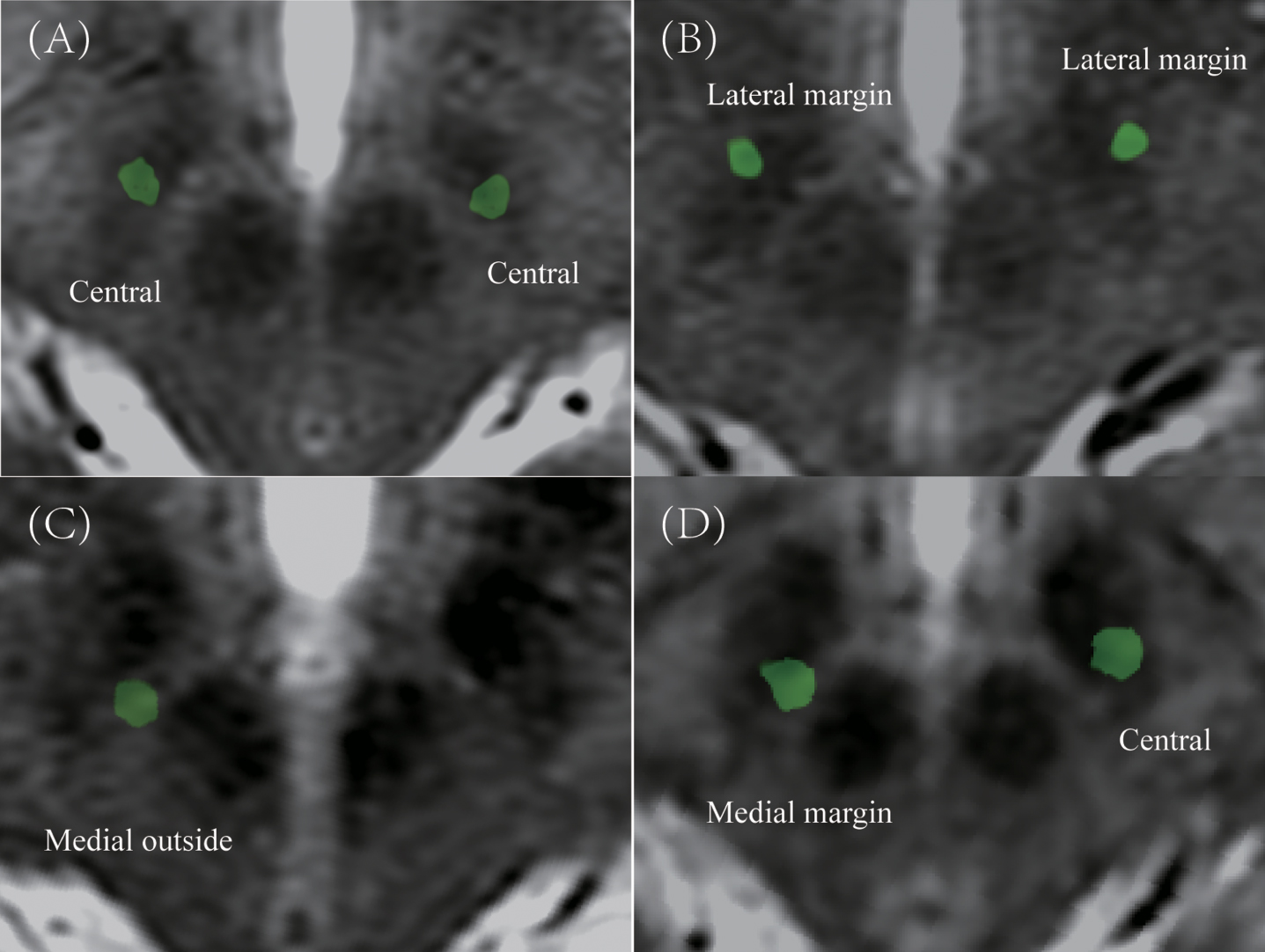
Statistical analysis
Categorical variables were tested by two tails chi-square test. Two tails unpaired t-test with Welch’s correlation was used for comparing trajectory error of MER physiological signals length ≥4 mm and < 4 mm group. The chi-square test with Bonferroni correction was used for comparing STN subregion position of MER adjusted and non-adjusted electrodes. p < 0.05 was considered statistically significance. Statistical analysis was performed in Statistical Product and Service Solutions (SPSS) version 22.0.
