Effect of nighttime UV-C irradiation of strawberry plants on phenolic content of fruit: Targeted and non-targeted metabolomic analysis
Abstract
BACKGROUND:
The new approach of using UV-C irradiation followed by a specific dark period to control plant diseases has the potential to become a mainstream treatment in the production of strawberries and other fruits and vegetables. Thus, it is imperative to study the effects of this treatment on fruit quality.
METHODS:
In this study, short-day ‘Chandler’ strawberry plants grown in growth chamber from bloom to harvest were irradiated twice a week with nighttime UV-C light (253 nm peak value 12.36 J m–2 and the total dose of 50 J m–2 in the range of 240 –260 nm). The effects on the content of key phenolic compounds in mature fruit were studied with targeted analysis and a non-targeted metabolomic approach based on ultra-high performance liquid chromatography high resolution mass spectrometry (UHPLC-HRMS). Analysis of variance-principal component analysis (ANOVA-PCA) was used to associate variance with different experimental factors.
RESULTS:
Results indicate that the content of anthocyanins, glucosides and glucuronides of quercetin and kaempferol, catechin, pelargonidin rutinoside, and ellagic acid was not affected by UV-C treatment. ANOVA-PCA analysis of the metabolomic dataset showed no significant differences in composition and content of strawberry metabolites between UV-C and control groups; however, differences were observed between harvest times.
CONCLUSIONS:
Our findings strongly indicate that UV-C treatment of 50 J/m2 twice per week did not affect nutritional values of strawberry fruit. The metabolomic approach combined with ANOVA-PCA used to evaluate strawberry fruit quality after UV-C irradiation proved to be a very powerful tool in providing an overall insight into fruit quality and accurately determines the influence of each experimental factor.
1Introduction
Strawberry (Fragaria×ananassa Duch.) is the fourth most consumed fruit, after bananas, apples and grapes in the United States [1]. The fruit is valued for its culinary as well as health benefits [2]. Strawberries sold in supermarkets come mostly from open field farms in California, Florida, and Mexico, of which 89% are produced in California [3]. However, a confluence of factors unique to the strawberry industry is making its future quite uncertain. High up-front costs, labor shortages, increased pesticide regulation, the cost of land, and the impacts of climate change, including hotter and drier conditions have had adverse impacts on the strawberry industry in California and other major production areas that have relied heavily on pre-plant fumigation to obtain high yields and to use same fields to grow strawberries year after year. These days, berry producers are embracing innovations, including organic alternatives to chemical fumigants, soilless growing systems, and breeding for disease resistance to develop more sustainable, resilient strawberry production technologies. Currently in the United States, strawberries harvested from plants in protected environments such as high tunnels and greenhouses supply less than 0.01% of annual consumption. Control of diseases and pests is the major challenge to growing strawberry both in the open field as well as in protected environment systems. Fungicides are routinely used to control disease; however, they are becoming less effective due to the development of resistance in the pathogens [4, 5]. In addition, a growing demand for produce free of pesticides is creating needs for alternative disease control strategies.
Gray mold of strawberry fruit caused by Botrytis cinerea is one of the most important diseases of this crop and as much as 80% of the fruit decays originate from flower infections, therefore, control of the disease that is manifested at and after harvest must start at bloom time [6]. Nevertheless, several attempts have been made to control this decay after harvest with UV-C irradiation. So far, the success has been limited. Only a moderate reduction in strawberry gray mold was observed from postharvest treatment with UV-C doses of 0.5 –1.00 kJ m-2 and it was attributed mainly to induction of host resistance responses. The direct effect on the external contamination with the pathogen was not excluded [7]. Similarly, B. cinerea growth on inoculated strawberries was significantly reduced with doses of 0.05–0.1 J cm -2 (= 0.5–1.00 kJ m-2) and the onset of observed fungal growth was delayed by only 1-2 d [8, 9]. At higher doses damage to sepals was observed [10]. In vitro studies showed that a heat treatment (40°C) of conidia prior to UV-C irradiation improved killing of the conidia [11]. But this does not appear to have a practical application for the strawberry cold and retail supply chain. In general, attempts to control plant diseases using UV-C either as a pre- or post-harvest treatment have not been successful because of the phytotoxicity at doses required for killing pathogens. This has changed recently after Janisiewicz et al. [12, 13], showed that a specific dark period (depending on pathogen) immediately after UV-C treatment enhanced the killing power of the UV-C. Apparently, the dark period prevents pathogens from activation of light-induced enzymes involved in repairing damaged DNA as a result of UV-C irradiation. This allows effective doses below phytotoxic levels and made the use of UV-C treatment for control of plant disease practical. Nighttime UV-C irradiation of strawberry plants with reduced doses of 50 J m–2 every 3-4 d from bloom until harvest has been used successfully to reduce not only gray mold, anthracnose, and powdery mildew but arthropod pests as well [14].
Now that UV-C irradiation is being considered for sterilizing the surfaces of fruits and vegetables, there is the need to evaluate the positive and negative aspects of this treatment on phytonutrients including polyphenols. Phenolics impact fruit and vegetable quality by effecting organoleptic properties and having beneficial effects on human health [15]. This has inspired many researchers to explore various avenues to manipulate phenolics content in different fruits and vegetables. Increases in total phenolic content or variety of different phenolics were observed after irradiation with hermetic doses of UV-C in winter jujube [16], rocket salads [17], lettuce [18] or spinach [19]. UV-C irradiation was shown to increase stilbenoids, catechins and trans-resveratrol in grape berries irradiated 3 d before or 2 d after harvest [20, 21]. A pulsed UV-C irradiation of harvested table grapes increased resveratrol in berries suggesting a novel approach in the development “functional” fruit [22]. However, UV-C irradiation was not effective for the enhancement of polyphenol, β-carotene, ascorbic acid, or chlorophyll in persimmon and cucumber [23].
In strawberry, preharvest UV-C treatment has been reported to increase a variety of phenolic compounds [24–30]. Treatment of fruits at different stages of maturity with UV-C had various effects on phenolic content such as transient effect on content of anthocyanins in harvested large green strawberries [31] and a higher ellagic acid content in strawberry fruit harvested from plants irradiated twice a week from the onset of flowering until harvest with 60 mJ cm-2 (= 0.6 kJ m-2) [32]. As the ellagic acid concentration of strawberries declines with fruit maturation [33], the UV-C treatment can have a positive effect on maintaining a high level of this health beneficial compound [32, 34]. A recent study indicates the potential to stimulate the accumulation of phenolic compounds in ripe strawberry fruit following UV-C irradiation of plants during the entire process of fruit development. However, the changes in phenolics were often cultivar dependent and they were also influenced by the season and growing conditions [25, 35]. UV-C irradiation increased the level of polyphenols and gene expression of the flavonoid pathway. Several genes, i.e. FaCHS1, FaCHI, FaFHT, FaDFR, FaFLS, and FaFGT, were upregulated at the low (9.6 kJ m–2) and middle (15 kJ m-2) doses while the early stage genes were not affected by the high dose (29.4 kJ m-2) [26]. Considering these investigations, the interaction between different experimental factors, such as UV-C irradiation, harvest time, and growing conditions needs further evaluation.
The major compounds of strawberry fruits include sugars, anthocyanins, amino acids, vitamins, proanthocyanins, flavonols, ellagic acid and derivatives of hydroxycinnamic acids [36–42]. In the previous studies of UV-C irradiated strawberries, the nutrient evaluation was mostly based on several major phenolic compounds such as pelargonidin-3-O-glucoside, pelargonidin-3-O-rutinoside, cyanidin-3-O-glucoside, ellagic acid and quercetin/kaempferol glucosides by targeted analysis [25, 43].
A metabolomic strategy has been used to determine the comprehensive composition of metabolites in strawberries from different genotypes grown under different agronomic conditions [44] or management [45]. To gain a more comprehensive insight of chemical composition of strawberry fruit treated and untreated with UV-C, a non-targeted metabolomic analysis based on ultra-high performance liquid chromatography high resolution mass spectrometry (UHPLC-HRMS) was used to determine differences in the strawberry fruit nutrient content. In metabolomic studies, chemometric approaches such as principal component analysis (PCA) and partial least squares discriminant analysis (PLS-DA) are widely used to understand how different experimental factors affect the occurrence of plant secondary metabolites. However, this approach may be problematic when the variance may come from different sources and may not be directly related to the experimental design, especially when a multiple-factor experimental design is used. To solve this problem, Harrington et al. combined analysis of variance (ANOVA), which is the well-recognized methodology for separation of the different sources of data variation, with PCA [46]. ANOVA–PCA was then employed for differentiation of food or botanicals with different sources of variance such as species [47], cultivar [48], growing conditions [49] and growing locations [50].
