Understanding Sleep Regulation in Normal and Pathological Conditions, and Why It Matters
Abstract
Sleep occupies a peculiar place in our lives and in science, being both eminently familiar and profoundly enigmatic. Historically, philosophers, scientists and artists questioned the meaning and purpose of sleep. If Shakespeare’s verses from MacBeth depicting “Sleep that soothes away all our worries” and “relieves the weary laborer and heals hurt minds” perfectly epitomize the alleviating benefits of sleep, it is only during the last two decades that the growing understanding of the sophisticated sleep regulatory mechanisms allows us to glimpse putative biological functions of sleep. Sleep control brings into play various brain-wide processes occurring at the molecular, cellular, circuit, and system levels, some of them overlapping with a number of disease-signaling pathways. Pathogenic processes, including mood disorders (e.g., major depression) and neurodegenerative illnesses such Huntington’s or Alzheimer’s diseases, can therefore affect sleep-modulating networks which disrupt the sleep-wake architecture, whereas sleep disturbances may also trigger various brain disorders. In this review, we describe the mechanisms underlying sleep regulation and the main hypotheses drawn about its functions. Comprehending sleep physiological orchestration and functions could ultimately help deliver better treatments for people living with neurodegenerative diseases.
INTRODUCTION
Sleeping, like eating or breathing, is inherent and vital to human existence. It is commonly said that we spend one third of our life asleep. An abundant body of literature describes how important sleep is for both our mental and physical health [1–3]. Yet, sleep remains enigmatic in its regulation, and even more puzzling in its functions. The understanding of sleep biology and raison d’être has nevertheless made tremendous progress since the beginning of sleep science almost 100 years ago [4, 5]. From that moment onwards, the vision of sleep as an inactive state progressively gave way to the recognition of a period of intense brain activity involving intricate cellular and molecular processes that give rise to complex biological and behavioral phenomena.
Sleep, or a state resembling sleep, has been reported in almost all species. However, sleep might not entail similar physiological processes nor functions in all animals [6,7]. In mammals and birds, sleep is composed of two distinct global activity states that alternate cyclically: non-rapid eye movement sleep (NREMS) generally followed by rapid eye movement sleep (REMS). In other species including fish and reptiles, two sleep stages are also observed, but they cannot be unambiguously identified as NREMS and REMS [7]. Despite being a highly conserved feature among different phyla, the origin and necessity of two distinct sleep states remains to be elucidated [7]. Typically, in mammals and birds, the two sleep stages are characterized by polysomnographic measures including the scalp electrical activity (electroencephalography; EEG), muscular activity (electromyography; EMG), as well as eye movements (electrooculography). In species where EEG/EMG recording is impractical, such as flies or worms, sleep is monitored using behavioral criteria. The neural signature of sleep in zebrafish can be monitored through fluorescence-based polysomnography using a calcium indicator [8]. Human sleep is monophasic, occurring in a single block lasting between four to eight hours, and typically consists of three to five cycles of NREMS-REMS stages, whereas for other mammals, like rodents, sleep is polyphasic and distributed over 24 hours, with more fragmented cycles [9,10]. NREMS, REMS, and wakefulness exhibit specific electrophysiological, physiological, and behavioral features that are explored in dedicated sections later in this review.
Sleep architecture can vary according to endogenous biological factors, such as age or sex, and environmental factors, such as work, culture, and lifestyle [1]. For instance, NREMS tends to decrease with age in humans [11], whereas the opposite is usually observed in rodents [12]. For both humans and rodents, males and females display slightly different NREMS and REMS profiles [13, 14]. Moreover, in humans, sleep is socially and societally driven [1], and many external parameters can influence sleep-wake patterns, including working time (e.g., shift-work) [2], cultural sleep habits [15], and screen time [16]. The impact these exogenous factors have on sleep is not trivial. According to the Whitehall longitudinal study of about 8,000 British civil servants, less than 7 hours of sleep per night over 25 years is associated with poor health outcomes, including a 30% increased risk of developing dementia [17]. Similarly, in the Million Women Study with over 800,000 women followed since 2001 for their sleep habits, longer sleep (greater than 8 hours per night) or daytime napping was not correlated with greater dementia risk, but short sleep was (less than 7 hours per night), although this increased risk was rather small [18]. In both studies, the sleep is mostly self-reported rather than measured by EEG, and the consequences for dementia risk of poor sleep continuity (e.g., sleep-wake fragmentation) are not taken into account. Indeed, insomnia (fragmented sleep) could produce worse health outcomes [19], but this is more difficult to study in a large-scale longitudinal design.
The detailed structure of sleep-wake orchestration has for long been unclear, but recent technological advancements have allowed the study of sleep by untangling the Gordian knot instead of cutting it. Indeed, the monitoring of specific neuronal ensembles using calcium imaging, or their manipulation through optogenetics and chemogenetics, have facilitated the dissection of the neural networks that cooperate to generate sleep and wakefulness. In this review, we aim at highlighting the importance of understanding how and why we sleep, especially in pathological contexts, by looking at the mechanisms underlying sleep-wake regulation, and by delineating the suggested functions of sleep.
THE FUNCTION(S) OF SLEEP: AN EVOLVING PROBLEM
Sleep has been observed, described, and studied since Antiquity [5]. Nonetheless, although progress has been made toward understanding the biological mechanisms underlying how we sleep, the reason why we sleep, i.e., its most fundamental function(s), remain poorly understood [6, 20]. From the perspective of species survival, there is an obvious advantage of being awake instead of asleep, engaging in foraging, reproduction, avoiding predation, and protecting offspring. Therefore, the benefits of sleep must outweigh its evolutionary costs. Moreover, extended periods of sleep loss are detrimental for the organism of animals and humans and could even lead to death [21–23].
Sleep, or at least rest, is often defined as ubiquitous since it has been reported in one form or another in all animal species studied, although some animals seem to sleep rather little. Northern elephant seals sleep only 2 hours per day while they are at sea for seven months [24]. Moreover, some migrating birds can postpone sleep with apparently no consequence [6]. Indeed, the phylogenetic comparative studies of sleep show that not all animals sleep the same way, so that the function of sleep (or rest) might serve different purposes in different types of animals (e.g., vertebrates and invertebrates, warm-blooded and non-warm blooded) [6]. Furthermore, for mammals and birds, it is unclear why there is a need to be unconscious when sleeping, so whatever physiological purpose(s) sleep serves, consciousness is incompatible with it [20]. Here, we summarize the main hypotheses that have been proposed in respect to the functions of mammalian sleep. Surprisingly, and in spite of intensive study, researchers have not yet reached a consensus on the function of sleep, or even if it is primarily for the body (non-neural tissue) or the brain.
The failure to reach a consensus, so far, about the function of sleep is probably because whatever the function of sleep is (assuming it has one), the consequences of not getting enough of this function likely impacts many other processes indirectly. For instance, if memory is poorer after sleep deprivation, this does not necessarily mean that one of the functions of sleep is to help form memories, but could instead mean that memory formation requires some aspect of general brain circuitry that is “serviced”, for want of a better word, during sleep [20]. Although there is a view that sleep is a purely adaptive behavior dependent on the type of animal (e.g., a top predator, such as a lion, sleeps because there is little else to do when not hunting) [6, 25], the cardinal importance of sleep for physiological function(s) is a broadly accepted hypothesis among most neuroscientists. We also know from our own (human) experience that after a night of less sleep, or no sleep, we feel nonoptimal, and if sleep deprivation is extreme, we are forced to sleep even against our will. Such introspective observations underline that the need to sleep is a primal drive, like hunger and thirst, suggesting sleep’s purpose, at least for humans, is essential.
Sleep has classically been seen as a somewhat inactive but efficient state that was ultimately useful for suppressing behavioral activity when wakefulness is not advantageous, and by reducing energy expenditure, sharing energy-saving functions with torpor [25]. It has been suggested that rather than just energy conservation, sleep could also underlie molecular mechanisms that reallocate energy for restorative anabolic processes [26, 27]. This could be facilitated by the decrease of brain and core body temperature during sleep [20, 28, 29].
Beyond the idea of adaptative energy preservation, molecular and cellular regenerative functions of sleep have been proposed. Sleep is associated with an increase in the expression of genes required for biosynthesis and transport, suggesting the restoration of vital cellular components in preparation for subsequent wakefulness [30]. Sleep could then facilitate growth, increase resistance to biochemical stressors, prevent DNA damage and support the immune system [31–34], as well as provide adaptative temporal compartmentalization of diverse cellular processes such as metabolic and biochemical reactions that would be less efficient if happening during wakefulness [35, 36]. REMS, in particular, correlates with sensorimotor circuit development through sensory feedback received after the generation of myoclonic twitches occurring exclusively during REMS [37]. Sleep might also facilitate the clearance of toxic molecules from the brain, including protein aggregates and metabolic waste products, through an increased flux of the interstitial fluid [38].
Sleep might also regulate higher brain functions such as synaptic plasticity, supporting learning processes and memory formation. During sleep, there is an increased expression of genes involved in neuroplasticity and protein synthesis [39–42]. Building new memories could involve the strengthening of specific existing synapses and the creation of new ones, a process that is thought to be happening during wakefulness, as well as the sleep-associated selective mechanism of removing weak synapses and keeping the strongest, called synaptic downscaling [43, 44]. The absence of sensory input during sleep may support the reorganization of neural circuits as memories consolidate [45]. Of note, REMS-associated hippocampal theta oscillations contribute to spatial and contextual memory consolidation [46, 47]. Sleep could also facilitate, at least in humans, novel insight and creativity by changing the representational structure of newly created memories [48]. Furthermore, sleep could be involved in recovering from emotional stress or processing emotionally stressful events, so reducing anxiety [49, 50]. Again, we emphasize that many of these suggested benefits of sleep may be indirect consequences of some other, more basic, essential process that happens during sleep.
THE SLEEP-WAKE CYCLE: TO SLEEP OR NOT TO SLEEP
Whether diurnal or nocturnal, most species exhibit a drive to sleep during a specific period of the day. Meanwhile, sleep is also prompted by the increasing amount of time spent awake. These two phenomena are the foundation of the two-process model [51]. The model postulates that the timing, duration, and intensity of sleep is orchestrated by two interacting processes: the circadian rhythm (process C) and sleep homeostasis (process S) (Fig. 1).
Fig. 1
The two-process model of sleep regulation. Simplified representation of the homeostatic process S (blue line) and circadian process C (brown lines) over a two-day period with 16 hours of wakefulness and 8 hours of sleep (grey bars). During the increase of process S, the individual is awake and the sleep pressure builds up. When process S reaches the upper threshold of process C, the individual falls asleep and process S decreases until it reaches the lower threshold of process C, which triggers the awakening of the individual.