RESULTS
The demographics of patients are shown in Table 1. In total, 154 patients (76 male and 78 female) were included in our study, with a mean age of 62.6±7.6 (mean±SD).
Table 1
Demographics of patients
Characteristics | Value±SD |
No. Patients | 155 |
Age (y) | 62.6±7.6 |
Gender (Male/Female) | 76/78 |
Duration of symptoms (y) | 9.5±3.3 |
L-dopa equivalents dose | 891.2±343.7 |
UPDRS III-off | 55.2±16.0 |
UPDRS III-on | 28.2±11.0 |
UPDRS, Unified Parkinson’s Disease Rating Scale; SD, standard deviation.
85.9% (262/305) of electrodes were implanted based on preoperative-imaging alone without adjustment. 11.8% (18/153) of the electrodes in the left hemisphere and 16.4% (25/152) in the right hemisphere were adjusted intraoperatively. This result showed that imaging-guided alone was insufficient, as approximately 14% of electrodes needed repositioning.
Moving to intraoperative MER data, 15.0% (23/153) and 18.4% (28/152) of electrodes in the left and right hemispheres showed MER physiological signals length < 4 mm at first penetration, respectively. Of the 51 electrodes that required a second MER trajectory, 51.0% (26/51) required only one additional trajectory to identify the physiologically optimal target location, while 49% (25/51) required more than one additional penetration. The adjustment directions of microelectrodes were mostly medial-posterior and lateral-anterior (Fig. 3). We were unable to obtain MER criteria in 3.9% (12/305) of electrodes despite multiple repositioning. Inversely, 2.3% (7/305) of electrodes showed satisfactory threshold testing results, despite suboptimal MER findings.
Fig. 3
The intraoperative microelectrodes adjustment direction.
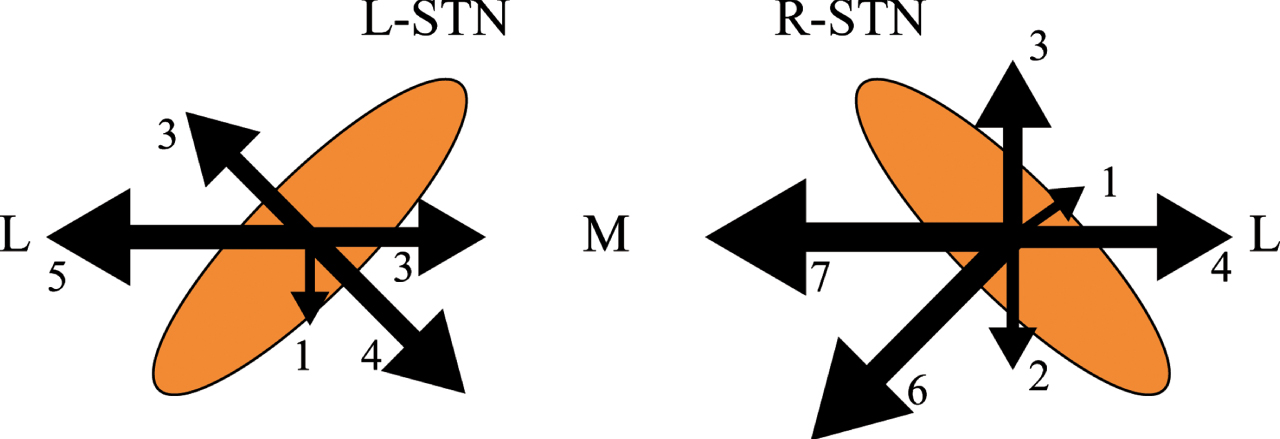
The targeting error in the planned vs. final placement of electrodes verified by postoperative images were shown in Table 2. The mean trajectory error was 1.22±0.06 mm (mean±SEM) and 1.26±0.06 mm (mean±SEM) in the left and right hemispheres, respectively, with no significant difference in x, y, and z axial. 86.0% (245/285) of electrode trajectory errors were ≤2.0 mm (Fig. 4), while 12.4% and 14.3% of electrodes trajectory error were > 2 mm in the left and right hemispheres, respectively. Neither laterality nor timing of second electrodes implantation (simultaneous vs. staged) showed significant influence on trajectory error.