It appears that the UV-C irradiation followed by a dark period is becoming adopted by other research groups and may become a mainstream technology in strawberry production, in protective culture of high tunnels and greenhouses as well as in open field production [14, 51–56]. It is imperative that the effects of this treatment on fruit quality are thoroughly investigated. The objective of this study was to determine the effect of the UV-C/dark treatment on changes in the content of major phenolic compounds of short-day ‘Chandler’ strawberries grown in protective culture. In this study, we used an ANOVA-PCA approach on metabolomic data to determine which experimental factor, exposure to UV-C, conditions in growth chamber or harvest time, caused the most variance in the nutrient compounds in strawberry fruit. The application of ANOVA-PCA to metabolomic analyses of strawberry fruit could identify and quantify all the constituents in the fruit and contributes to providing an informative insight of the nutrient value of strawberry fruit.
2Materials and methods
2.1Plants and growth chamber
‘Chandler’ is a short-day strawberry cultivar released in 1984 by the University of California (U.S. Plant Pat. No. 5,262). In October 2018, cold-stored, bare-root transplants of ‘Chandler’ strawberry were obtained from a commercial nursery (Lassen Canyon Nursery, Redding, CA) and established in 16.25 cm (6.5 in) standard TEKU round pots (Pöppelmann GmbH & Co., Lohne, Germany) filled with Sun Gro peat-based growing medium (Sun Gro Horticulture Inc., Agawam, MA).
Strawberries were grown in a glass-glazed greenhouse at Appalachian Fruit Research Station, Kearneysville, WV (lat. 39.4 °N, long. 77.5 °W) with exhaust fan and evaporative-pad cooling, and radiant hot water heating controlled by an environmental control system (Johnson Controls, Cork, Ireland). The greenhouse air temperature set point was a constant 20°C under natural daylengths. Plants were maintained on a greenhouse bench from October 2018 to February 2019. Plants were watered daily and flowers and runners removed at 2-week intervals.
Strawberry plants were removed from the greenhouse bench in February 2019 and placed on wire shelving units (2 shelving units per chamber) in 3 separate growth chambers within the greenhouse. Eight pots were placed along the center of a shelf in the midline of shelving unit on top of inverted TEKU round pots to elevate the plants and prevent fruit from hanging on/through shelves. Open flowers were removed from all plants at the beginning of the experiment.
2.2UV-C/dark treatment
The UV-C irradiation equipment consisted of 8 GermAwayUV disinfection lamps (55Watt UV-C lamps, Philips Model TUV PL-L 55/HF, CureUV, Delray Beach, FL) installed with an electronic brightener reflector surface housing. The lamps were positioned along the side of the plant row, four below and four above plant at a 30 cm distance from plants (Fig. 1). Plants were irradiated for 60 sec at night (11:00 pm) every 3 d, resulting in irradiation dose of 12.36 J m–2 at 253±1 nm for a total dose of 50 J m–2 after integration of the entire peak with the base ranging from 240 to 260 nm. Plants were randomized within each treatment group and shelves were repositioned within chambers weekly. Plants were watered every second day with 100 mL water increasing to 250 mL after 4 weeks. Control (no UV-C treatment) plants were kept on separate wire shelving units in each of the 3 growth chambers. The shelving unit containing control plants in the same chamber as the UV-C apparatus was separated from irradiated plants by black fabric in the late afternoon to block UV-C from control plants during nighttime irradiation. The fabric was removed early in the morning the following day.
Fig. 1
Nighttime UV-C irradiation of strawberry plants with multidirectional positioned lamps to maximize penetration into plant canopy.
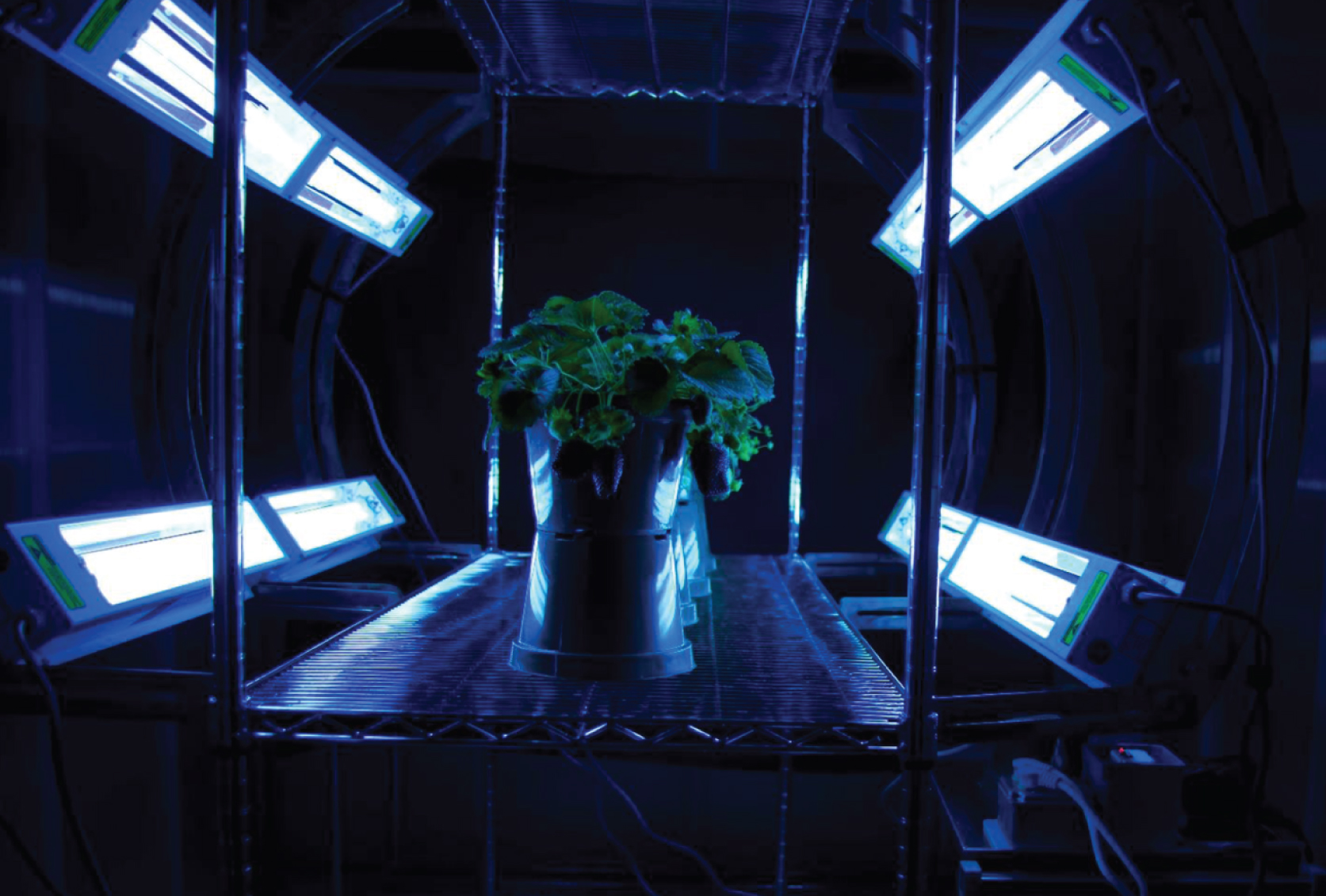
2.3Fruit sampling
Uniformly red-colored strawberry fruit were harvested as they ripened in 3 to 4 d intervals for five weeks. Ripe fruit were cut from the plant leaving approximately 0.7-cm-long pedicel attached, placed in labeled weigh boats and taken to the lab. The pedicel and sepals were removed by hand by grasping all the sepals and stem together and twisting gently. The fruit was sliced to fit and placed in individual 50 mL conical tubes (labeled with treatment ID, fruit number and date) and weighed (wet weight). The flesh in the tubes was flash frozen with liquid nitrogen and placed in a –20°C freezer to allow for evaporation of liquid nitrogen. The samples were lyophilized and stored at –20°C for the analysis.
2.4Freeze-drying samples
The samples were moved from –20°C freezer for freeze-drying. Four tubes per jar were placed on the lyophilizer (Freezemobile 24, The Virtis Company, Gardiner, NY) for 4 d. When samples were dry, the jars were removed, lids were replaced, and dry weight was determined. Tubes were wrapped at lid-tube juncture with Parafilm and stored at –20°C until their transport on ice to USDA-ARS Food Composition and Methods Development Lab in Beltsville, MD for analysis.