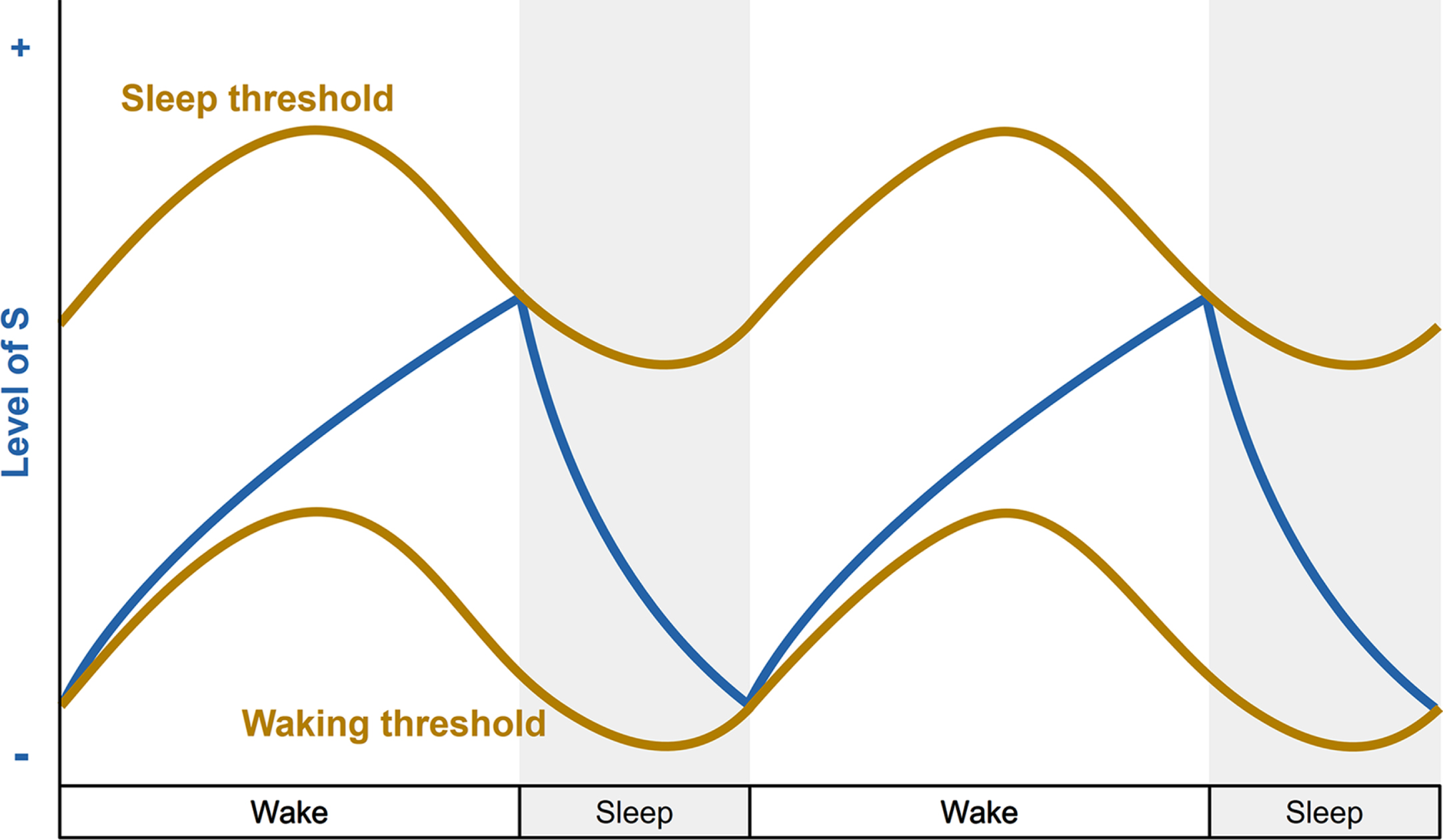
Circadian rhythms are internally generated cycles of approximately 24 hours that underlie essential body functions and processes. In mammals, circadian timekeeping is driven by a central pacemaker, the suprachiasmatic nucleus of the hypothalamus (SCN), which orchestrates the circadian rhythmicity of the physiological and behavioral aspects of the sleep-wake cycle [52]. The SCN exhibits endogenous circadian oscillations of gene expression, firing rate and GABA/peptide neurotransmitter release that constitute the gears of a molecular clockwork. This biological clock is synchronized by the daily predictable changes of environmental time cues (zeitgebers), such as light and ambient temperature, and in turn synchronizes the circadian molecular oscillators in all other neurons and cells. Harboring GABAergic and peptidergic (e.g., vasoactive intestinal polypeptide) neurons, the SCN is hypothesized to bidirectionally link to many sleep-wake regulatory regions of the brain, especially in the hypothalamus. Indeed, the anatomical connections between the SCN and neighboring hypothalamic nuclei are predicted to be a cardinal component of the daily regulation of sleep behavior; although curiously, the precise connections that specify how the SCN promotes sleep, for example, have still not yet been convincingly found [53]. It may be well that the SCN promotes wake, rather than sleep per se [54].
Sleep homeostasis, on the other hand, is proposed to balance the drive to sleep as a function of fatigue accumulation [51]. This drive eventually triggers physiological mechanisms that prepare the organism for sleep, leading to an increased difficulty of staying awake. The prolonging of wakefulness while homeostatic sleep pressure augments usually produces, once asleep, a compensatory “sleep rebound” or “recovery sleep” characterized by an increase of sleep duration and intensity. In mammals, this sleep drive translates into an increase of cortical slow wave activity (SWA; 0.5–4.5 Hz) whose intensity correlates with the need for sleep. SWA increases when sleep pressure is high and decreases subsequently during NREMS. SWA could also be related to the type of brain activity processes engaged while awake [55]. Nonetheless, the underlying biological mechanisms of sleep homeostasis are still poorly understood, but it has already been proposed by Legendre and Piéron in 1907 that an accumulating somnogenic substance in the brain during wakefulness could reflect sleep drive and promote sleep when that substance reaches a high concentration. Notably, the extracellular levels of adenosine in the basal forebrain (BF), an important brain area for sleep regulation, increase following extended wakefulness and decrease during recovery sleep [56, 57]. In addition, the antagonism of adenosine signaling prevents sleep rebound after sleep deprivation [58]. If adenosine seems a good candidate to underlie sleep drive, more research is needed to fully explain the physiological mechanisms behind sleep homeostasis. Many other sleep-promoting molecules could govern or influence sleep homeostasis [59], but none of these molecules, including adenosine, are essential for this phenomenon. Furthermore, the circuitry that regulates sleep homeostasis seems distributed throughout the mammalian brain [20], in contrast to the circadian system controlled primarily by one nucleus, the SCN. Additionally, the length of sleep in mammals can be influenced by genetic programs. Polymorphisms of the transcription factor DEC2, for instance, determines sleep length in humans [60], and activation of the liver kinase-salt-inducible kinase3 pathway, that then inhibits histone deacetylase 4, increases sleep time [61, 62]. So far, it is not known how these new transcriptional pathways fit into the process S and C concepts.
In summary, decades of research dedicated to the understanding of the neural circuitry that orchestrates the circadian and homeostatic regulation of the sleep-wake cycle have enabled the uncovering of a remarkably complex system involving many regions scattered throughout the brain. Wake- and sleep-promoting neuronal populations of these brain regions release, and are affected by, a wide range of neurotransmitters and neuromodulators that underlie sophisticated mechanisms of activations and inhibitions, ultimately giving rise to a stable sleep-wake behavior and architecture [63].
THE REGULATION OF WAKEFULNESS: STAYING AWAKE
It is usually easier to find a consensual definition of wakefulness than sleep. When awake, animals set into motion physiological and cognitive processes allowing the interaction with, and response to, its environment through voluntary motor activity that enables goal-directed behavior. Therefore, wakefulness is also associated with muscle tone and variable levels of brain activity, breathing and heart rate. Electrophysiologically, the waking state is characterized by high-frequency, low-amplitude cortical oscillations (desynchronized EEG), and sustained EMG activity [64, 65].
The first glimpse into the physiological mechanisms responsible for wakefulness and sleep regulation arose in the early 20th century. In 1917, von Economo reported the epidemic outbreak of a peculiar polioencephalitis, characterized by unusual neurological symptoms including hypersomnia, that he named “encephalitis lethargica” [4, 66]. In the brain of patients who succumbed from severe types of the disease, von Economo observed the pathological involvement of the posterior hypothalamus, and concluded that the latter must exert wake-promoting effect upon the brain. He also later identified the anterior hypothalamus as responsible for sleep initiation.
More than a century later, tremendous progress has been made in the depiction of the neurocircuitry responsible for the regulation of wakefulness (Fig. 2). It especially implicates cortical and thalamocortical stimulation arising from multiple excitatory and inhibitory neuronal populations throughout the brain, which also prevent sleep initiation by silencing sleep-promoting regions [64, 65]. The fast-acting neurotransmitters glutamate and GABA are essential for the promotion of wakefulness, while neuromodulators which include monoamines, acetylcholine and many neuropeptides seem to provide a fine-tuning role in wakefulness generation and persistence. Fundamentally, brainstem glutamatergic neurons in the parabrachial nucleus (PBN) and the pedunculopontine tegmental nucleus (PPT) form the foundation of the wake-promoting system through axonal projections to the BF and hypothalamic regions such as the lateral preoptic area (LPO) and lateral hypothalamus (LH) [64, 65]. Interestingly, the PBN is an important hub that integrates internal and external sensory inputs related to, for instance, temperature, pain, breathing, appetite, thirst, or heart rate, and may therefore support the adaptative regulation of arousal [67].
Fig. 2
Summary of the wake-promoting pathways in the rodent brain. Wakefulness is mainly generated by fast-acting glutamatergic projections from the brainstem (e.g., PBN, LDT, PPT) to the BF and hypothalamic nuclei (e.g., PAO, LH), as well as widespread-projecting monoaminergic neurons from the LC, DR, and vPAG. The BF promotes arousal through GABAergic, cholinergic, and glutamatergic projections to the thalamus, hypothalamus, and cortex. The LH generates and maintain wakefulness mainly through innervations of the thalamus, POA and TMN. Wake-modulating pathways from the hypothalamus and VTA are shown with dashed lines. BF, basal forebrain; DR, dorsal raphe; LC, locus coeruleus; LDT, laterodorsal tegmental nucleus; LH, lateral hypothalamus; PBN, parabrachial nucleus; POA, preoptic area of the hypothalamus; PPT, pedunculopontine tegmental nucleus; TMN, tuberomammillary nucleus of the hypothalamus; vPAG, ventral periaqueductal gray; VTA, ventral tegmental area; ZI, zona incerta. Created with BioRender.com.