Table 2
The pre- and post-electrodes coordinate in stereotactic space and deviation
Left side (mm) | Right side (mm) | |||||
x | y | z | x | y | z | |
Pre-op±SEM | 111.3±0.20 | 95.0±0.42 | 113.1±0.79 | 88.1±0.19 | 95.1±0.41 | 113.2±0.79 |
Post-op±SEM | 111.0±0.20 | 94.8±0.40 | 113.0±0.79 | 88.0±0.64 | 94.9±0.76 | 113.2±1.11 |
Vector error±SEM | –0.3±0.07 | –0.1±0.08 | –0.1±0.04 | –0.1±0.07 | –0.2±0.08 | 0.0±0.05 |
Absolute vector error±SEM | 0.7±0.05 | 0.7±0.05 | 0.4±0.03 | 0.7±0.04 | 0.8±0.05 | 0.5±0.03 |
Trajectory error±SEM | 1.22±0.06 | 1.26±0.06 |
SEM, standard error of mean.
Fig. 4
The deviation of final electrodes position compared with the planned target (0,0,0). The red dots represent trajectory error≤2 mm electrodes and the blue dot represent trajectory error > 2 mm electrodes. A, anterior; D, dorsal; L, lateral; M, medial; P, posterior; V, ventral.
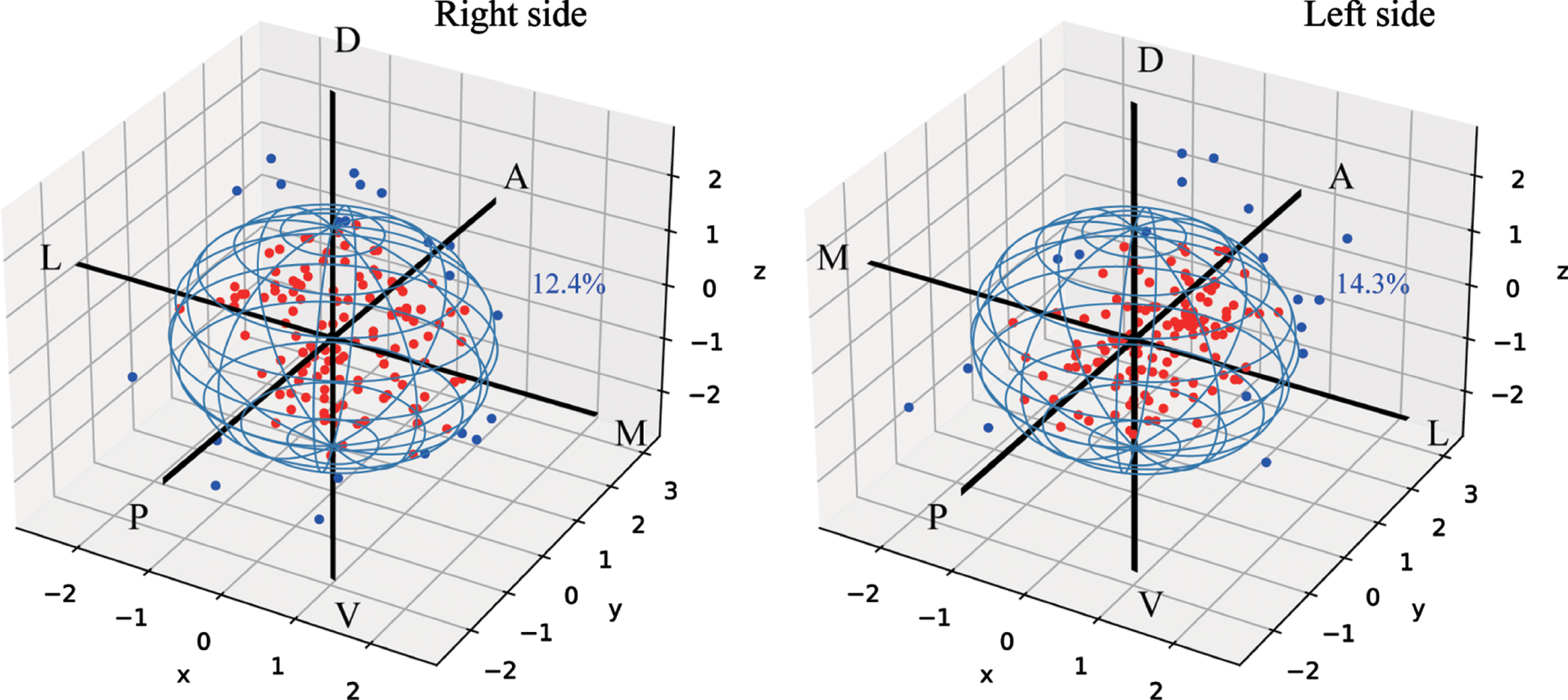