2.5Chemicals
HPLC grade methanol, acetonitrile, and formic acid were purchased from Sigma-Aldrich (St. Louis, MO). Pelargonidin-3-O-glucoside, cyanidin-3-O-glucoside, quercetin-3-O-glucoside and kaempferol-3-O-glucoside were purchased from Chromadex (Irvine, CA). Ellagic acid, quercetin, and (+) catechin were purchased from Sigma Aldrich (Irvine, CA).
2.6Sample preparation for chemical composition analysis in strawberry
Two hundred milligrams of each freeze-dried sample was extracted with 5 mL of methanol/water/formic acid; 70:30:1 v/v/v by sonication in an ultrasound bath for 2 h (Branson 3200, Danbury, CT). The slurries were then centrifuged at 5,000 g for 10 min (IEC Clinical Centrifuge, Needham Heights, MA). The upper layer was filtered through a 0.22μm PTFE filter, transferred into a 2 mL HPLC vial, and 2μL were injected for UHPLC-HRMSn analysis.
2.7UHPLC-HRMS conditions
The UHPLC-HRMS (Thermo Scientific, Waltham, MA) system consists of an Orbitrap ID-X tribrid mass spectrometer with a Vanquish UHPLC including a high-pressure binary pump, thermostatting column temperature control compartment, and an HL Diode Array Detector. The separation was carried out on an Agilent Poroshell 120 EC-C18 column (4.6 mm×50 mm, 2.7μm particle size, Agilent, Palo Alto, CA) with a flow rate of 0.6 mL/min. The mobile phases A and B were H2O (0.1% formic acid) and acetonitrile (0.1% formic acid), respectively. The linear gradient was 2% to 50% B (v/v) from 0 to 5 min, to 95% B at 8 min, with a flow rate of 0.6 mL/min. A post-column splitter was used to divert the fluent to diode array detector and mass spectrometer at 1:3 ratio. The re-equilibration time was 2 min. The column temperature was set at 60 °C and the UV/Vis spectra were recorded between 200–700 nm. Accurate mass measurements were carried out under both positive and negative ionization modes. The MS conditions were set as follows: sheath gas at 50 (arbitrary units), auxiliary gas at 10 (arbitrary units), and sweep gas at 1(arbitrary units), spray voltage at 3.5 kV for positive ionization mode and 3 kV for negative ionization mode, ion transfer tube temperature at 325°C, vaporizer temperature at 350°C, RF lens at 60%. The full scan mass range was from 100 to 1000 m/z with a resolution of 60,000, AGC target value of 200,000 in full scan and 10,000 FTMS/MS, and max ion injection time of 50 ms. The most intense ion was selected for the data-dependent scan with normalization collision energy at 30% in HCD. Anthocyanins were monitored at 504 nm and quantified as pelargonidin-3-O-glucoside. Ellagic acids derivatives were quantified as ellagic acid, proanthocyanins were quantified as (+) catechin and flavanols were quantified as quercetin-3-O-glucoside using high resolution selected ion chromatogram (HR-SIM). Data were collected using the Xcalibur 4.1 (Themo Scientific, Waltham, MA).
2.8Statistical analysis
All the Student’s t-tests were performed using Excel 2016 (Microsoft, Seattle, WA). For metabolomic study, raw files were converted using Compound Discoverer 3.0 (Thermo Scientific, Waltham, MA). A data matrix was generated including variable index (paired m/z-retention time), sample names and peak area were exported from Compound Discoverer for further statistical analysis. ANOVA-PCA was performed with in-house scripts compiled in Matlab R2012b (MathWorks Inc., Natick, MA). Principle component analysis (PCA) was performed on the output table using SIMCA 13 (Umetrics, Sartorium Stedim Biotech, Umeå, Sweden).
3Results and discussion
3.1Strawberry metabolites
Overall, 32 metabolites were identified (Table 1) and typical total ion chromatograms from analysis of UV-C treated and untreated strawberries are presented in Fig. 2. The phenolic compounds of ‘Chandler’ strawberry include anthocyanins, proanthocyanindins, flavonols, O-acyl carbohydrates and derivatives of hydroxycinnamic and ellagic acid, which is consistent with previous reports [36–42]. Other compounds such as amino acids and nucleosides were also found. Compounds identification was carried out by comparison between MS data (accurate masses, isotopic distribution, and MS/MS fragmentation behaviors) and the spectra archived in mzCloud [57] in-house databases and previously published MS data for strawberry [36–42].
Fig. 2
Total ion chromatogram of strawberry fruit harvested from control (A) and UV-C treated plants (B).
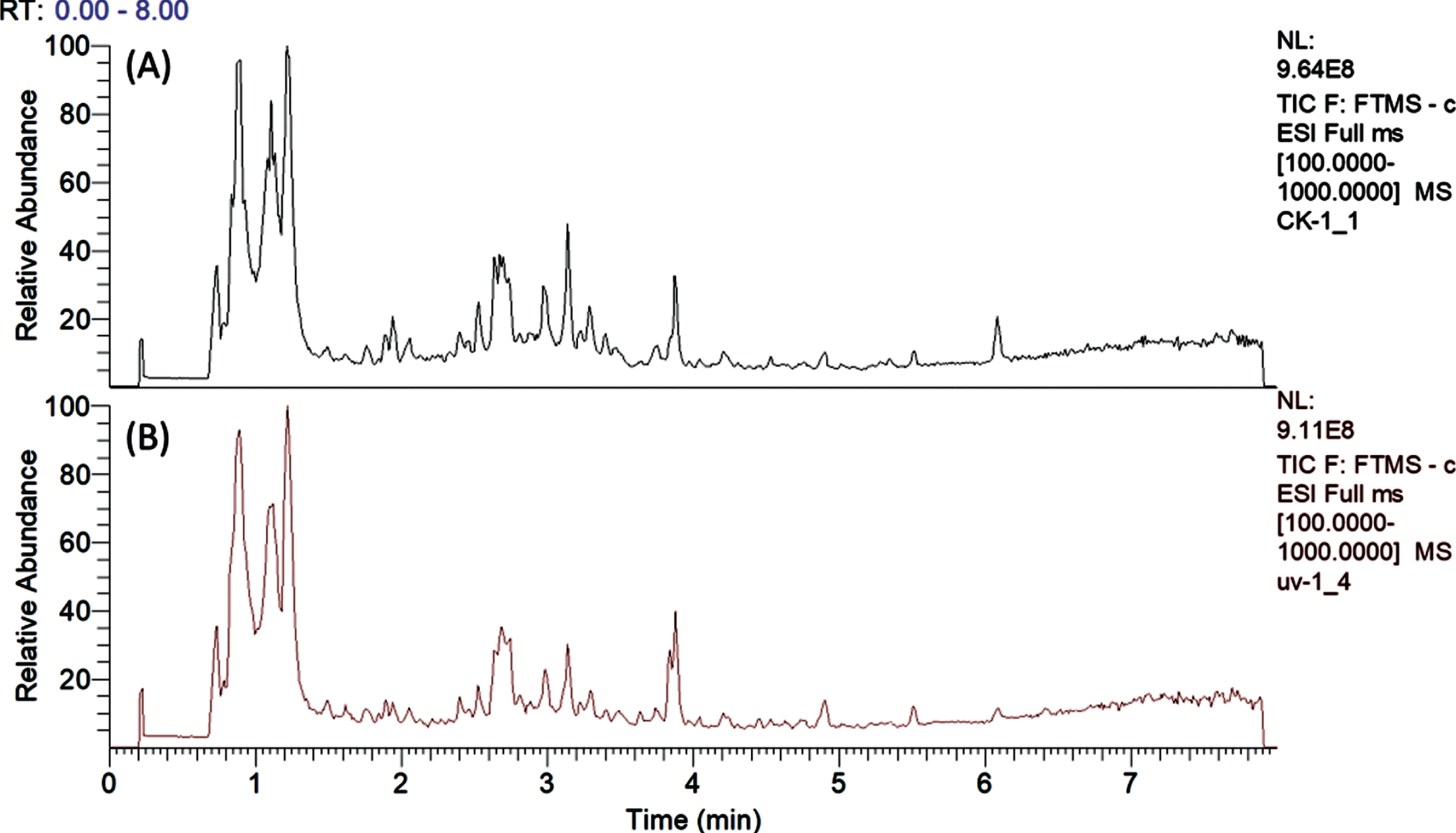
Table 1
Major compounds identified in short day ‘Chandler’ strawberry fruit from plants in growth chambers using UHPLC-HRMS data
Compound name | Formula | Mass to charge ratio (m/z) of [M-H]- or M+,[M + H]+ ions | Rt (min) | Error (ppm) | Major product ions |
Glucose | C6H12O6 | 179.0561 | 0.20 | 0.16 | MS2[179]: 179(100), 161(22), 143(16), 125(11) |
di-Saccharide | C12H22O11 | 341.1091 | 0.88 | 0.57 | MS2[341]: 341(15), 179(41), 161(19), 149(12), 143(14), 131(16), 119(100), 113(71), 101(80) |
Ascorbic acid | C6H8O6 | 175.0249 | 0.96 | 0.28 | MS2[175]: 175(14), 157(100), 131(13), 127(9), 117(12), 115(71), 113(41) |
Citric acid or Isocitric acid | C6H8O7 | 191.0196 | 1.10 | –0.71 | MS2[191]: 191(14), 111(100) |
Glutathione | C10H17O6N3S | 308.0917* | 1.21 | 2.00 | MS2[308]: 245(10), 233(15), 215(8), 187(8), 179(32), 170(10), 162(100), 144(38), 142(20), 130(39), 116(37) |
Aconitic acid | C6H6O6 | 173.0092 | 1.28 | 0.23 | MS2[173]: 129(10), 111(100) |
Adenosine | C10H12N5O4 | 268.1049* | 1.63 | 3.20 | MS2[268]: 136(100) |
Procyaninin C1 | C45H38O18 | 865.1991 | 1.93 | 0.65 | MS2[865]: 695(40), 577(32), 451(35), 425(31), 407(73), 405(32), 289(69), 287(100), 243(36), 125(59) |