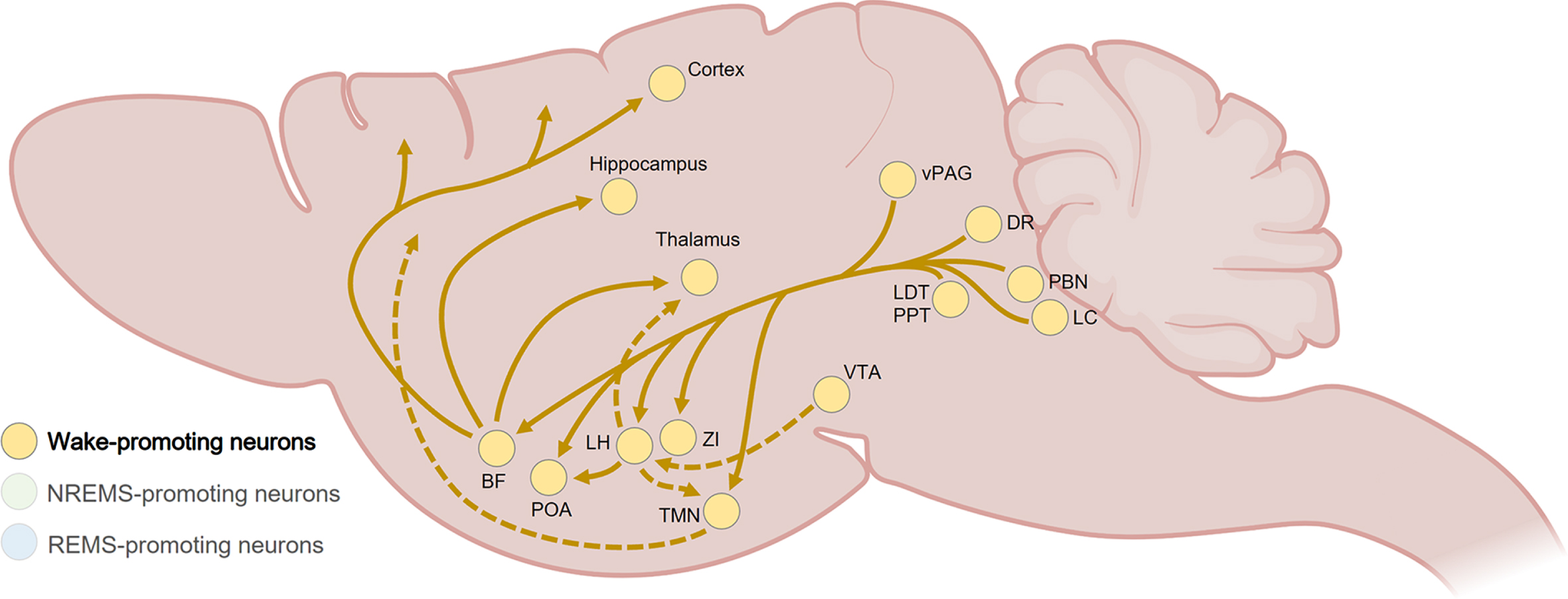
In the BF, GABAergic, cholinergic and glutamatergic neuronal populations then induce arousal through diffuse cortical projections [68–70], while GABA-expressing LH neurons also play a crucial role in generating and maintaining wakefulness via innervations of the brainstem, the thalamus, and the preoptic area of the hypothalamus (POA) [64, 65, 71]. Furthermore, glutamatergic/nitrergic neurons of the ventral tegmental area (VTA), located in the midbrain, are crucial for the generation of wakefulness through projections to the LH and the nucleus accumbens (NAc) [72].
The hypothalamus regulates wakefulness by releasing key wake-promoting neuromodulators. Among them, the hypocretin (also known as orexin) neuropeptide is released by neurons exclusively located in the LH that innervate wide areas of the brain, including wake-promoting neurons in the sublaterodorsal tegmental nucleus (SLD), hypothalamic tuberomammillary nucleus (TMN) and locus coeruleus (LC) [73, 74]. The selective loss of hypocretinergic neurons is associated with narcolepsy, characterized by excessive daytime sleepiness and REMS dysregulation [75]. Moreover, histaminergic neurons in the TMN are only active during wakefulness and are reciprocally connected to several arousal-related regulatory systems [76]. The stimulation of histamine neurons induces wakefulness while their specific deletion causes persistent sleep-wake fragmentation [77]. Cooperatively, several widespread-projecting monoaminergic neurons are wake-active and uphold the arousal system by supporting the transition from sleep to wake and/or the maintenance of wakefulness [64, 65], such as noradrenergic neurons in the LC [78, 79], dopaminergic neurons in the dorsal raphe (DR) and ventral periaqueductal gray (vPAG) [80, 81], and the serotoninergic neurons in the DR [82, 83], even if the latter can also induce sleep [84, 85].
THE REGULATION OF NON-REM SLEEP: LOST IN TRANSITION
Under enough sleep pressure, the brain of mammals and birds can transit from wakefulness to NREMS. This transitional phase of physiological appeasement and diminished alertness is thought to facilitate the emergence of NREMS through relevant pre-sleep behaviors and a gradual decrease of wake-promoting neuromodulators [86, 87]. NREMS is then characterized by a reduction of responsiveness to internal and external stimuli (higher arousal threshold), a decrease of core body and brain temperature, low muscle tone, and a slowing down and stabilization of breathing and heart rate [64, 65]. What distinguishes the quiescence of NREMS from anesthesia, hibernation, or coma, is its rapid reversibility to wakefulness providing stimuli exceed the arousal threshold. Mammals, including humans, display species-specific body posture (e.g., curling up) when preparing for sleep in a nest or bed, creating microclimates of skin warmth [29, 88]. On an electrophysiological level, NREMS episodes exhibit large amounts of high-amplitude slow wave oscillations in the delta (0.5–4.5 Hz) frequency ranges that propagate across the cortex. After a prolonged period of wakefulness, an increased amount of NREMS with high delta oscillations is generally observed (sleep rebound) [20]. In addition, NREMS is characterized by intermittent oscillatory events, such as sleep spindles (oscillatory burst events of 11 to 15 Hz lasting 0.5 to 1.5 s), k-complexes (delineated negative sharp waves followed by a positive component lasting ∼0.5 s), or infraslow oscillations (<0.1 Hz).
NREMS is generated by a scattered neural network, involving a broad range of neurotransmitters and neuromodulators, which inhibit and stimulate wake-promoting and sleep-enabling neuronal populations, respectively [64, 65] (Fig. 3). As for wakefulness, the hypothalamus is a core node of the sleep system, and in particular the highly heterogeneous POA which also controls sleep-associated features including thermoregulation and stress [89, 90]. Indeed, in 1996, Saper and colleagues described the first sleep-active group of neurons in the ventrolateral preoptic nucleus of the hypothalamus (VLPO). The key role of the POA in the initiation of sleep was later confirmed with the identification of NREMS-promoting GABAergic and galaninergic neurons in the VLPO, LPO and median preoptic hypothalamic region sending inhibitory projections to the main structures of the arousal system including the histaminergic TMN, the orexinergic LH, the dopaminergic VTA, the noradrenergic LC, the glutamatergic PBN, the serotoninergic DR, and the cholinergic BF and laterodorsal tegmental nucleus (LDT) [64, 65]. The LH also receives inhibitory terminals from GABA neurons in the VTA, participating in the induction of NREMS [50, 72].
Fig. 3
Summary of NREMS-promoting pathways in the rodent brain. The POA plays a key role in NREMS generation through GABAergic and galaninergic inhibitory projections to the wake-promoting LH, TMN, VTA, DR, LC, PBN, LDT, and SLD. Within the brainstem (dashed lines), the PFZ and vM participate to NREMS maintenance by inhibiting wake-promoting regions such as the VTA, LC, DR and PBN, while the activity of the vM is sustained by glutamatergic projections from the vPAG. The VTA also sends inhibitory GABAergic projections to the LH. The BF inhibits wake-promoting neurons throughout the cortex and is also involved in the generation of NREMS-associated delta waves and spindle oscillations by sending axonal terminals in the thalamus. BF, basal forebrain; DR, dorsal raphe; LC, locus coeruleus; LDT, laterodorsal tegmental nucleus; LH, lateral hypothalamus; PBN, parabrachial nucleus; POA, preoptic area of the hypothalamus; PPT, pedunculopontine tegmental nucleus; PFZ, parafacial zone; SLD, sublaterodorsal tegmental nucleus; TMN, tuberomammillary nucleus of the hypothalamus; vM, vental medulla; vPAG, ventral periaqueductal gray; VTA, ventral tegmental area. Created with BioRender.com.
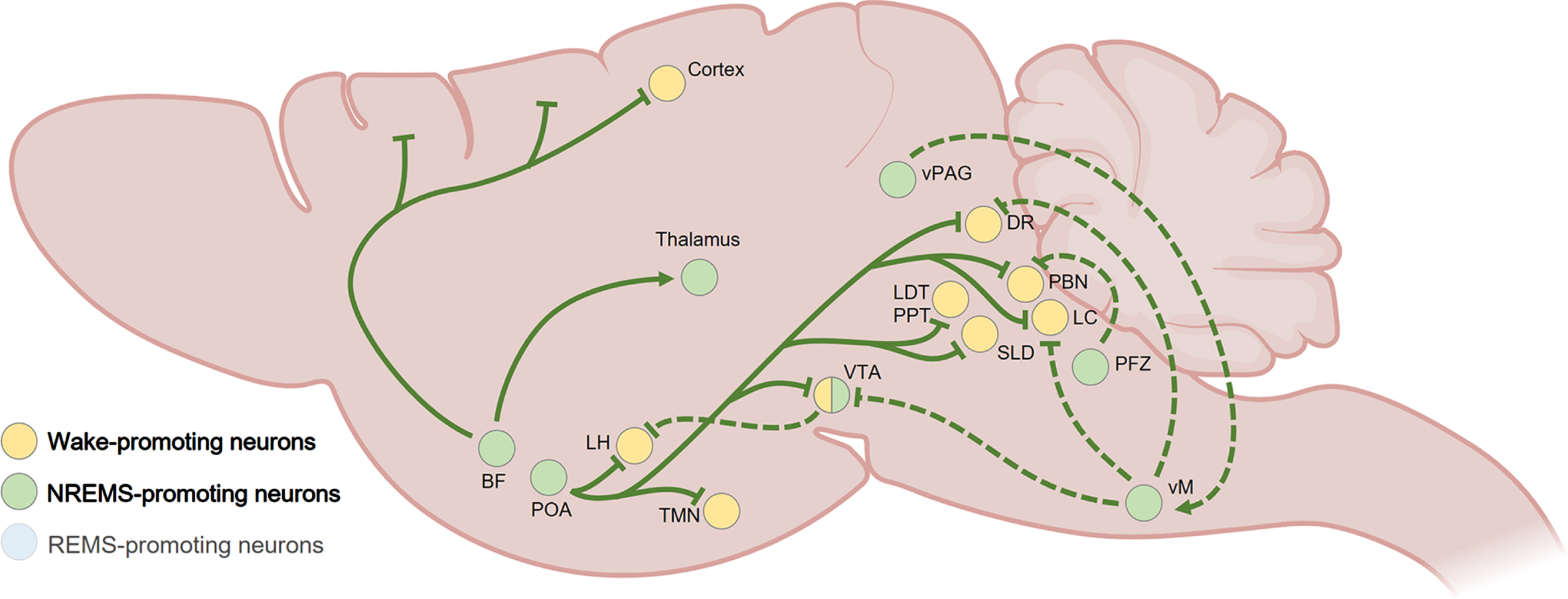
GABAergic subnuclei from the medulla also play a major role in NREMS generation by inhibiting wake-promoting neurons. For instance, the parafacial zone (PFZ) sends axonal terminals to the PBN [91], and ventromedial medulla (vmM) inhibits monoaminergic neuronal clusters in the LC, VTA, and DR [92]. GABA neuronal firing in the vmM can be sustained by glutamatergic/neurotensinergic projection from the vlPAG [92], while the activity of the POA and the PFZ can be supported through glutamate released by neuronal projections originating from the perioculomotor (pIII) midbrain nucleus [93].
Outside the hypothalamus and the brainstem, a constellation of other important NREMS-promoting neuronal populations, reciprocally connected to sleep-wake regulatory regions, have been identified. In the BF, a cluster of somatostatin-expressing GABA neurons potently inhibits wake-promoting BF neuronal subpopulations [70]. Several neuronal ensembles located in the thalamus, in particular GABAergic cells in the thalamic reticular nucleus, have been involved in the generation of NREMS-associated delta waves and spindle oscillations through cholinergic inputs from the BF and the brainstem [94, 95]. In addition, glutamatergic/neurotensinergic neurons in the posterior part of the thalamus can promote NREMS through activation of GABAergic cells in the amygdala [96].