Trajectory error in groups with MER physiological signals length ≥4 mm and < 4 mm at first penetration were compared (Fig. 5). The MER physiological signals length ≥4 mm group showed a significantly smaller trajectory error vs. the MER physiological signals length < 4 mm group (1.15 mm vs. 1.67 mm, p < 0.01). Further, the trajectory error (percentage of leads with > 2 mm targeting error) was compared in above groups. 38.0% (19/50) of electrodes with MER physiological signals length < 4 mm show trajectory error > 2 mm, while 8.8% (21/238) of electrodes with MER physiological signals length ≥4 mm show trajectory error > 2 mm (χ2 = 29.59, p < 0.001).
Fig. 5
The histogram represents trajectory error difference between MER physiological signals length ≥4 mm and < 4 mm groups at first penetration. **p < 0.01.
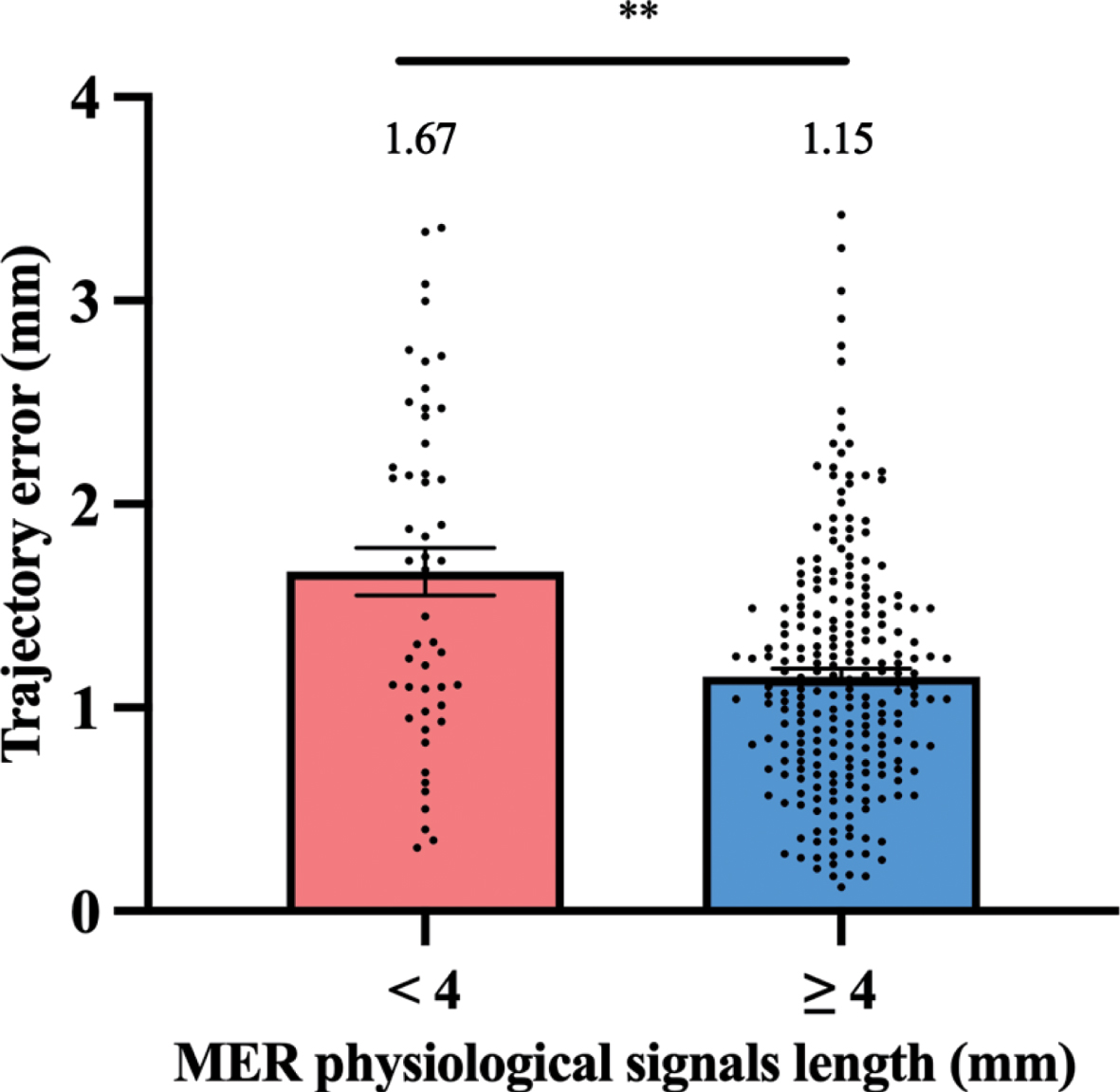
Finally, we verified final electrode positions in non-adjusted and MER-based adjusted trajectory in Table 3. Our results showed that electrodes that were adjusted intraoperatively had significantly greater level of deviation compared to electrodes without intraoperative adjustment (χ2 = 34.8, p < 0.001).
Table 3
The adjusted and non-adjusted electrode position in postoperative STN region
Central | Medial | Medial | Lateral | Lateral | Total | χ2 | p | |
margin | outside | margin | outside | |||||
Non-adjusted electrodes | 179 (71.9%) | 57 (22.9%) | 5 (2.0%) | 8 (3.2%) | 0 (0%) | 249 | 34.8 | < 0.001 |
Adjusted electrodes | 13 (36.1%) | 12 (33.3%) | 7 (19.4%) | 4 (11.1%) | 0 (0%) | 36 | ||
Total | 192 (67.4%) | 69 (24.2%) | 12 (4.2%) | 12 (4.2%) | 0 (0%) | 285 |
DISCUSSION
In this study, we aim to evaluate the success rate of preoperative imaging-guided alone STN-DBS surgery. The results show that 85.9% of electrodes are implanted without intraoperative adjustment, while 14.1% of electrodes need repositioning. These results are slightly better than previous study by Lozano et al. and Montgomery et al. [8, 18], as they reported that 70–80% of the electrodes were in good position using imaging-guided alone method. Our results reiterate the validation that preoperative imaging-guided alone was not sufficient for optimal electrodes placement during DBS procedure.