bis-HHDP-glucose | C24H24O22 | 783.0698 | 2.05 | 1.42 | MS2[783]: 783(25), 481(11), 301(100), 275(36) |
Proanthocyanidin B1 | C30H26O12 | 577.1356 | 2.40 | 0.71 | MS2[577]: 451(6), 425(8), 407(83), 339(7), 289(100), 287(6), 273(6), 245(17), 161(12), 125(37) |
DL-Tryptophan | C11H12O2N2 | 203.0825 | 2.42 | 0.35 | MS2[203]: 203(17), 159(9), 142(36), 116(100) |
Cyanidin-3-O-glucoside | C21H21O11 | 449.1090** | 2.53 | 2.78 | MS2[449]: 287(100) |
HHDP-galloylglucoside | C27H22O18 | 633.0733 | 2.53 | –0.58 | MS2[633]: 633(40), 463(11), 301(100), 275(7) |
(+) Catechin | C15H14O6 | 289.0713 | 2.63 | –0.49 | MS2[289]: 289(67), 245(100), 221(25), 205(53), 203(81), 187(21), 179(32), 165(21), 161(22), 151(38), 137(30), 125(55), 123(26), 109(53) |
Pelargonindin-3-O-glucoside | C21H21O10 | 433.1137** | 2.67 | 2.02 | MS2[433]: 271(100) |
Coumaroyl-glucose | C15H18O8 | 325.0927 | 2.74 | –0.65 | MS2[325]: 163(8), 145(100) |
2-Isopropylmalic acid | C7H12O5 | 175.0612 | 2.81 | 0.92 | MS2[175]: 175(8), 115(100) |
Ellagic acid glucose | C20H16O13 | 463.0521 | 2.87 | 0.60 | MS2[463]: 463(67), 301(100), 300(21), 283(7), 271(10) |
Pelargonindin-3-O-rutinoside | C30H27O12 | 579.1512** | 2.93 | 2.56 | MS2[579]: 271(100) |
Ellagic acid | C14H6O8 | 300.9986 | 2.99 | –1.30 | MS2[301]: 301(100),284(2), 257(2), 229(2), 201(13), 185(2) |
Agrimoniin | C82H52O54 | 934.0726*** | 3.13 | –0.42 | MS2[934]: 935(37), 934(7), 897(29), 783(17), 633(15), 613(10), 301(100) |
Ellagic acid pentose | C20H16O12 | 447.0573 | 3.22 | –0.85 | MS2[447]: 447(76), 301(100), 300(73) |
Galloyl-glucose | C17H16O7 | 331.0825 | 3.25 | 0.53 | MS2[331]: 331(22), 271(7), 211(5), 169(100), 168(5), 161(3), 125(8) |
Pelargonidin-3-O-acetylglucoside | C23H23O11 | 475.1248** | 3.30 | 2.68 | MS2[475]: 475(3), 271(100) |
Quercetin-3-O-glucuronide | C21H18O13 | 477.0679 | 3.41 | 0.92 | MS2[477]: 477(2), 301(100), 179(2), 151(2) |
Quercetin-3-O-glucoside | C21H20O12 | 463.0886 | 3.49 | 0.71 | MS2[463]: 463(30), 301(63), 300(100), 179(2) |
Kaempferol-3-O-glucuronide | C21H18O12 | 461.0728 | 3.76 | 0.50 | MS2[461]: 461(2), 285(100), 257(2), 113(7) |
Kaempferol-3-O-glucoside | C21H20O11 | 447.0934 | 3.86 | 0.21 | MS2[447]: 447(81), 327(4), 285(53), 284(100), 256(2), 255(4), 151(3) |
Feruloyl-glucose | C16H20O9 | 355.1032 | 3.87 | –0.61 | MS2[355]: 161(2), 147(100) |
Syringin | C17H24O9 | 371.1348 | 4.21 | 0.04 | MS2[417]: 251(2), 209(100) |
(±)-Abscisic acid | C15H20O4 | 263.1290 | 4.74 | 0.33 | MS2[263]: 219(65), 204(59), 201(27), 163(13), 153(100), 152(12), 151(11), 138(13) |
Methylsyringin | C18H26O9 | 385.1704 | 4.87 | –0.12 | MS2[309]: 309(13), 225(19), 205(100) |
*[M + H]+, ** M+, ***[M-2H]2 - Compounds written in bold font were confirmed with reference standards.
Four major anthocyanins were found in all samples (cyanidin-3-O-glucoside, pelargonidin-3-O-glucoside, pelargonidin-3-O-rutinoside and pelargonidin-3-O-acetylgluoside) with monitor set at 504 nm (Fig. 3A). MS/MS product ion spectra of these 4 anthocyanins are presented in Fig. 3B. At peak 1, with M+ at m/z 449.1088 (C21H21O11, –1.12 ppm), loss of one hexosyl group (C6H10O5) was observed. The major product ion at m/z 287.0551 (C15H11O6, 0.12 ppm) suggested the anthocyanin aglycone is cyanidin. This peak was identified as cyanidin-3-O-glucoside (C3G) and confirmed with a reference standard. Peak 2 was the major anthocyanin peak with M+ at m/z 433.1135 (C21H21O10, 2.09 ppm) and m/z 271.0821 (C21H15O7, 3.07 ppm) is the major product ion, this peak was identified and confirmed as pelargonidin-3-O-glucoside (P3G). Similarly, Peak 3 was identified as pelargonidin-3-O-rutinoside (P3R, m/z 579.1719, C27H31O14, 1.84 ppm). Peak 4 with M+ at m/z 475.1248 (C23H23O11, –2.77 ppm) and the major product ion at m/z 271 was produced via the loss of an acetyl and a hexose moiety, thus this peak was identified as pelargonidin-3-O-acetyl glucoside (P3AG).
Typical flavonols were found to be quercetin-3-O-glucoside (Q3G), kaempferol-3-O-glucoside (K3G), quercetin-3-O-glucuronide (Q3GR) and kaempferol-3-O-glucurondie (Q3GR). For these compounds, the typical MS2 product ion at m/z 301 and m/z 285 were used to confirm the flavonol glycosides with quercetin and kaempferol aglycone, respectively. The Q3G and K3G were also confirmed by reference standards. Ellagic acid (EA) and its derivatives were another major kind of phenolic compound found in both UV-C treated and control groups of strawberry. A diagnostic product ion at m/z 300.9987(C14H5O8), corresponding to deprotonated form of ellagic acid, was used as diagnostic ion to confirm ellagic acid hexoside and pentoside. It is worth noting that ellagic acid glucoside and quercetin glucoside in strawberry share the same nominal mass of m/z 463 in full scan and product ion of m/z 301 in the MS/MS spectra under negative ionization mode. They might be mis-identified as one another if only using a nominal mass spectrometer because of its insufficient resolving power. However, as shown in Fig. 4, these two compounds were easily differentiated using a high-resolution mass spectrometer. Ellagic acid glucoside has an elemental composition of C20H16O13 ([M-H]-, 463.0515, –0.591 ppm) while quercetin has an elemental composition of C21H20O12 ([M-H]-, 463,0883, 0.196 ppm) in the full scan. Though these two compounds exhibit very similar fragment behavior with the neutral loss of a glucosyl unit, the m/z 151 and m/z 179 were only observed in quercetin-3-O-glucoside, in addition to the elemental composition differences of the two aglycone ions. This strategy can be also used for the differentiation of kaempferol glucoside and ellagic acid pentoside.