The midbrain and basal ganglia contribute substantially to the generation of NREMS. One of the largest experimental effects on NREMS in mice occurs when GABA neurons in the VTA are selectively lesioned [72]. This produces uninterrupted wakefulness selectively during the dark (active) period, and artificially activating these GABA VTA cells induces sustained NREMS [72]. These cells induce sleep by local inhibition of dopamine neurons and by sending projections to the lateral hypothalamus [72]. A subtype of these VTA GABA cells, those expressing somatostatin, respond to social defeat stress in male mice to induce sleep [50]. Furthermore, GABAergic neurons in the substantia nigra pars reticulata were found to promote NREMS [97, 98]. The basal ganglia, through interconnections with regions responsible for motor control, cognition, and arousal, may be an important hub for sleep-wake regulation [99]. Indeed, adenosine inputs to the striatum, NAc, and olfactory tubercle contribute to NREMS generation [100–102].
There is also evidence for top-down control of sleep by the neocortex. Long-term genetic silencing of layer 5 pyramidal neurons and dentate granule cells in mice produces permanent and substantial increases in wakefulness selectively during the dark period [103], although the relevant targets for these cells for inhibiting wakefulness are not known.
How all these extremely diverse NREMS- and wake-promoting areas link up, involving most of the brain, is an unsolved problem (Figs. 2 and 3). There does not seem to be a simple main hub regulating sleep or wakefulness. If we could understand the function of sleep, then this may give insight into why this circuitry is so distributed.
THE REGULATION OF REM SLEEP: THE PARADOX
REMS was first discovered in 1953 when Kleitman and Aserinsky noticed in children’s sleep the occurrence of 10 to 20 min episodes of rapid, jerky eye movements regularly interspersed among longer phases without ocular movement [5, 7]. Later, Jouvet described in cats the recurrence of short “paradoxical” sleep episodes characterized by waking EEG but flat EMG, and called them “sommeil paradoxal” [5].
Despite accounting for only 20 to 25 percent of total sleeping time in healthy human adults, REMS is a sophisticated heterogenous cerebral state encompassing continuous (tonic) and discrete (phasic) behavioral and neurophysiological features [104]. Notably, the tonic components of REMS include fast EEG oscillations of low amplitude (theta rhythm between 6 and 9 Hz, and gamma rhythm around 50 Hz or higher), suppression of skeletal muscle tone (atonia), suspension of thermoregulation and elevation of brain temperature [64, 105]. The phasic REMS features especially include bursts of rapid eye movements linked to ponto-geniculo-occipital (PGO) wave forms (P-waves in rodents) that propagate between the pons, the lateral geniculate nucleus and the occipital cortex, but also include myoclonic twitches of skeletal muscles as well as increase and irregularities in breathing and heart rate [64, 105]. REMS is characterized by low arousal, but high awareness compared to NREMS [106]. In addition, REMS is associated with vivid dreams emanating from the activity of cortical regions that are normally silent during sleep [107].
REMS is orchestrated by a dyadic neuronal network, somewhat different from the one regulating NREMS, which either prevents the onset of REMS during wakefulness and NREMS, or upholds REMS initiation and continuation to the detriment of the other vigilance states [64, 65, 105] (Fig. 4). Alongside the hypothalamus, the brainstem is the main seat of REMS-controlling circuitry that involves a range of neurotransmitters and neuropeptides. Interestingly, several of these hypothalamic and brainstem-located neuronal clusters are active during both REMS and wakefulness [64, 65, 105].
Fig. 4
Summary of REMS-promoting pathways in the rodent brain. Glutamatergic, GABAergic, and cholinergic neurons from the PPT, LDT, and SLD, located in the pons, are principally responsible for the generation of REMS through projections to the thalamus, LH, and BF. They also suppress REMS-associated muscle activity through direct and indirect (via the vM) connections to spinal cord premotor interneurons and motoneurons, respectively. GABAergic and MCH-expressing neurons from the LH and ZI generate and maintain REMS by inhibiting wake-active and/or REMS-suppressing neuronal clusters in the BF, TMN, vPAG, LC, and DR (dashed lines). REMS is negatively modulated by interconnected midbrain nuclei such as the vPAG, DR, and LC that inhibit the PPT, LDT, and SLD. BF, basal forebrain; DR, dorsal raphe; LC, locus coeruleus; LDT, laterodorsal tegmental nucleus; LH, lateral hypothalamus; MCH, melanin concentrating hormone; PPT, pedunculopontine tegmental nucleus; SLD, sublaterodorsal tegmental nucleus; TMN, tuberomammillary nucleus of the hypothalamus; vM, vental medulla; vPAG, ventral periaqueductal gray; ZI, zona incerta. Created with BioRender.com.
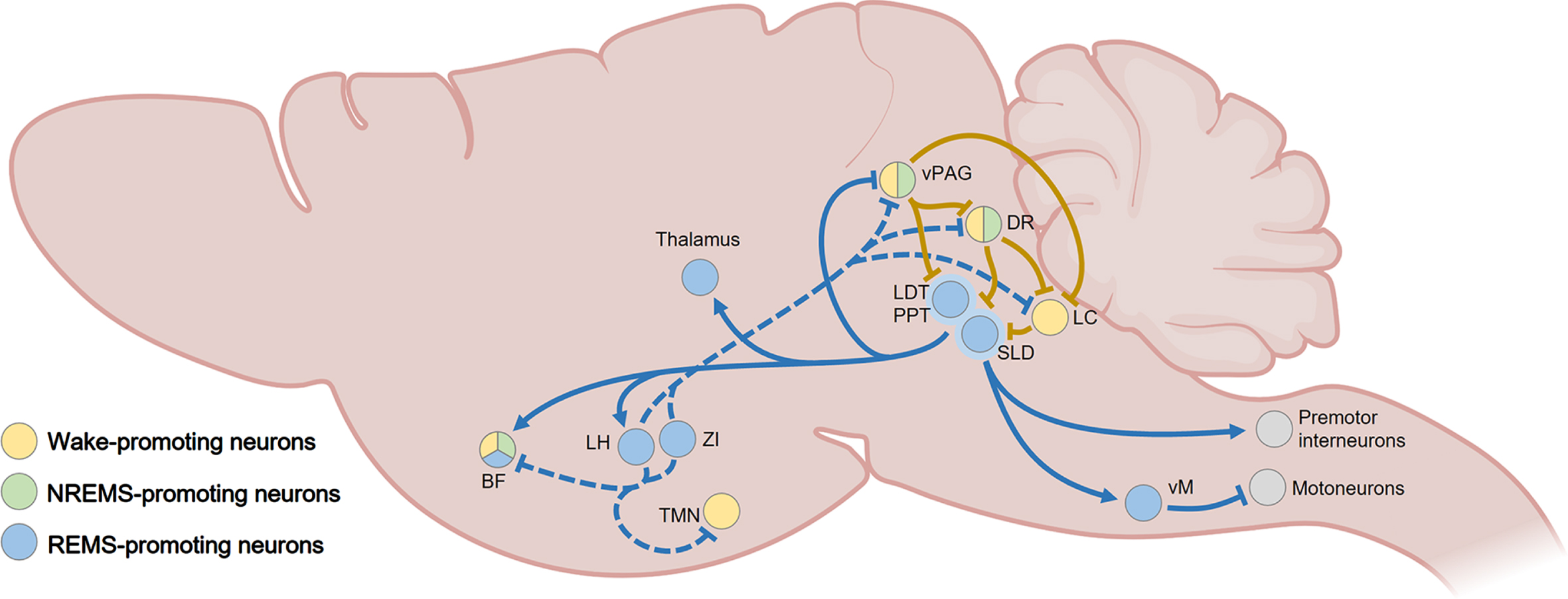
The suppression of REMS is principally achieved by GABAergic neurons located in two adjacent midbrain nuclei, the ventrolateral periaqueductal gray (vlPAG) and the dorsal part of the deep mesencephalic reticular nucleus [64, 65, 105]. They inhibit several REMS- and/or wake-promoting neuronal clusters from the midbrain and pons, including those in the SLD, the LDT, the LC and the DR [64, 65, 105]. They receive multiple inputs from sleep-wake regulatory brain regions [105], including inhibiting projections from REMS-active GABA containing neurons in the ventral medulla [108], the POA [65, 109], and the LH [110]. The LC and the DR are also important gatekeepers for REMS [105].
Several brainstem regions are responsible for the generation and maintenance of REMS. In the pons, the SLD contains glutamatergic, GABAergic, and cholinergic neurons, however only the activity of glutamatergic neurons has been consistently associated with REMS promotion [64, 105]. The glutamatergic projections to several nuclei of the thalamus, the hypothalamus and BF seem responsible for the REMS-associated desynchronization [105], while also suppress muscle activity during REMS through direct and indirect (via the vmM) connections to spinal cord interneurons and motoneurons, respectively [64]. Moreover, the PPT and LDT nuclei are composed of intermingled cholinergic, glutamatergic and GABAergic neurons that are though to serve as a densely innervated input and output hub for the modulation of REMS physiology [105]. Neurons from the PPT, LDT, and SLD have been implicated in the emergence of characteristic REMS phasic features. Through their interaction with the midbrain red nucleus and the thalamus [111, 112], they may be responsible for the myoclonic twitching [113, 114]. Furthermore, they fire in burst during REMS-associated PGO waves, and acetylcholine have been found to mediate PGO wave generation in the SLD [64].
In the medulla, a heterogeneous group of glycinergic and GABAergic nuclei with distinct neuronal connectivity regulate REMS. While ventral and dorsal medulla may support the initiation and persistence of REMS [64, 65, 105], the ventral part of medulla, which receive strong glutamatergic terminals from the SLD, has also been linked with the generation of REMS-associated atonia through projections to spinal cord motoneurons [115, 116]. A neuronal population of glycinergic/GABAergic neurons in the ventral medulla (lateral paragigantocellular nuclei), that is innervated by cholinergic axons, has been hypothesized to regulate the phasic increase of cardiac activity by inhibiting vagal neurons in the nucleus ambiguous [117]. In addition, the regulation of rapid eye movements could emanate from neurons expressing Calbindin and glutamate in the dorsal medulla nucleus papilio [118]. Of note, dopaminergic projections from the VTA to the basolateral amygdala have been recently implicated in REMS generation [119].
Outside the brainstem, the hypothalamus is also crucial for the regulation of REMS. The LH and the zona incerta (ZI) contain neurons releasing GABA and melanin-concentrating hormone (MCH) that are involved in the generation and maintenance of REMS [120–122]. MCH-expressing neurons can dynamically influence the predominance of REMS, notably through the inhibition of REMS-suppressing neurons in the vlPAG and wake-active neurons in the LH, BF, TMN, LC, and DR [64, 105]. They are locally modulated by several hypothalamic areas regulating the sleep-wake cycle, but also by the NAc, BF, PAG, and VTA among others [64, 105]. Galanin (GAL) is another neuropeptide that has been found to play a role in initiation and maintenance of REMS [105]. From the POA, galaninergic neurons project to sleep-regulating regions including the vlPAG, LDT, DRN, and LC [123, 124]. GAL in the dorsomedial hypothalamus may also support the REMS-associated suppression of thermoregulatory processes by receiving information from thermosensitive neurons in the POA and LH and then sending tonic inhibitory projections to the raphe pallidus that control thermogenesis [89, 125]. Neurons expressing NMDA receptors in the LPO are also essential for REMS [109]. Cholinergic, GABAergic and glutamatergic neurons in the BF, which is highly interconnected with the hippocampus, have been implicated in the generation of cortical and hippocampal theta oscillations during REMS [126].