The electrode implantation accuracy assisted by MER in our study is similar to previous studies [7, 9, 10, 17]. Some studies propose smaller trajectory error using intraoperative MRI/CT-guided technique compared to MER [10, 17]. One possible reason is the multi-penetration in MER caused loss of cerebrospinal fluid and generation of intracranial air, while intraoperative MRI/CT-guided method usually only required one penetration. Similar finding is reported when dural sealant system prevented brain shift and improved targeting accuracy [19]. This may explain the result in our study that MER physiological signals length < 4 mm group show greater trajectory error, as multiple penetrations are done in this group. In our study, we choose 2 mm error as cut-off to represent optimal electrode accuracy based on volume of tissue activation model [20, 21], which has also been used as re-implantation breakpoints in previous clinical studies [22]. Our results also show that the electrode adjustment due to MER criteria predict deviation off central STN region. We find that neither laterality nor timing of second electrode implantation had significant impact on targeting accuracy, which is consistent with another previous study [23].
There is ongoing debate about MER’s necessity in STN-DBS surgery [24, 25]. Limousin et al. [4] performed preoperative MRI-guided and intraoperative MRI-verified approach without MER, and showed satisfactory UPDRS III scores improvement in 5 to 8 years. Similar findings were also reported in other studies [9, 10, 26]. MER may increase the risk of surgical complication, such as intracranial hemorrhage, compared to DBS surgery without MER [27]. However, in both awake and asleep STN-DBS surgery, the MER could be used to map out boundaries of the STN [28–30]. In our center, we find that MER provides useful targeting information intraoperatively. 31 (10.2%) of electrodes are repositioned to an optimal placement after intraoperative MER and symptom evaluation, which rescue suboptimal targeting at first penetration. However, whether MER is necessary for STN-DBS remains controversial. Different perspectives, such as outcomes, complication risks, side effects, and cost should be also be taken into account.
There are several limitations in our study. First, we do not include UPDRS III improvement outcome, and the optimal electrodes placement is based on intraoperative tests. There is no lead location correlation with therapeutic benefit to verify the very objective of the study. Second, the electrode position is obtained postoperative one week after surgery, during which brain shift remains a potential factor to error of actual location. Postoperative imaging performed at one month after surgery may be more reliable. Also, we do not consider the intracranial pneumocephalus in our study, which is suggested as a critical factor in stereotactic accuracy [31].
CONCLUSIONS
Our study concludes that preoperative imaging-guided alone STN targeting resulted in approximately 14% of electrodes that require intraoperative repositioning. Cases with intraoperative MER physiological signals < 4 mm at first penetration showed worse targeting accuracy, which can be rescued by MER and intraoperative testing.
ACKNOWLEDGMENTS
This work was supported by the National Natural Science Foundation of China (81301181) to Zhe Zheng and Zhejiang Key Research and Development Project (2019C03033) to Junming Zhu.
CONFLICT OF INTEREST
The authors declared no conflicts of interest.
REFERENCES
[1] | Seifried C , Weise L , Hartmann R , Gasser T , Baudrexel S , Szelenyi A , van de Loo S , Steinmetz H , Seifert V , Roeper J , Hilker R ((2012) ) Intraoperative microelectrode recording for the delineation of subthalamic nucleus topography in Parkinson’s disease. Brain Stimul 5: , 378–387. |