Fig. 3
The HPLC chromatogram (504 nm) of four major anthocyanins: 1 = C3G, 2 = P3G, 3 = P3R, and 4 = P3AG (A), and MS/MS spectra of C3G, P3G, P3R, and P3AG (B). C3G = cyanidin-3-O-glucoside, P3G = pelargonidin-3-O-glucoside, P3R = pelargonidin-3-O-rutinoside, and P3AG = pelargonidin-3-O-acetylgluoside.
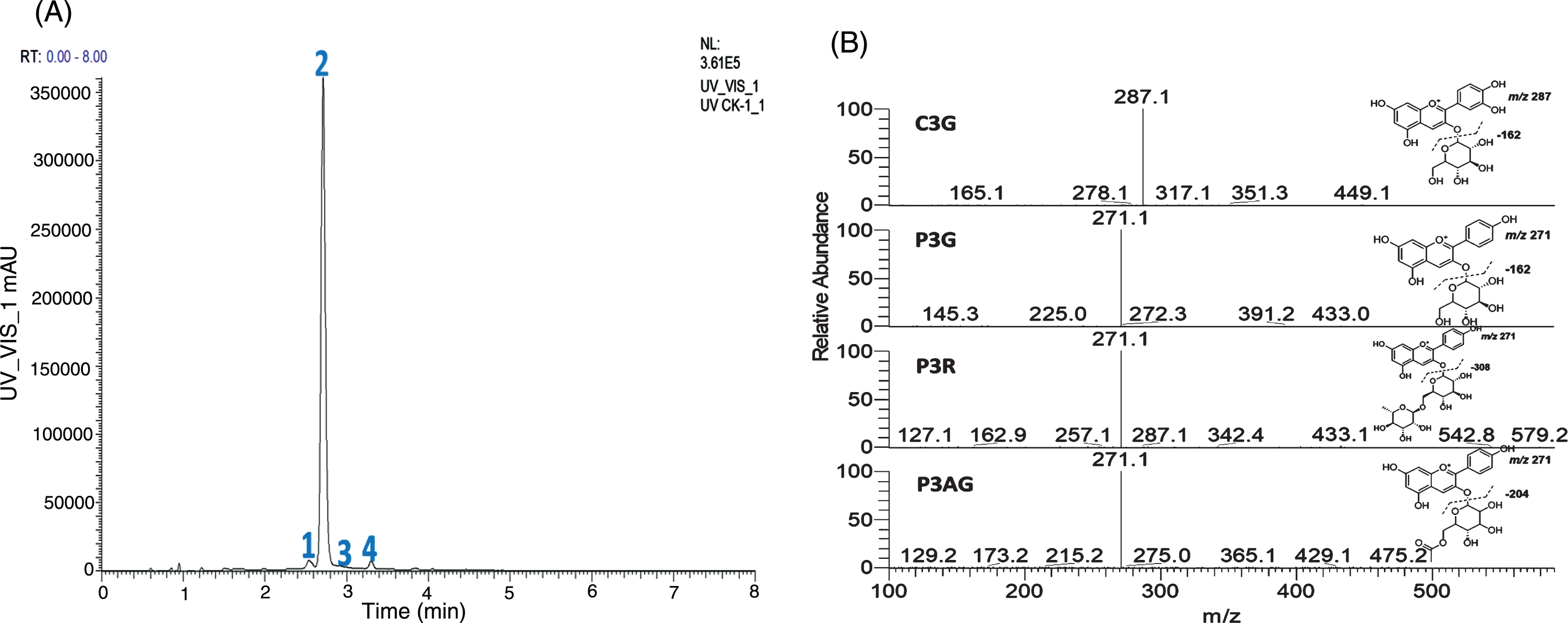
Fig. 4
High-resolution mass spectra (MS2) of quercetin-3-O-glucoside (A) and ellagic acid glucoside (B).
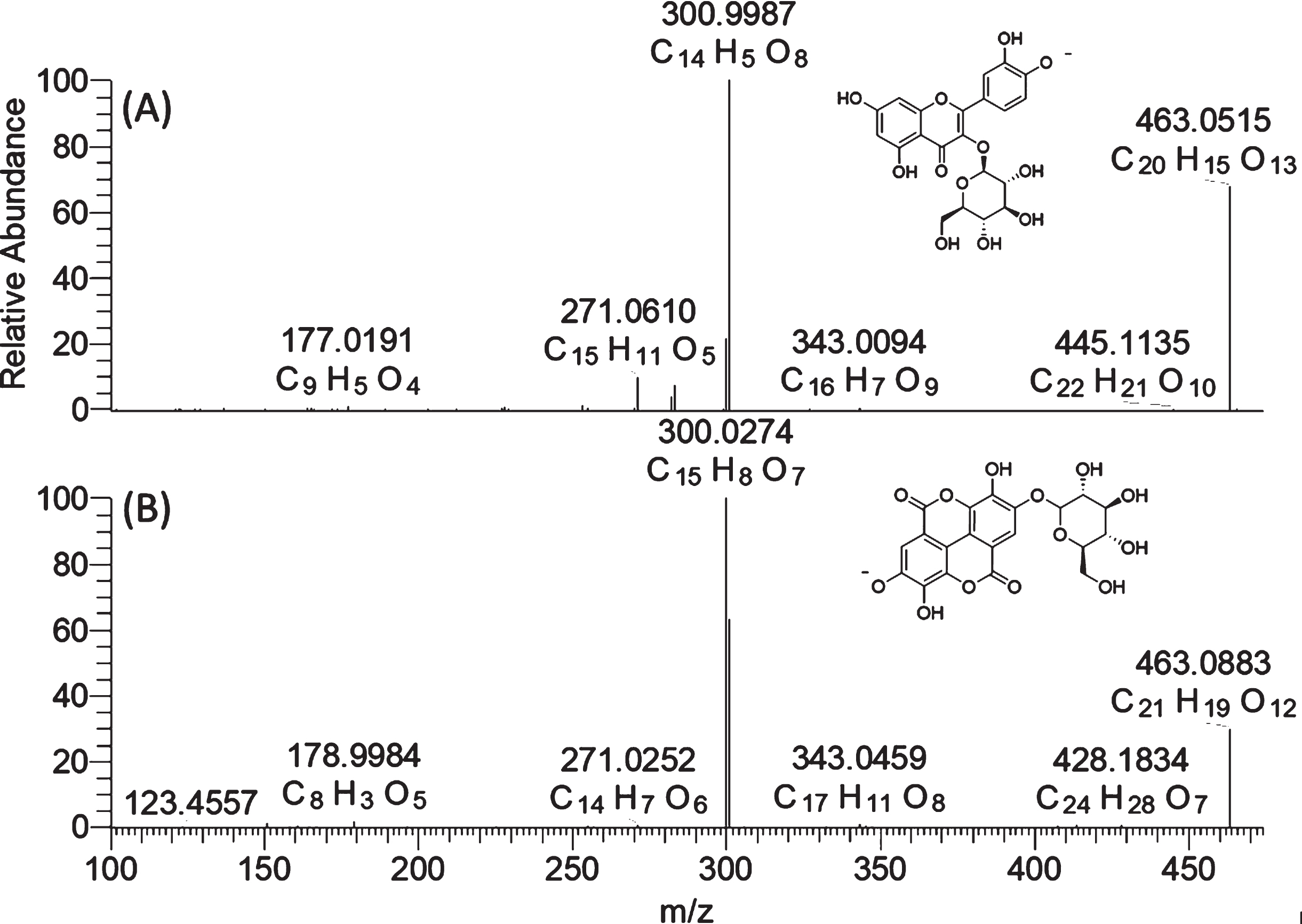
3.2Effect of UV-C on anthocyanins and other phenolic compounds
Anthocyanins concentrations were quantified based on the P3G content using the HPLC-UV peak area under 504 nm, and P3G was found to be the most abundant anthocyanin in ‘Chandler’ strawberry and was about 40, 60 and 140 times more abundant than C3G, P3AG and P3R, respectively, in both UV-C treated and not treated strawberries. The levels from irradiated strawberry did not differ from the non-irradiated control group at all three harvest dates. The individual anthocyanin concentrations were not significantly (P > 0.05) influenced by UV-C treatments, except for a minor anthocyanin, C3G, which was slightly different (0.41 vs 0.50μmol/g dry weight) in the fruit from the first harvest (Table 2).