SLEEP AND PATHOLOGIES: AN INTRICATE LINK
As mentioned above, sleep-wake regulation involves a myriad of molecular changes occurring in neural microcircuits spanning throughout the brain, forming a complex mechanism of biochemical gears. A certain level of redundancy in this sleep-wake regulatory system allows a degree of adaptability and suggests an essential physiological role, yet not fully understood [127]. Considering the wide distribution of sleep-regulating neuronal networks, the overlap with a number of pathological pathways is inevitable, and the defect of one or several shared gear(s) may lead to sleep impairments (Fig. 5). It is then not surprising to find that sleep disturbances are common in a multitude of neuropsychiatric and neurodegenerative diseases, such as major depressive disorder, bipolar disorders, post-traumatic stress disorder, schizophrenia, Alzheimer’s disease, Parkinson’s disease or Huntington’s disease [128]. For instance, the neuronal degeneration occurring in the cortex, basal ganglia, thalamus or hypothalamus of people living with Huntington’s [129, 130] or Alzheimer’s diseases [131, 132] may contribute to their sleep alterations [133–137], considering the involvement of those brain regions in sleep regulation [64, 65, 138]. The exact nature of these overlapping processes and their underpinning molecular roots are not understood. The diversity of sleep regulatory mechanisms, widespread throughout the brain, combined with specific pathological pathways give rise to different sleep alteration patterns for each illness. Usually, NREMS and/or REMS are positively or negatively affected, along with a change of total sleep time principally through the number of awakenings at night. The homeostatic control of sleep is not the only parameter impaired, since the circadian rhythmicity of sleep often deteriorates as well [2]. Indeed, the homeostatic and circadian processes underlying the regulation of sleep are deeply interweaved [51]. Circadian rhythm sleep-wake disorders are characterized by sleep onset occurring too early or too late, as well as irregular sleep patterns including excessive daytime sleep and night-time wake [2] (Fig. 6).
Fig. 5
Examples of overlaps between wake- and sleep-regulating neurons and various neurodegenerative diseases in the rodent brain. Several brain regions that exhibit neuropathological alterations in Alzheimer’s disease (e.g., hippocampus, neocortex, basal forebrain, thalamus, hypothalamus, striatum, brainstem), Parkinson’s disease (e.g., basal ganglia, substantia nigra, thalamus, hypothalamus) or Huntington’s disease (e.g., basal ganglia, substantia nigra, thalamus, hypothalamus) share neuronal signaling pathways with regions exerting control over sleep and wakefulness. BF, basal forebrain; DR, dorsal raphe; LC, locus coeruleus; LDT, laterodorsal tegmental nucleus; LH, lateral hypothalamus; NAc, nucleus accumbens; PBN, parabrachial nucleus; POA, preoptic area of the hypothalamus; PPT, pedunculopontine tegmental nucleus; PFZ, parafacial zone; SLD, sublaterodorsal tegmental nucleus; TMN, tuberomammillary nucleus of the hypothalamus; vM, vental medulla; vPAG, ventral periaqueductal gray; VTA, ventral tegmental area; ZI, zona incerta. Created with BioRender.com.
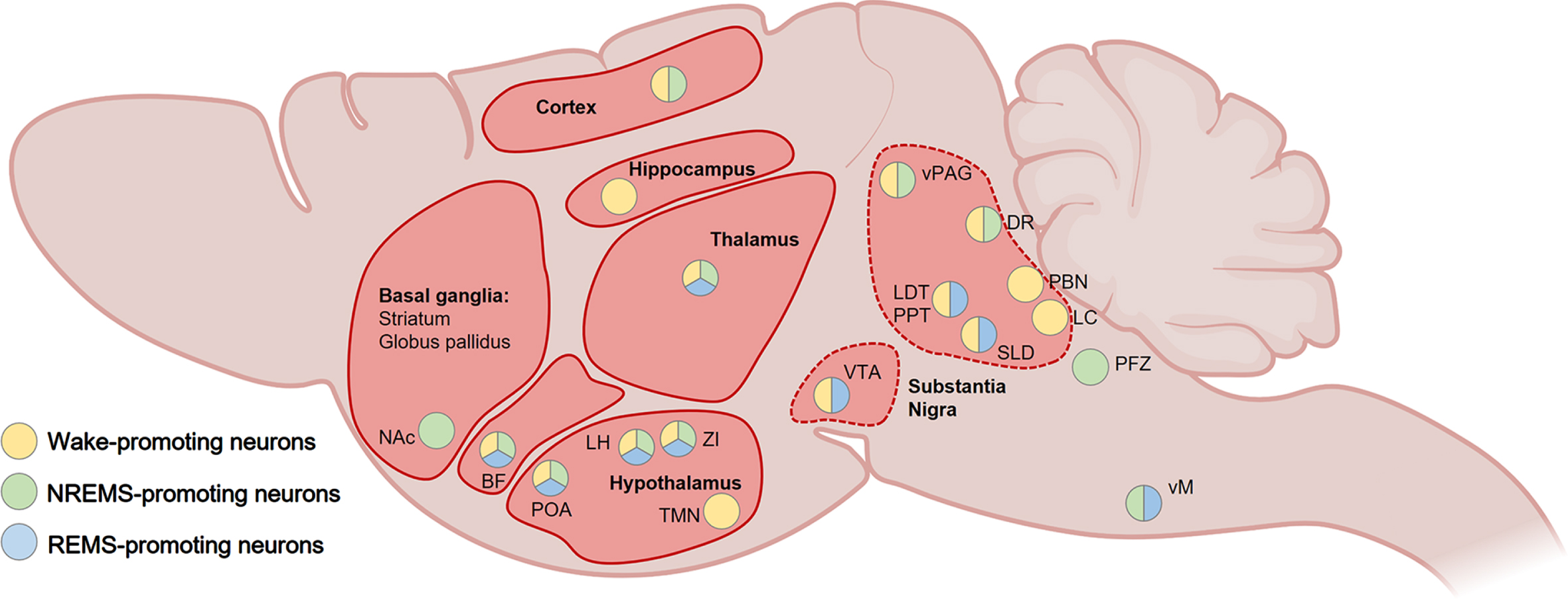
Fig. 6
Disturbances of the two-process model of sleep regulation. Simplified representation of the homeostatic process S (blue line) and circadian process C (brown lines) over a two-day period with 16 hours of wakefulness and 8 hours of sleep (grey bars) when an individual experiences sleep deprivation (red line) or irregular sleep pattern (purple line). During sleep deprivation, the individual stays awake when the increasing process S (blue line) reaches the upper threshold of process C, and sleep pressure continues to build up for 24 hours (increasing red line). Recovery sleep then allows the decrease of process S. An irregular sleep pattern is shown in purple, when an individual experiences frequent sleep episodes (decreasing sleep pressure) and awakenings (increasing sleep pressure) irrespective of normal wake and sleep time windows, and disconnected from circadian process C.
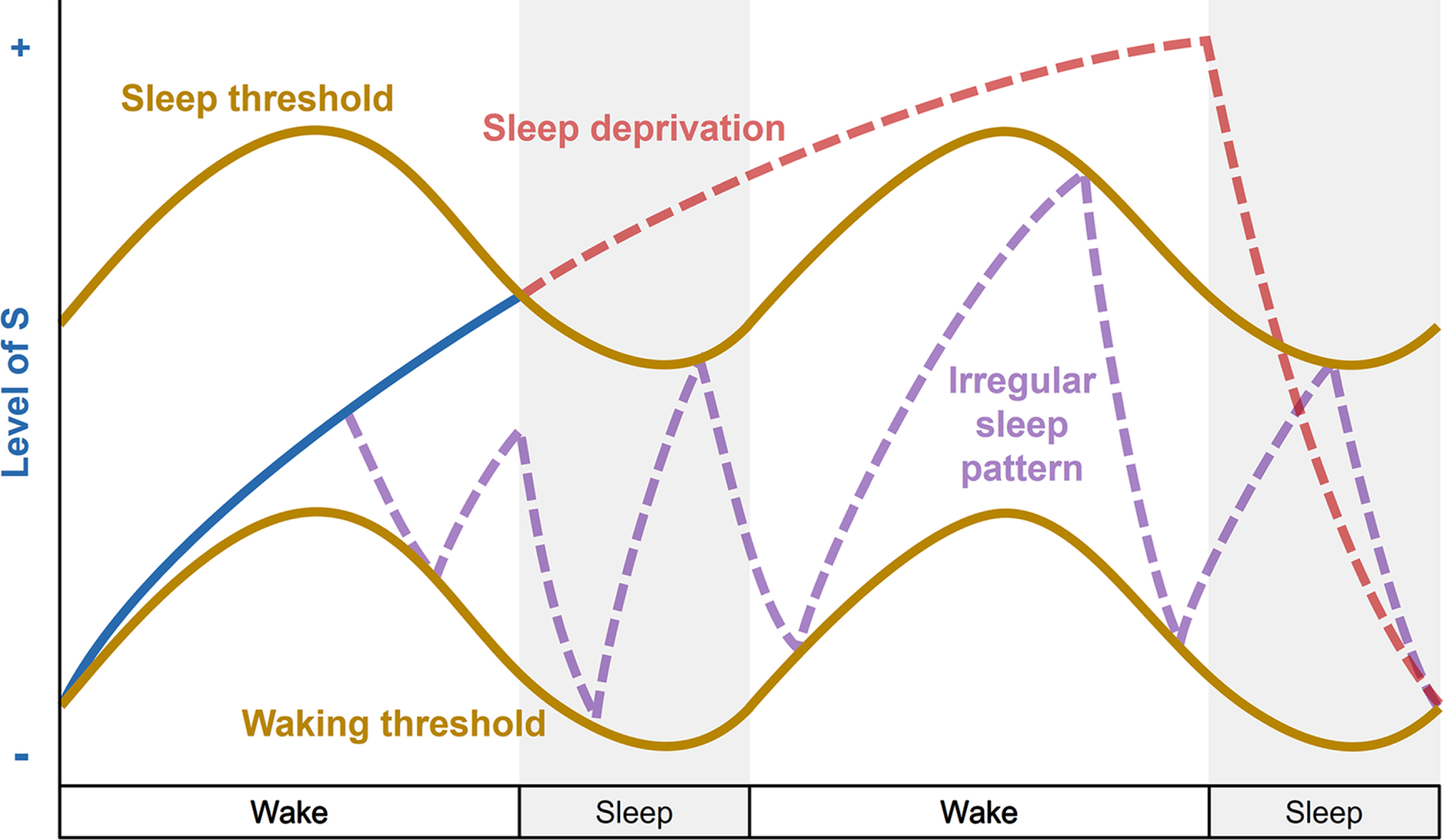
Whether sleep deterioration, and associated neurobiological alterations, precede the pathogenesis and thus represent a risk factor for the disease, or whether they are only a debilitating symptom of the pathophysiological alterations, is still unclear for most of the conditions. Regardless, sleep disturbances constitute an additional burden that can usually exacerbate the disease outcomes. Poor sleep quality and inadequate sleep duration can have dire repercussions on both physical and mental health, which could lead to obesity, diabetes, inflammation, cardiovascular diseases, cognitive impairments, anxiety and stress [1, 2].