[2] | Koirala N , Serrano L , Paschen S , Falk D , Anwar AR , Kuravi P , Deuschl G , Groppa S , Muthuraman M ((2020) ) Mapping of subthalamic nucleus using microelectrode recordings during deep brain stimulation. Sci Rep 10: , 19241. |
[3] | Foltynie T , Zrinzo L , Martinez-Torres I , Tripoliti E , Petersen E , Holl E , Aviles-Olmos I , Jahanshahi M , Hariz M , Limousin P ((2011) ) MRI-guided STN DBS in Parkinson’s disease without microelectrode recording: Efficacy and safety. J Neurol Neurosurg Psychiatry 82: , 358–363. |
[4] | Aviles-Olmos I , Kefalopoulou Z , Tripoliti E , Candelario J , Akram H , Martinez-Torres I , Jahanshahi M , Foltynie T , Hariz M , Zrinzo L , Limousin P ((2014) ) Long-term outcome of subthalamic nucleus deep brain stimulation for Parkinson’s disease using an MRI-guided and MRI-verified approach. J Neurol Neurosurg Psychiatry 85: , 1419–1425. |
[5] | Park HR , Lim YH , Song EJ , Lee JM , Park K , Park KH , Lee WW , Kim HJ , Jeon B , Paek SH ((2020) ) Bilateral subthalamic nucleus deep brain stimulation under general anesthesia: Literature review and single center experience. J Clin Med 9: , 3044. |
[6] | LaHue SC , Ostrem JL , Galifianakis NB , San Luciano M , Ziman N , Wang S , Racine CA , Starr PA , Larson PS , Katz M ((2017) ) Parkinson’s disease patient preference and experience with various methods of DBS lead placement. Parkinsonism Relat Disord 41: , 25–30. |
[7] | McClelland S 3rd , Ford B , Senatus PB , Winfield LM , Du YE , Pullman SL , Yu Q , Frucht SJ , McKhann GM 2nd , Goodman RR ((2005) ) Subthalamic stimulation for Parkinson disease: Determination of electrode location necessary for clinical efficacy. Neurosurg Focus 19: , E12. |
[8] | Lozano CS , Ranjan M , Boutet A , Xu DS , Kucharczyk W , Fasano A , Lozano AM ((2019) ) Imaging alone versus microelectrode recording-guided targeting of the STN in patients with Parkinson’s disease. J Neurosurg 130: , 1847–1852. |
[9] | Liu X , Zhang J , Fu K , Gong R , Chen J , Zhang J ((2017) ) Microelectrode recording-guided versus intraoperative magnetic resonance imaging-guided subthalamic nucleus deep brain stimulation surgery for Parkinson disease: A 1-year follow-up study. World Neurosurg 107: , 900–905. |
[10] | Lee PS , Weiner GM , Corson D , Kappel J , Chang YF , Suski VR , Berman SB , Homayoun H , Van Laar AD , Crammond DJ , Richardson RM ((2018) ) Outcomes of interventional-MRI versus microelectrode recording-guided subthalamic deep brain stimulation. Front Neurol 9: , 241. |
[11] | Li Z , Zhang JG , Ye Y , Li X ((2016) ) Review on factors affecting targeting accuracy of deep brain stimulation electrode implantation between 2001 and 2015. Stereotact Funct Neurosurg 94: , 351–362. |
[12] | Ko AL , Ibrahim A , Magown P , Macallum R , Burchiel KJ ((2017) ) Factors affecting stereotactic accuracy in image-guided deep brain stimulator electrode placement. Stereotact Funct Neurosurg 95: , 315–324. |
[13] | van den Munckhof P , Contarino MF , Bour LJ , Speelman JD , de Bie RM , Schuurman PR ((2010) ) Postoperative curving and upward displacement of deep brain stimulation electrodes caused by brain shift. Neurosurgery 67: , 49–53; discussion 53-44. |
[14] | Andrade-Souza YM , Schwalb JM , Hamani C , Eltahawy H , Hoque T , Saint-Cyr J , Lozano AM ((2005) ) Comparison of three methods of targeting the subthalamic nucleus for chronic stimulation in Parkinson’s disease. Neurosurgery 56: , 360–368; discussion 360-368. |
[15] | Contarino MF , Bour LJ , Bot M , van den Munckhof P , Speelman JD , Schuurman PR , de Bie RM ((2012) ) Tremor-specific neuronal oscillation pattern in dorsal subthalamic nucleus of parkinsonian patients. Brain Stimul 5: , 305–314. |