Table 2
Quantitation of individual and total anthocyanins in short day ‘Chandler’ strawberry fruit harvested at three different times from plants that were treated or not treated (Control) with UV-C
Harvest | Treatment | C3G | P3G | P3R | P3AG | Total Anthocyanins |
1 | UV-C (n = 20) | 0.41±0.03* | 20.70±5.79 | 0.14±0.03 | 0.29±0.11 | 21.54±6.01 |
03/28/19 | Control (n = 23) | 0.50±0.11 | 19.89±5.00 | 0.14±0.03 | 0.30±0.10 | 20.83±5.19 |
2 | UV-C (n = 22) | 0.45±0.11 | 21.16±3.44 | 0.17±0.03 | 0.32±0.07 | 22.10±3.57 |
04/01/19 | Control (n = 22) | 0.45±0.13 | 19.87±4.49 | 0.17±0.04 | 0.32±0.07 | 20.81±4.65 |
3 | UV-C (n = 19) | 0.47±0.13 | 22.41±4.20 | 0.15±0.03 | 0.31±0.06 | 23.30±4.35 |
04/04/19 | Control (n = 20) | 0.52±0.11 | 20.25±5.11 | 0.14±0.04 | 0.28±0.08 | 21.19±5.26 |
Concentrations are expressed as mean±standard deviation in μmol g–1 dry weight (DW); * indicates significant differences (P < 0.05) between the UV-C and control group with the same harvest time by Student’s t-test. C3G = cyanidin-3-O-glucoside, P3G = pelargonidin-3-O-glucoside, P3R = pelargonidin-3-O-rutinoside, and P3AG = pelargonidin-3-O-acetylhexoside.
Besides anthocyanidins, we found that the UV-C irradiation did not cause significant changes in individual phenolic compounds including ellagic acid (EA), (+) catechin (C), quercetin 3-O-glucoside (Q3G), quercetin 3-O-glucuronide (Q3GR), kaempferol-3-O-glucoside (K3R), and kaempferol 3-O-glucuronide (K3GR). The quantitative analysis showed that in ‘Chandler’ strawberry C and EA were two major phenolic compounds (Table 3). The concentration of glucuronides of quercetin and kaempferol were 5 to 15 times higher than the related glucosides in both groups.
Table 3
Content of phenolic compounds in strawberries from different harvest dates from plants that were treated or not treated (Control) with UV-C
Harvest | Treatment | C | EA | Q3G | Q3GR | K3G | K3GR |
1 | UV-C (n = 20) | 1313.5±197.3 | 579.0±200.4 | 49.0±18.4 | 257.5±22.4 | 7.0±2.5 | 102.2±21.7 |
03/28/19 | Control (n = 23) | 1355.1±17.9 | 687.0±175.2 | 52.8±14.9 | 246.1±30.3 | 7.0±2.6 | 114.4±23.7 |
2 | UV-C (n = 22) | 1313.6±432.2 | 598.9±303.7 | 49.2±16.0 | 259.7±26.5 | 8.5±2.3 | 65.5±40.8 |
04/01/19 | Control (n = 22) | 1152.6±404.8 | 622.2±256.0 | 48.3±15.1 | 241.1±36.5 | 7.7±2.7 | 85.0±40.9 |
3 | UV-C (n = 19) | 1340.2±445.2 | 495.6±204.5 | 37.6±13.9 | 288.8±70.8 | 8.3±3.2 | 43.5±43.2 |
04/04/19 | Control (n = 20) | 1405.1±217.6 | 527.4±201.4 | 40.3±14.2 | 271.3±31.8 | 10.0±4.1 | 55.5±38.6 |
Concentrations are expressed as mean±standard deviation in nmol g–1 dry weight (DW). C = (+) catechin, EA = ellagic acid, Q3G = quercetin-3-O-glucoside, Q3GR = quercetin-3-O-glucuronide, K3GR = kaempferol-3-O-glucuronide, and K3G = kaempferol-3-O-glucoside.
3.3Principal component analysis and ANOVA-PCA of metabolomic data
The original UHPLC-HRMS raw data were deconvoluted using Compound Discoverer 3.0 (Thermo Scientific, Waltham, MA) and it led to the extraction of 2,617 ion features (m/z, retention time, intensity) and 2,019 ion features under positive and negative ionization modes, respectively, from the 126 fruit samples analyzed. First PCA was used on these two datasets and we established that the variance found may not be attributed to UV-C treatment, which was consistent with our targeted analysis (Fig. 5). The PC1/PC2 score plot shows that samples from first harvest were clustered together. Samples from the second and third harvests formed a separate cluster; however, no separation was observed between UV-C treated and untreated (control) groups using both positive and negative ionization data. This suggests that other environmental factors, such as light intensity, day length and daily light interval influenced the composition of the metabolites in this short-day strawberry cultivar. This agrees with recent reports that found that seasonal and growing conditions can affect the composition of strawberry metabolites rather than the pre-harvest treatment with UV-C [25, 35]. The non-targeted metabolomics analysis can provide a complimentary comparison of treated and not treated strawberry based on all metabolites detected by UHPLC-HRMS method. It can determine any potential differences between two groups of strawberry, especially for possible minor metabolites changes. As all the metabolite information from the raw data was used in non-targeted metabolomic study, it provided a more comprehensive picture than using only major metaboloites identified in the targeted analysis.
Fig. 5
PCA score plot of 126 strawberry samples from three harvests (Hvst1-3) using positive ionization (A) and negative ionization data (B). Blue triangles represent samples from strawberries treated with UV-C and red triangles are from the control (CK) samples.
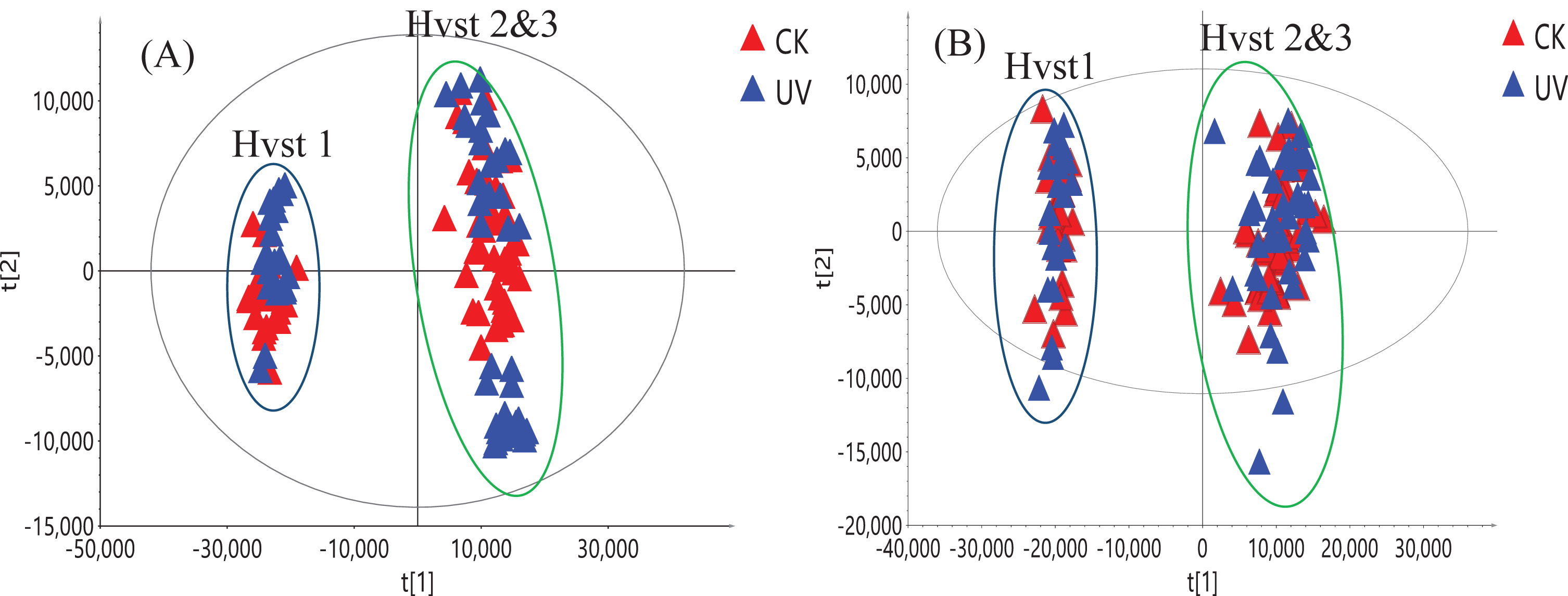
As all experimental factors may contribute to variability, other factors such as growth chamber and harvest time also may have an effect in addition to UV-C treatment. ANOVA-PCA was subsequently used to isolate and identify the contribution of each experimental factor. The raw data were deconvoluted into 8 sub-matrices; means and residuals for irradiation (I), growth chamber (G), harvest time (T), plus factor interaction (I×G×T) and the factor interaction residual matrix (FIres). The ANOVA data computed for preprocessed data showed that iradiation (I), harvest time (T), and growth chamber (G) accounted for 2.5, 25.7 and 8.7% of the total variance, respectively, for positive ionization data. For negative ionization data, these three experimental factors accounted for 1.0, 48.7 and 1.9% of the total variance, respectively. The separation was not observed on the score plots of UV-C irradiation mean matrix plus the factor interaction residual matrix (I + FIres, Fig. 6A and D) and growth chamber mean matrix plus interaction residual matrix (G + FIres, Fig. 6C and F). However, obvious separation was observed for the harvest time mean plus interaction residual matrix (T + FIres, Fig. 6B and E) for both positive and negative ionization data. ANOVA-PCA confirmed that the highest experimental variance was explained by harvest time, while UV-C irradiation did not affect the kind and concentration of secondary metabolites.