In particular, stress can have strong detrimental effects on several pathogenic pathways [139], and the bidirectional link between sleep and stress has been well documented [21, 49, 50, 139]. The perturbation of sleep homeostasis is generally accompanied by an increase in hypothalamic-pituitary-adrenal (HPA) axis activity, leading to a rise in circulating levels of glucocorticoid stress hormones (e.g., cortisol in humans, corticosterone in rodents). On the other hand, the HPA-regulating neuropeptide corticotropin-releasing factor (CRF), as well as glucocorticoids, can alter sleep [140–142]. In a pathological context, this could generate a vicious cycle that further aggravate the other physiological and behavioral symptoms [139, 143]. In mice, GABA-somatostatin neurons in the VTA that are activated by stress induce NREMS (as mentioned earlier), but these cells also directly innervate and inhibit CRF-producing neurons in the paraventricular nucleus of the hypothalamus, thus shutting off excessive CRF production [50].
Beyond the detrimental aftermaths of many illnesses on sleep architecture, a wealth of evidence also sheds light on the risk of being afflicted by certain neurodegenerative and neuropsychiatric diseases following the onset of persistent sleep disruption [19]. For instance, individuals suffering from chronic sleep loss or insomnia display an increased risk of developing Alzheimer’s and Parkinson’s diseases, as well as mood disorders such as anxiety and major depression [19, 144, 145]. Considering the overlap between a number of disease pathways and sleep-regulating neuronal network, the initial failure of the sleep-wake system may affect specific pathological neural signaling. Inasmuch as the increasing prevalence of sleep disorders that can be driven at an individual, social and societal level [1, 6], this poses an important threat to the surge of severe comorbidities.
CONCLUSION
The complexity of the regulation and functions of sleep has sparked a long scientific interest and continues to be relentlessly uncovered by researchers worldwide. If some fundamental aspects of sleep remain a major knowledge gap, it is now clear that sleep is not just the absence of wakefulness, but a period of vital brain activity that has been conserved throughout evolution. In that respect, sleep serves an indispensable adaptative role, although its physiological and behavioral functions remain mysterious. Virtually all brain disorders encompass various degrees of sleep disturbances. Moreover, sleep’s contributions to mental and physical health have repeatedly been highlighted in epidemiological and clinical studies. This is the reason why it is of paramount importance to gain further insight into the purpose of sleep so we can better understand its crucial role in many pathologies. Novel biotechnological tools allowing region-, circuit- and cell-type-specific interrogations are at the cornerstone of recent progresses made at unravelling the biochemical regulatory machinery of sleep and wakefulness. By elucidating how we can manipulate and alleviate sleep, as well as the neurophysiological mechanisms underlying the development of sleep abnormalities in pathological context, we may be able to improve diagnosis and therapeutic interventions.
ACKNOWLEDGMENTS
This research was funded in whole, or in part, by the Wellcome Trust [Grant number 220759/Z/20/Z] to NPF and WW and the UK Dementia Research Institute to NPF and WW. For the purpose of open access, the authors have applied a CC BY public copyright license to any Author Accepted Manuscript version arising from this submission.
CONFLICT OF INTEREST
The authors have no conflict of interest to report.
REFERENCES
[1] | Grandner MA Sleep, health, and society. Sleep Med Clin. (2017) ;12: (1):1–22. |
[2] | Meyer N , Harvey AG , Lockley SW , Dijk D-J Circadian rhythms and disorders of the timing of sleep. Lancet. (2022) ;400: (10357):1061–78. |
[3] | Pavlova MK , Latreille V Sleep disorders. Am J Med. (2019) ;132: (3):292–9. |
[4] | da Mota Gomes M Encephalitis lethargica epidemic milestones in early sleep neurobiology researches. Sleep Med. (2020) ;74: :349–56. |
[5] | Schulz H The history of sleep research and sleep medicine in Europe. J Sleep Res. (2022) ;31: (4):e13602. |
[6] | Siegel JM Sleep function: An evolutionary perspective. Lancet Neurol. (2022) ;21: (10):937–46. |
[7] | Yamazaki R , Toda H , Libourel PA , Hayashi Y , Vogt KE , Sakurai T Evolutionary origin of distinct NREM and REM sleep. Front Psychol. (2020) ;11: :567618. |
[8] | Leung LC , Wang GX , Madelaine R , Skariah G , Kawakami K , Deisseroth K , et al. Neural signatures of sleep in zebrafish. Nature. (2019) ;571: (7764):198–204. |
[9] | Weber F , Dan Y Circuit-based interrogation of sleep control. Nature. (2016) ;538: (7623):51–9. |
[10] | Franken P , Malafosse A , Tafti M Genetic determinants of sleep regulation in inbred mice. Sleep. (1999) ;22: (2):155–69. |
[11] | Mander BA , Winer JR , Walker MP Sleep and human aging. Neuron. (2017) ;94: (1):19–36. |
[12] | Campos-Beltran D , Marshall L Changes in sleep EEG with aging in humans and rodents. Pflugers Arch. (2021) ;473: (5):841–51. |
[13] | Mallampalli MP , Carter CL Exploring sex and gender differences in sleep health: A Society for Women’s Health Research Report. J Womens Health (Larchmt). (2014) ;23: (7):553–62. |
[14] | Dib R , Gervais NJ , Mongrain V A review of the current state of knowledge on sex differences in sleep and circadian phenotypes in rodents. Neurobiol Sleep Circadian Rhythms. (2021) ;11: :100068. |
[15] | Jeon M , Dimitriou D , Halstead EJ A systematic review on cross-cultural comparative studies of sleep in young populations: The roles of cultural factors. Int J Environ Res Public Health. (2021) ;18: (4):2005. |
[16] | Hisler G , Twenge JM , Krizan Z Associations between screen time and short sleep duration among adolescents varies by media type: Evidence from a cohort study. Sleep Med. (2020) ;66: :92–102. |
[17] | Sabia S , Fayosse A , Dumurgier J , van Hees VT , Paquet C , Sommerlad A , et al. Association of sleep duration in middle and old age with incidence of dementia. Nat Commun. (2021) ;12: (1):2289. |
[18] | Wong ATY , Reeves GK , Floud S Total sleep duration and daytime napping in relation to dementia detection risk: Results from the Million Women Study. Alzheimers Dement (2023) . doi: 10.1002/alz.13009. |
[19] | Van Someren EJW Brain mechanisms of insomnia: New perspectives on causes and consequences. Physiol Rev. (2021) ;101: (3):995–1046. |
[20] | Franks NP , Wisden W The inescapable drive to sleep: Overlapping mechanisms of sleep and sedation. Science. (2021) ;374: (6567):556–9. |
[21] | Nollet M , Wisden W , Franks NP Sleep deprivation and stress: A reciprocal relationshiInterface Focus (2020) ;10: (3):20190092. |
[22] | Chu C , Holst SC , Elmenhorst EM , Foerges AL , Li C , Lange D , et al. Total sleep deprivation increases brain age prediction reversibly in multisite samples of young healthy adults. J Neurosci. (2023) ;43: (12):2168–77. |
[23] | Vaccaro A , Kaplan Dor Y , Nambara K , Pollina EA , Lin C , Greenberg ME , et al. Sleep loss can cause death through accumulation of reactive oxygen species in the gut. Cell. (2020) ;181: (6):1307–28e 15. |
[24] | Kendall-Bar JM , Williams TM , Mukherji R , Lozano DA , Pitman JK , Holser RR , et al. Brain activity of diving seals reveals short sleep cycles at depth. Science. (2023) ;380: (6642):260–5. |
[25] | Siegel JM Sleep viewed as a state of adaptive inactivity. Nat Rev Neurosci. (2009) ;10: (10):747–53. |
[26] | Schmidt MH The energy allocation function of sleep: A unifying theory of sleep, torpor, and continuous wakefulness. Neurosci Biobehav Rev. (2014) ;47: :122–53. |
[27] | Schmidt MH , Swang TW , Hamilton IM , Best JA State-dependent metabolic partitioning and energy conservation: A theoretical framework for understanding the function of sleep. PLoS One. (2017) ;12: (10):e0185746. |
[28] | Harding EC , Ba W , Zahir R , Yu X , Yustos R , Hsieh B , et al. Nitric oxide synthase neurons in the preoptic hypothalamus are NREM and REM sleep-active and lower body temperature. Front Neurosci. (2021) ;15: :709825. |
[29] | Harding EC , Franks NP , Wisden W Sleep and thermoregulation. Curr Opin Physiol. (2020) ;15: :7–13. |
[30] | Mackiewicz M , Shockley KR , Romer MA , Galante RJ , Zimmerman JE , Naidoo N , et al. Macromolecule biosynthesis: A key function of sleep. Physiol Genomics. (2007) ;31: (3):441–57. |
[31] | Besedovsky L , Lange T , Haack M The sleep-immune crosstalk in health and disease. Physiol Rev. (2019) ;99: (3):1325–80. |
[32] | Kayser MS , Biron D Sleep and development in genetically tractable model organisms. Genetics. (2016) ;203: (1):21–33. |
[33] | Krueger JM , Frank MG , Wisor JP , Roy S Sleep function: Toward elucidating an enigma. Sleep Med Rev. (2016) ;28: :46–54. |
[34] | Zada D , Bronshtein I , Lerer-Goldshtein T , Garini Y , Appelbaum L Sleep increases chromosome dynamics to enable reduction of accumulating DNA damage in single neurons. Nat Commun. (2019) ;10: (1):895. |
[35] | Tu BP , McKnight SL Metabolic cycles as an underlying basis of biological oscillations. Nat Rev Mol Cell Biol. (2006) ;7: (9):696–701. |
[36] | Wang Z , Ma J , Miyoshi C , Li Y , Sato M , Ogawa Y , et al. Quantitativephosphoproteomic analysis of the molecular substrates of sleep need. Nature. (2018) ;558: (7710):435–9. |
[37] | Tiriac A , Sokoloff G , Blumberg MS Myoclonic twitching andsleep-dependent plasticity in the developing sensorimotor system. Curr Sleep Med Re. (2015) ;1: (1):74–9. |
[38] | Rasmussen MK , Mestre H , Nedergaard M Fluid transport in the brain. Physiol Rev. (2022) ;102: (2):1025–151. |
[39] | Cirelli C , Gutierrez CM , Tononi G Extensive and divergent effects of sleep and wakefulness on brain gene expression. Neuron. (2004) ;41: (1):35–43. |