[16] | Gross RE , Krack P , Rodriguez-Oroz MC , Rezai AR , Benabid AL ((2006) ) Electrophysiological mapping for the implantation of deep brain stimulators for Parkinson’s disease and tremor.S. Mov Disord 21: (Suppl 14), 259–283. |
[17] | Burchiel KJ , McCartney S , Lee A , Raslan AM ((2013) ) Accuracy of deep brain stimulation electrode placement using intraoperative computed tomography without microelectrode recording. J Neurosurg 119: , 301–306. |
[18] | Montgomery EB Jr ((2012) ) Microelectrode targeting of the subthalamic nucleus for deep brain stimulation surgery. Mov Disord 27: , 1387–1391. |
[19] | Sasaki T , Agari T , Kuwahara K , Kin I , Okazaki M , Sasada S , Shinko A , Kameda M , Yasuhara T , Date I ((2018) ) Efficacy of dural sealant system for preventing brain shift and improving accuracy in deep brain stimulation surgery. Neurol Med Chir (Tokyo) 58: , 199–205. |
[20] | Kuncel AM , Cooper SE , Grill WM ((2008) ) A method to estimate the spatial extent of activation in thalamic deep brain stimulation. Clin Neurophysiol 119: , 2148–2158. |
[21] | Butson CR , Cooper SE , Henderson JM , McIntyre CC ((2007) ) Patient-specific analysis of the volume of tissue activated during deep brain stimulation. Neuroimage 34: , 661–670. |
[22] | Holl EM , Petersen EA , Foltynie T , Martinez-Torres I , Limousin P , Hariz MI , Zrinzo L ((2010) ) Improving targeting in image-guided frame-based deep brain stimulation. Neurosurgery 67: , 437–447. |
[23] | Peng S , Levine D , Ramirez-Zamora A , Chockalingam A , Feustel PJ , Durphy J , Hanspal E , Novak P , Pilitsis JG ((2017) ) A comparison of unilateral deep brain stimulation (DBS), simultaneous bilateral DBS, and staged bilateral DBS lead accuracies. Neuromodulation 20: , 478–483. |
[24] | Aziz TZ , Hariz M ((2017) ) To sleep or not to sleep during deep brain stimulation surgery for Parkinson disease? Neurology 89: , 1938–1939. |
[25] | Pastor J , Vega-Zelaya L ((2020) ) Can we put aside microelectrode recordings in deep brain stimulation surgery? Brain Sci 10: , 571. |
[26] | Holewijn RA , Verbaan D , van den Munckhof PM , Bot M , Geurtsen GJ , Dijk JM , Odekerken VJ , Beudel M , de Bie RMA , Schuurman PR ((2021) ) General anesthesia vs local anesthesia in microelectrode recording-guided deep-brain stimulation for Parkinson disease: The GALAXY randomized clinical trial. JAMA Neurol 78: , 1212–1219. |
[27] | Zrinzo L , Foltynie T , Limousin P , Hariz MI ((2012) ) Reducing hemorrhagic complications in functional neurosurgery: A large case series and systematic literature review. J Neurosurg 116: , 84–94. |
[28] | Malinova V , Pinter A , Dragaescu C , Rohde V , Trenkwalder C , Sixel-Doring F , von Eckardstein KL ((2020) ) The role of intraoperative microelectrode recording and stimulation in subthalamic lead placement for Parkinson’s disease. PLoS One 15: , e0241752. |
[29] | Morace R , De Angelis M , Aglialoro E , Maucione G , Cavallo L , Solari D , Modugno N , Santilli M , Esposito V , Aloj F ((2016) ) Sedation with α2 agonist dexmedetomidine during unilateral subthalamic nucleus deep brain stimulation: A preliminary report. World Neurosurg 89: , 320–328. |
[30] | Micieli R , Rios AL , Aguilar RP , Posada LF , Hutchison WD ((2017) ) Single-unit analysis of the human posterior hypothalamus and red nucleus during deep brain stimulation for aggressivity. J Neurosurg 126: , 1158–1164. |
[31] | Sharim J , Pezeshkian P , DeSalles A , Pouratian N ((2015) ) Effect of cranial window diameter during deep brain stimulation surgery on volume of pneumocephalus. Neuromodulation 18: , 574–578; discussion 578-579. |