Fig. 6
Score plot from ANOVA-PCA on metabolomic data based on different experimental factors: Irradiation factor (A, D), harvest time factor (B, E) and growth chamber factor (C, F) using positive (A, B, C) and negative (D, E, F) ionization metabolomic data sets.
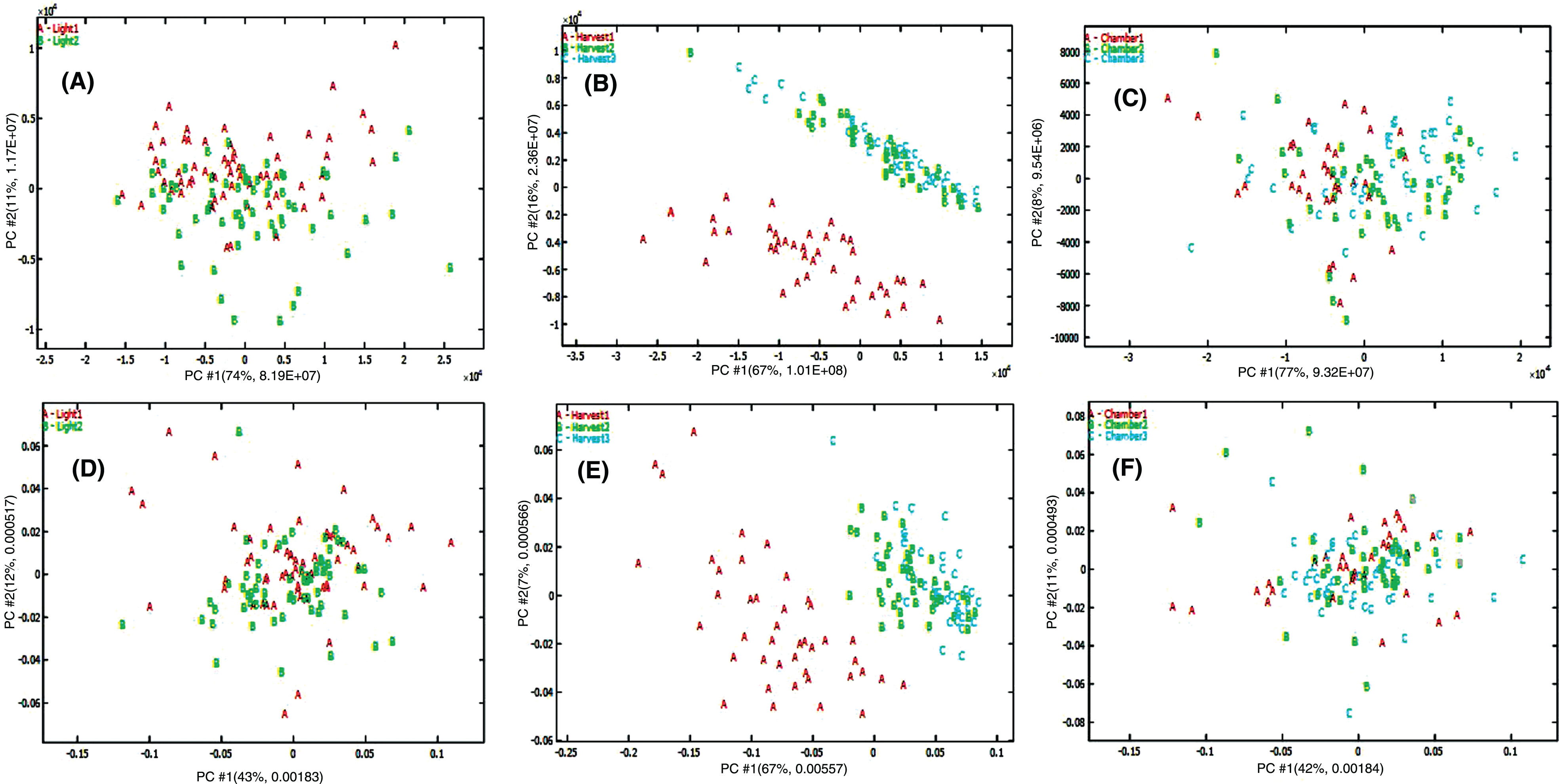
4Conclusions
UV-C treatment is considered an environmentally friendly approach for controlling undesirable microbes including plant pathogens. In order to make UV-C technology acceptable for commercial strawberry production, its effect on fruit quality, especially on compounds of nutraceutical value, needs to be examined. In the current work, the effects of preharvest UV-C irradiation on the composition and content of major phenolic compounds of strawberries was determined. No deleterious effects on the secondary metabolites of strawberry fruit were detected after UV-C irradiation of plants during the entire production cycle. Neither the kind nor the level of anthocyanins, glucosides and glucuronides of quercetin and kaempferol, catechin, or ellagic acid were affected by UV-C irradiation, as demonstrated by targeted quantitative analysis and non-targeted metabolomic analysis. For the first time, metabolomic approach combined with ANOVA-PCA was used to evaluate strawberry fruit quality after repeated UV-C irradiation of plants during the entire production cycle. We demonstrated that this approach is very powerful in providing an overall insight into fruit quality and pinpoints the influence of each experimental factor.
Funding
The authors report no funding.
Conflict of interest
The authors have no conflict of interest to report.
Acknowledgments
This research was supported by the Agricultural Research Service of the U.S. Department of Agriculture (USDA) and an Interagency Agreement with the Office of Dietary Supplements (ODS) of the National Institutes of Health (NIH).