[40] | Marshall L , Helgadottir H , Molle M , Born J Boosting slow oscillations during sleep potentiates memory. Nature. (2006) ;444: (7119):610–3. |
[41] | Seibt J , Dumoulin MC , Aton SJ , Coleman T , Watson A , Naidoo N , et al. ((2012) ) Protein synthesis during sleep consolidates cortical plasticity in vivo. Curr Biol. 22: (8):676–82. |
[42] | Jha PK , Valekunja UK , Ray S , Nollet M , Reddy AB Single-cell transcriptomics and cell-specific proteomics reveals molecular signatures of sleep. Commun Biol. (2022) ;5: (1):846. |
[43] | de Vivo L , Bellesi M , Marshall W , Bushong EA , Ellisman MH , Tononi G , et al. Ultrastructural evidence for synaptic scaling across the wake/sleep cycle. Science. (2017) ;355: (6324):507–10. |
[44] | Diering GH , Nirujogi RS , Roth RH , Worley PF , Pandey A , Huganir RL Homer1a drives homeostatic scaling-down of excitatory synapses during sleep. Science. ((2017) );335: (6324):511–5. |
[45] | Dudai Y , Karni A , Born J The consolidation and transformation of memory. Neuron. (2015) ;88: (1):20–32. |
[46] | Boyce R , Williams S , Adamantidis A REM sleep and memory. Curr Opin Neurobiol. (2017) ;44: :167–77. |
[47] | Peever J , Fuller PM The biology of REM sleep.. Curr Biol. (2017) ;27: (22):R1237–R48. |
[48] | Wagner U , Gais S , Haider H , Verleger R , Born J Sleep inspires insight. Nature. (2004) ;427: (6972):352–5. |
[49] | Nollet M , Hicks H , McCarthy AP , Wu H , Moller-Levet CS , Laing EE , et al. REM sleep’s unique associations with corticosterone regulation, apoptotic pathways, and behavior in chronic stress in mice. Proc Natl Acad Sci U S A. (2019) ;116: (7):2733–42. |
[50] | Yu X , Zhao G , Wang D , Wang S , Li R , Li A , et al. A specific circuit in the midbrain detects stress and induces restorative sleep. Science. (2022) ;377: (6601):63–72. |
[51] | Borbely A The two-process model of sleep regulation: Beginnings and outlook. J Sleep Res. (2022) ;31: (4):e13598. |
[52] | Hastings MH , Maywood ES , Brancaccio M Generation of circadian rhythms in the suprachiasmatic nucleus. Nat Rev Neurosci. (2018) ;19: (8):453–69. |
[53] | Collins B , Pierre-Ferrer S , Muheim C , Lukacsovich D , Cai Y , Spinnler A , et al. Circadian VIPergic neurons of the suprachiasmatic nuclei sculpt the sleep-wake cycle. Neuron. (2020) ;108: (3):486–l99.e5. |
[54] | Todd WD , Venner A , Anaclet C , Broadhurst RY , De Luca R , Bandaru SS , et al. Suprachiasmatic VIP neurons are required for normal circadian rhythmicity and comprised of molecularly distinct subpopulations. Nat Commun. (2020) ;11: (1):4410. |
[55] | Li Z , Sheth AB , Sheth BR What drives slow wave activity during early non-REM sleep: Learning during prior wake or effort? PLoS One. (2017) ;12: (10):e0185681. |
[56] | Leenaars CHC , Savelyev SA , Van der Mierden S , Joosten R , Dematteis M , Porkka-Heiskanen T , et al. Intracerebral adenosine during sleep deprivation: A meta-analysis and new experimental data. J Circadian Rhythms. (2018) ;16: :11. |
[57] | Peng W , Wu Z , Song K , Zhang S , Li Y , Xu M Regulation of sleep homeostasis mediator adenosine by basal forebrain glutamatergic neurons. Science. (2020) ;369: (6508). |
[58] | Lazarus M , Oishi Y , Bjorness TE , Greene RW Gating and the need for sleep: Dissociable effects of adenosine A(1) and A(2A) receptors. Front Neurosci. (2019) ;13: :740. |
[59] | Porkka-Heiskanen T Sleep homeostasis. Curr Opin Neurobiol. (2013) ;23: (5):799–805. |
[60] | He Y , Jones CR , Fujiki N , Xu Y , Guo B , Holder JL , et al. The transcriptional repressor DEC2 regulates sleep length in mammals. Science. (2009) ;325: (5942):866–70. |
[61] | Kim SJ , Hotta-Hirashima N , Asano F , Kitazono T , Iwasaki K , Nakata S , et al. Kinase signalling in excitatory neurons regulates sleep quantity and depth. Nature. (2022) ;612: (7940):512–8. |
[62] | Zhou R , Wang G , Li Q , Meng F , Liu C , Gan R , et al. A signalling pathway for transcriptional regulation of sleep amount in mice. Nature. (2022) ;612: (7940):519–27. |
[63] | Saper CB , Chou TC , Scammell TE The sleep switch: Hypothalamic control of sleep and wakefulness. Trends Neurosci. (2001) ;24: (12):726–31. |
[64] | Sulaman BA , Wang S , Tyan J , Eban-Rothschild A Neuro-orchestration of sleep and wakefulness. Nat Neurosci. (2023) ;26: (2):196–212. |
[65] | Scammell TE , Arrigoni E , Lipton JO Neural circuitry of wakefulness and sleep. Neuron. (2017) ;93: (4):747–65. |
[66] | Hoffman LA , Vilensky JA Encephalitis lethargica: 100 years after the epidemic. Brain. (2017) ;140: (8):2246–51. |
[67] | Pauli JL , Chen JY , Basiri ML , Park S , Carter ME , Sanz E , et al. Molecular and anatomical characterization of parabrachial neurons and their axonal projections. Elife. (2022) ;11: :e81868. |
[68] | Anaclet C , Pedersen NP , Ferrari LL , Venner A , Bass CE , Arrigoni E , et al. Basal forebrain control of wakefulness and cortical rhythms. Nat Commun. (2015) ;6: :8744. |
[69] | Han Y , Shi YF , Xi W , Zhou R , Tan ZB , Wang H , et al. Selective activation of cholinergic basal forebrain neurons induces immediate sleep-wake transitions. Curr Biol. (2014) ;24: (6):693–8. |
[70] | Xu M , Chung S , Zhang S , Zhong P , Ma C , Chang WC , et al. Basal forebrain circuit for sleep-wake control. Nat Neurosci. (2015) ;18: (11):1641–7. |
[71] | Yamashita T , Yamanaka A Lateral hypothalamic circuits for sleep-wake control. Curr Opin Neurobiol. (2017) ;44: :94–100. |
[72] | Yu X , Li W , Ma Y , Tossell K , Harris JJ , Harding EC , et al. GABA and glutamate neurons in the VTA regulate sleep and wakefulness. Nat Neurosci. (2019) ;22: (1):106–19. |
[73] | Feng H , Wen SY , Qiao QC , Pang YJ , Wang SY , Li HY , et al. Orexin signaling modulates synchronized excitation in the sublaterodorsal tegmental nucleus to stabilize REM sleep. Nat Commun. (2020) ;11: (1):3661. |
[74] | Iyer M , Essner RA , Klingenberg B , Carter ME Identification of discrete, intermingled hypocretin neuronal populations. J Comp Neurol. (2018) ;526: (18):2937–54. |
[75] | Krahn LE , Zee PC , Thorpy MJ Current understanding of narcolepsy 1 and its comorbidities: What clinicians need to know. Adv Ther. (2022) ;39: (1):221–43. |
[76] | Fujita A , Bonnavion P , Wilson MH , Mickelsen LE , Bloit J , de Lecea L , et al. Hypothalamic tuberomammillary nucleus neurons: Electrophysiological diversity and essential role in arousal stability. J Neurosci. (2017) ;37: (39):9574–92. |
[77] | Yu X , Ma Y , Harding EC , Yustos R , Vyssotski AL , Franks NP , et al. Genetic lesioning of histamine neurons increases sleep-wake fragmentation and reveals their contribution to modafinil-induced wakefulness. Sleep. (2019) ;42: (5):zsz031. |
[78] | Liang Y , Shi W , Xiang A , Hu D , Wang L , Zhang L The NAergic locus coeruleus-ventrolateral preoptic area neural circuit mediates rapid arousal from sleep. Curr Biol. (2021) ;31: (17):3729–42 e5. |
[79] | Osorio-Forero A , Cherrad N , Banterle L , Fernandez LMJ , Luthi A When the locus coeruleus speaks up in sleep: Recent insights, emerging perspectives. Int J Mol Sci. (2022) ;23: (9):5028. |
[80] | Lu J , Jhou TC , Saper CB Identification of wake-active dopaminergic neurons in the ventral periaqueductal gray matter. J Neurosci. (2006) ;26: (1):193–202. |
[81] | Cho JR , Treweek JB , Robinson JE , Xiao C , Bremner LR , Greenbaum A , et al. Dorsal raphe dopamine neurons modulate arousal and promote wakefulness by salient stimuli. Neuron. (2017) ;94: (6):1205–19 e8. |
[82] | Cui SY , Li SJ , Cui XY , Zhang XQ , Yu B , Huang YL , et al. Ca(2+) in the dorsal raphe nucleus promotes wakefulness via endogenous sleep-wake regulating pathway in the rats. Mol Brain. (2016) ;9: (1):71. |
[83] | Monti JM The role of dorsal raphe nucleus serotonergic and non-serotonergic neurons, and of their receptors, in regulating waking and rapid eye movement (REM) sleep. Sleep Med Rev. (2010) ;14: (5):319–27. |
[84] | Oikonomou G , Altermatt M , Zhang RW , Coughlin GM , Montz C , Gradinaru V , et al. The serotonergic raphe promote sleep in zebrafish and mice. Neuron. (2019) ;103: (4):686–701 e8. |
[85] | Venner A , Broadhurst RY , Sohn LT , Todd WD , Fuller PM Selective activation of serotoninergic dorsal raphe neurons facilitates sleep through anxiolysis. Sleep. (2020) ;43: (2):zsz231. |
[86] | Eban-Rothschild A , Rothschild G , Giardino WJ , Jones JR , de Lecea L VTA dopaminergic neurons regulate ethologically relevant sleep-wake behaviors. Nat Neurosci. (2016) ;19: (10):1356–66. |
[87] | Sotelo MI , Tyan J , Markunas C , Sulaman BA , Horwitz L , Lee H , et al. Lateral hypothalamic neuronal ensembles regulate pre-sleep nest-building behavior. Curr Biol. (2022) ;32: (4):806–22 e7. |
[88] | Harding EC , Yu X , Miao A , Andrews N , Ma Y , Ye Z , et al. A neuronal hub binding sleep initiation and body cooling in response to a warm external stimulus. Curr Biol. (2018) ;28: (14):2263–73 4e. |
[89] | Rothhaas R , Chung S Role of the preoptic area in sleep and thermoregulation. Front Neurosci. (2021) ;15: :664781. |
[90] | Zhang GW , Shen L , Tao C , Jung AH , Peng B , Li Z , et al. Medial preoptic area antagonistically mediates stress-induced anxiety and parental behavior. Nat Neurosci. (2021) ;24: (4):516–28. |
[91] | Anaclet C , Ferrari L , Arrigoni E , Bass CE , Saper CB , Lu J , et al. The GABAergic parafacial zone is a medullary slow wave sleep-promoting center. Nat Neurosci. (2014) ;17: (9):1217–24. |
[92] | Zhong P , Zhang Z , Barger Z , Ma C , Liu D , Ding X , et al. Control of non-REM sleep by midbrain neurotensinergic neurons. Neuron. (2019) ;104: (4):795–809 e6. |
[93] | Zhang Z , Zhong P , Hu F , Barger Z , Ren Y , Ding X , et al. An excitatory circuit in the perioculomotor midbrain for non-REM sleep control. Cell. (2019) ;177: (5):1293–307 e16. |
[94] | Herrera CG , Cadavieco MC , Jego S , Ponomarenko A , Korotkova T , Adamantidis A Hypothalamic feedforward inhibition of thalamocortical network controls arousal and consciousness. Nat Neurosci. (2016) ;19: (2):290–8. |
[95] | Ni KM , Hou XJ , Yang CH , Dong P , Li Y , Zhang Y , et al. Selectively driving cholinergic fibers optically in the thalamic reticular nucleus promotes sleep. Elife. (2016) ;5: :e10382. |
[96] | Ma C , Zhong P , Liu D , Barger ZK , Zhou L , Chang WC , et al. Sleep regulation by neurotensinergic neurons in a thalamo-amygdala circuit. Neuron. (2019) ;103: (2):323–34 e7. |
[97] | Lai YY , Kodama T , Hsieh KC , Nguyen D , Siegel JM Substantia nigra pars reticulata-mediated sleep and motor activity regulation. Sleep. (2021) ;44: (1):zsaa151. |
[98] | Liu D , Li W , Ma C , Zheng W , Yao Y , Tso CF , et al. A common hub for sleep and motor control in the substantia nigra. Science. (2020) ;367: (6476):440–5. |
[99] | Hasegawa H , Selway R , Gnoni V , Beniczky S , Williams SCR , Kryger M , et al. The subcortical belly of sleep: New possibilities in neuromodulation of basal ganglia? Sleep Med Rev. (2020) ;52: :101317. |
[100] | Li R , Wang YQ , Liu WY , Zhang MQ , Li L , Cherasse Y , et al. Activation of adenosine A(2A) receptors in the olfactory tubercle promotes sleep in rodents. Neuropharmacology. (2020) ;168: :107923. |
[101] | Oishi Y , Xu Q , Wang L , Zhang BJ , Takahashi K , Takata Y , et al. Slow-wave sleep is controlled by a subset of nucleus accumbens core neurons in mice. Nat Commun. (2017) ;8: (1):734. |
[102] | Yuan XS , Wang L , Dong H , Qu WM , Yang SR , Cherasse Y , et al. Striatal adenosine A(2A) receptor neurons control active-period sleep via parvalbumin neurons in external globus pallidus. Elife. (2017) ;6: :e29055. |
[103] | Krone LB , Yamagata T , Blanco-Duque C , Guillaumin MCC , Kahn MC , van der Vinne V , et al. A role for the cortex in sleep-wake regulation. Nat Neurosci. (2021) ;24: (9):1210–5. |
[104] | Simor P , van der Wijk G , Nobili L , Peigneux P The microstructure of REM sleep: Why phasic and tonic? Sleep Med Rev. (2020) ;52: :101305. |
[105] | Herice C , Patel AA , Sakata S Circuit mechanisms and computational models of REM sleep. Neurosci Res. (2019) ;140: :77–92. |
[106] | Lee M , Sanz LRD , Barra A , Wolff A , Nieminen JO , Boly M , et al. Quantifying arousal and awareness in altered states of consciousness using interpretable deep learning. Nat Commun. (2022) ;13: (1):1064. |
[107] | ScarpelliS, BartolacciC, D’AtriA, GorgoniM, De GennaroL. The functional role of dreaming in emotional processes. Front Psychol. (2019) ;10: :459. |
[108] | Weber F , Chung S , Beier KT , Xu M , Luo L , Dan Y Control of REM sleep by ventral medulla GABAergic neurons. Nature. (2015) ;526: (7573):435–8. |
[109] | Miracca G , Anuncibay-Soto B , Tossell K , Yustos R , Vyssotski AL , Franks NP , et al. NMDA receptors in the lateral preoptic hypothalamus are essential for sustaining NREM and REM sleep. J Neurosci. ((2022) ;42: (27):5389–409. |
[110] | Clement O , Sapin E , Libourel PA , Arthaud S , Brischoux F , Fort P , et al. The lateral hypothalamic area controls paradoxical (REM) sleep by means of descending projections to brainstem GABAergic neurons. J Neurosci. (2012) ;32: (47):16763–74. |
[111] | Del Rio-Bermudez C , Sokoloff G , Blumberg MS Sensorimotor processing in the newborn rat red nucleus during active sleep. J Neurosci. (2015) ;35: (21):8322–32. |
[112] | Beak SK , Hong EY , Lee HS Collateral projection from the forebrain and mesopontine cholinergic neurons to whisker-related, sensory and motor regions of the rat. Brain Res. (2010) ;1336: :30–45. |
[113] | Cisse Y , Ishibashi M , Jost J , Toossi H , Mainville L , Adamantidis A , et al. Discharge and role of GABA pontomesencephalic neurons incortical activity and sleep-wake states examined by optogenetics andjuxtacellular recordings in mice. J Neurosci. (2020) ;40: (31):5970–89. |
[114] | Cisse Y , Toossi H , Ishibashi M , Mainville L , Leonard CS , Adamantidis A , et al. Discharge and role of acetylcholine pontomesencephalic neurons in cortical activity and sleep-wake states examined by optogenetics and juxtacellular recording in mice. eNeuro.ENEURO. (2018) ;5: (4):ENEURO.0270-18.2018. |
[115] | Uchida S , Soya S , Saito YC , Hirano A , Koga K , Tsuda M , et al. A discrete glycinergic neuronal population in the ventromedial medulla that induces muscle atonia during REM sleep and cataplexy in mice. J Neurosci. (2021) ;41: (7):1582–96. |
[116] | Valencia Garcia S , Brischoux F , Clement O , Libourel PA , Arthaud S , Lazarus M , et al. Ventromedial medulla inhibitory neuron inactivation induces REM sleep without atonia and REM sleep behavior disorder. Nat Commun. (2018) ;9: (1):504. |
[117] | Dergacheva O , Wang X , Lovett-Barr MR , Jameson H , Mendelowitz D The lateral paragigantocellular nucleus modulates parasympathetic cardiac neurons: A mechanism for rapid eye movement sleep-dependent changes in heart rate. J Neurophysiol. (2010) ;104: (2):685–94. |
[118] | Gutierrez Herrera C , Girard F , Bilella A , Gent TC , Roccaro-Waldmeyer DM , Adamantidis A , et al. Neurons in the Nucleus papilio contribute to the control of eye movements during REM sleep. Nat Commun. (2019) ;10: (1):5225. |
[119] | Hasegawa E , Miyasaka A , Sakurai K , Cherasse Y , Li Y , Sakurai T Rapid eye movement sleep is initiated by basolateral amygdala dopamine signaling in mice. Science. (2022) ;375: (6584):994–1000. |
[120] | Blanco-Centurion C , Luo S , Vidal-Ortiz A , Swank C , Shiromani PJ Activity of a subset of vesicular GABA-transporter neurons in the ventral zona incerta anticipates sleep onset. Sleep. (2021) ;44: (6):zsaa268. |
[121] | Bandaru SS , Khanday MA , Ibrahim N , Naganuma F , Vetrivelan R Sleep-wake control by melanin-concentrating hormone (MCH) neurons: A review of recent findings. Curr Neurol Neurosci Re. (2020) ;20: (12):55. |
[122] | Hassani OK , Henny P , Lee MG , Jones BE GABAergic neurons intermingled with orexin and MCH neurons in the lateral hypothalamus discharge maximally during sleep. Eur J Neurosci. (2010) ;32: (3):448–57. |
[123] | Lu J , Bjorkum AA , Xu M , Gaus SE , Shiromani PJ , Saper CB Selective activation of the extended ventrolateral preoptic nucleus during rapid eye movement sleep. J Neurosci (2002) ;22: (11):4568–76. |
[124] | Lu J , Sherman D , Devor M , Saper CB A putative flip-flop switch for control of REM sleep.. Nature. (2006) ;441: (7093):589–94. |
[125] | Chen KS , Xu M , Zhang Z , Chang WC , Gaj T , Schaffer DV , et al. A Hypothalamic switch for REM and non-REM sleep. Neuron. (2018) ;97: (5):1168–76 e4. |
[126] | Yang C , Thankachan S , McCarley RW , Brown RE The menagerie of the basal forebra: How many (neural) species are there, what do they look like, how do they behave and who talks to whom? Curr Opin Neurobiol. (2017) ;44: :159–66. |
[127] | Zielinski MR , McKenna JT , McCarley RW Functions and mechanisms of sleep. AIMS Neurosci. (2016) ;3: (1):67–104. |
[128] | Zhang Y , Ren R , Yang L , Zhang H , Shi Y , Vitiello MV , et al. Patterns of polysomnography parameters in 27 neuropsychiatric diseases: An umbrella review. Psychol Med. (2022) . doi: 10.1017/S0033291722001581. |
[129] | Blumenstock S , Dudanova I Cortical and striatal circuits in Huntington’s disease. Front Neurosci. (2020) ;14: :82. |
[130] | Petersen A , Gabery S Hypothalamic and limbic system changes in Huntington’s disease. J Huntingtons Dis. (2012) ;1: (1):5–16. |
[131] | Cho H , Kim JH , Kim C , Ye BS , Kim HJ , Yoon CW , et al. Shape changes of the basal ganglia and thalamus in Alzheimer’s disease: A three-year longitudinal study. J Alzheimers Dis. (2014) ;40: (2):285–95. |
[132] | DeTure MA , Dickson DW The neuropathological diagnosis of Alzheimer’s disease. Mol Neurodegener. (2019) ;14: (1):32. |
[133] | Herzog-Krzywoszanska R , Krzywoszanski L Sleep disorders in Huntington’s disease. Front Psychiatry. (2019) ;10: :221. |
[134] | Vas S , Nicol AU , Kalmar L , Miles J , Morton AJ Abnormal patterns of sleep and EEG power distribution during non-rapid eye movement sleep in the sheep model of Huntington’s disease. Neurobiol Dis. (2021) ;155: :105367. |
[135] | Falgas N , Walsh CM , Yack L , Simon AJ , Allen IE , Kramer JH , et al. Alzheimer’s disease phenotypes show different sleep architecture. Alzheimers Dement. (2023) . doi: 10.1002/alz.12963. |
[136] | Rigat L , Ouk K , Kramer A , Priller J Dysfunction of circadian and sleep rhythms in the early stages of Alzheimer’s disease. Acta Physiol (Oxf). (2023) ;e13970. |
[137] | Shen Y , Lv QK , Xie WY , Gong SY , Zhuang S , Liu JY , et al. Circadian disruption and sleep disorders in neurodegeneration. Transl Neurodegener. (2023) ;12: (1):8. |
[138] | Lazarus M , Chen JF , Urade Y , Huang ZL Role of the basal ganglia in the control of sleep and wakefulness. Curr Opin Neurobiol. (2013) ;23: (5):780–5. |
[139] | O’Connor DB , Thayer JF , Vedhara K Stress and health: A review of psychobiological processes. Ann Rev Psychol. (2021) ;72: (1):663–88. |
[140] | Hirotsu C , Tufik S , Andersen ML Interactions between sleep, stress, and metabolism: From physiological to pathological conditions. Sleep Sci. (2015) ;8: (3):143–52. |
[141] | Tseng Y-T , Zhao B , Chen S , Ye J , Liu J , Liang L , et al. The subthalamic corticotropin-releasing hormone neurons mediate adaptive REM-sleep responses to threat. Neuron. (2022) ;110: (7):1223–39.e8. |
[142] | Chung S , Weber F , Zhong P , Tan CL , Nguyen TN , Beier KT , et al. Identification of preoptic sleep neurons using retrograde labelling and gene profiling. Nature. (2017) ;545: (7655):477–81. |
[143] | Irish LA , Kline CE , Gunn HE , Buysse DJ , Hall MH The role of sleep hygiene in promoting public health: A review of empirical evidence. Sleep Med Rev. (2015) ;22: :23–36. |
[144] | Johansson M , Jansson-Fröjmark M , Norell-Clarke A , Linton SJ Changes in insomnia as a risk factor for the incidence and persistence of anxiety and depression: A longitudinal community study. Sleep Sci Pract. (2021) ;5: (1):5. |
[145] | Palma J-A , Urrestarazu E , Iriarte J Sleep loss as risk factor for neurologic disorders: A review. Sleep Med. (2013) ;14: (3):229–36. |