References
[1] | Statista. Most consumed fruits in the United States in 2018. Available from: www.statista.com/statistics/477475/us-most-consumed-fruit-and-fruit-products-by-type/ |
[2] | Hannum SM . Potential impact of strawberries on human health: a review of the science. Crit Rev Food Sci Nutr. (2004) ;44: (1):1–17. doi:10.1080/10408690490263756 |
[3] | Samtani JB , Rom CR , Friedrich H , Fennimore SA , Finn CE , Petran A et al. The status and future of the strawberry industry in the United States. Horttechnology. (2019) ;29: (1):11–24. doi:10.21273/Horttech04135-18 |
[4] | Pokorny A , Smilanick JL , Xiao CL , Forrar JJ , Shrestha A . Determination of fungicide resistance in Botrytis cinerea from strawberry in the central costal region of California. Plant Health Progress. (2016) ;17: (3):30–4. doi:10.1094/PHP-RS-15-0053 |
[5] | Oliveira MS , Amiri A , zuniga AI , Peres NA . Sources of primary inoculum of Botrytis cinerea and their impact on fungicide resistance development in commercial strawberry fields. Plant Dis. (2017) ;101: (10):1769–73. doi:10.1094/PDIS-02-17-0203-RE |
[6] | Bulger MA , Ellis MA , Madden LV . Influence of temperature and wetness duration on infection of strawberry flowers by Botrytis cinerea and disease incidence of fruit originating from infected flowers. Phytopathology. (1987) ;77: (8):1225–30. doi:Doi 10.1094/Phyto-77-1225 |
[7] | Nigro F , Ippolito A , Lattanzio V , Di Venere D , Salerno M . Effect of ultraviolet-C light on postharvest decay of strawberry. J Plant Pathol. (2000) ;82: :29–37. |
[8] | Marquenie D , Michiels CW , Geeraerd AH , Schenk A , Soontjen C , Van Impe JF et al. Using survival analysis to investigate the effect of UV-C and heat treatment on storage rot of strawberry and sweet cherry. Int J Food Microbiol. (2002) ;73: (2-3):187–96. doi:10.1016/s0168-1605(01)00648-1 |
[9] | Marquenie D , Lammertyn J , Geeraerd AH , Soontjens C , Van Impe JF , Nicolai BM et al. Inactivation of conidia of Botrytis cinerea and Monilinia fructigena using UV-C and heat treatment. Int J Food Microbiol. (2002) ;74: (1-2):27–35. doi:10.1016/s0168-1605(01)00719-x |
[10] | Lammertyn J , De Ketelaere B , Marquenie D , Molenberghs G , Nicolai BM . Mixed models for multicategorical repeated response: modelling the time effect of physical treatments on strawberry sepal quality. Postharvest Biol Tec. (2003) ;30: (2):195–207. |
[11] | Marquenie D , Michiels CW , Van Impe JF , Schrevens E , Nicolai BN . Pulsed white light in combination with UV-C and heat to reduce storage rot of strawberry. Postharvest Biol Tec. (2003) ;28: (3):455–61. |
[12] | Janisiewicz WJ , Takeda F , Nichols B , Glenn DM , Jurick WM , Camp MJ . Use of low-dose UV-C irradiation to control powdery mildew caused by Podosphaera aphanis on strawberry plants. Canadian J. Plant Pathol. (2016) ;38: (4):430–9. |
[13] | Janisiewicz WJ , Takeda F , Glenn DM , Camp MJ , Jurick WM . Dark period following UV-C treatment enhances killing of Botrytis cinerea conidia and controls gray mold of strawberries. Phytopathology. (2016) ;106: (4):386–94. |
[14] | Short BD , Janisiewicz W , Takeda F , Leskey TC . UV-C irradiation as a management tool for Tetranychus urticae on strawberries. Pest Management Science. (2018) ;74: (11):2419–23. |
[15] | Hamauzu Y . Role and evolution of fruit phenolic compounds during ripening. Stewart Postharvest Review. (2006) ;2: (2):1–7. doi:10.2212/spr.2006.2.5 |
[16] | Wang J , Lin Q , Li J , Feng C , Yang C , Rong R . Effects of chitosan and ultraviolet-C treatments on storage quality of winter jujube. Shipin Kexue (Beijing, China). (2010) ;31: (22):462–6. |
[17] | Lee H-J , Chun J-H , Kim S-J . Effects of pre harvest light treatments (LEDs, fluorescent lamp, UV-C) on glucosinolate contents in rocket salad (Eruca sativa). Weon’ye Gwahag Gi’sulji. (2017) ;35: (2):178–87. doi:10.12972/kjhst.20170021 |
[18] | Vasquez H , Ouhibi C , Lizzi Y , Azzouz N , Forges M , Bardin M et al. Pre-harvest hormetic doses of UV-C radiation can decrease susceptibility of lettuce leaves (Lactuca sativa L.) to Botrytis cinerea L. Sci Hortic (Amsterdam, Neth). (2017) ;222: :32–9. |
[19] | Martinez-Sanchez A , Guirao-Martinez J , Martinez JA , Lozano-Pastor P , Aguayo E . Inducing fungal resistance of spinach treated with preharvest hormetic doses of UV-C. LWT–Food Sci Technol. (2019) ;113: :108302. doi:10.1016/j.lwt.2019.108302 |
[20] | Guerrero RF , Cantos-Villar E , Puertas B , Richard T , Richard T . Daily Preharvest UV-C light maintains the high stilbenoid concentration in grapes. J Agric Food Chem. (2016) ;64: (25):5139–47. |
[21] | Romanazzi G , Gabler FM , Smilanick JL . Preharvest chitosan and postharvest UV irradiation treatments suppress gray mold of table grapes. Plant Dis. (2006) ;90: (4):445–50. doi:10.1094/PD-90-0445 |
[22] | Cantos E , Espin JC , Tomas-Barberan FA . Postharvest induction modeling method using UV irradiation pulses for obtaining resveratrol-enriched table grapes: a new “functional” fruit? J Agric Food Chem. (2001) ;49: (10):5052–8. doi:10.1021/jf010366a |
[23] | Imaizumi T , Yamauchi M , Sekiya M , Shimonishi Y , Tanaka F . Responses of phytonutrients and tissue condition in persimmon and cucumber to postharvest UV-C irradiation. Postharvest Biol Tec. (2018) ;145: :33–40. doi:10.1016/j.postharvbio.2018.06.003 |
[24] | Xu Y , Charles MT , Luo Z , Roussel D , Rolland D . Potential link between fruit yield, quality parameters and phytohormonal changes in preharvest UV-C treated strawberry. Plant Physiol Biochem. (2017) ;116: :80–90. doi:10.1016/j.plaphy.2017.05.010 |
[25] | Xu Y , Luo Z , Xu Y , Charles MT , Mimee B , Veronneau P-Y et al. Preharvest ultraviolet C irradiation increased the level of polyphenol accumulation and flavonoid pathway gene expression in strawberry fruit. J Agric Food Chem. (2017) ;65: (46):9970–9. |
[26] | Xu Y , Luo Z , Tong Z , Xu Y , Charles MT , Mimee B , et al . Preharvest ultraviolet C treatment affected senescence of stored strawberry fruit with a potential role of microRNAs in the activation of the antioxidant system. J Agric Food Chem. (2018) ;66: (46):12188–97. |
[27] | Xie Z , Fan J , Charles MT , Charlebois D , Khanizadeh S , Rolland D et al. Preharvest ultraviolet-C irradiation: Influence on physicochemical parameters associated with strawberry fruit quality. Plant Physiol Biochem. (2016) ;108: :337–43. doi:10.1016/j.plaphy.2016.07.026 |
[28] | Xu Y , Luo Z , Charles MT , Rolland D , Roussel D . Pre-harvest UV-C irradiation triggers VOCs accumulation with alteration of antioxidant enzymes and phytohormones in strawberry leaves. J Plant Physiol. (2017) ;218: :265–74. |
[29] | Severo J , de Oliveira IR , Bott R , Le Bourvellec C , Renard CMGC , Page D et al. Preharvest UV-C radiation impacts strawberry metabolite content and volatile organic compound production. LWT–Food Sci Technol. (2017) ;85: (Part_B):390–3. doi:10.1016/j.lwt.2016.10.032 |
[30] | Xu Y , Charles MT , Luo Z , Mimee B , Tong Z , Roussel D et al. Preharvest UV-C treatment affected postharvest senescence and phytochemicals alternation of strawberry fruit with the possible involvement of abscisic acid regulation. Food Chem. (2019) ;299: :125138. doi:10.1016/j.foodchem.2019.125138 |
[31] | Li DD , Luo ZS , Mou WS , Wang YS , Ying TJ , Mao LC . ABA and UV-C effects on quality, antioxidant capacity and anthocyanin contents of strawberry fruit (Fragaria ananassa Duch.). Postharvest Biol Tec. (2014) ;90: :56–62. |
[32] | Xie Z , Charles MT , Charlebois D , Rolland D , Roussell D , Deschênes M et al. Preharvest exposure to UV-C radiation: impact on strawberry fruit quality. Acta Hort. (2015) ;1079: :589–92. |
[33] | Pineli LDD , Moretti CL , dos Santos MS , Campos AB , Brasileiro AV , Cordova AC et al. Antioxidants and other chemical and physical characteristics of two strawberry cultivars at different ripeness stages. J Food Compos Anal. (2011) ;24: (1):11–6. |
[34] | Williner MR , Pirovani ME , Guemes DR . Ellagic acid content in strawberries of different cultivars and ripening stages. J Sci Food Agr. (2003) ;83: (8):842–5. doi:10.1002/jsfa.1422 |
[35] | Xie Z , Charles MT , Fan J , Charlebois D , Khanizadeh S , Rolland D et al. Effects of preharvest ultraviolet-C irradiation on fruit phytochemical profiles and antioxidant capacity in three strawberry (Fragaria×ananassa Duch.) cultivars. J Sci Food Agric. (2015) ;95: (14):2996–3002. doi:10.1002/jsfa.7064 |
[36] | Maatta-Riihinen KR , Kamal-Eldin A , Torronen AR . Identification and quantification of phenolic compounds in berries of Fragaria and Rubus species (family Rosaceae). J Agric Food Chem. (2004) ;52: (20):6178–87. doi:10.1021/jf049450r |
[37] | Buendia B , Gil MI , Tudela JA , Gady AL , Medina JJ , Soria C et al. HPLC-MS analysis of proanthocyanidin oligomers and other phenolics in 15 strawberry cultivars. J Agric Food Chem. (2010) ;58: (7):3916–26. doi:10.1021/jf9030597 |
[38] | Hilt P , Schieber A , Yildirim C , Arnold G , Klaiber I , Conrad J et al. Detection of phloridzin in strawberries (Fragaria x ananassa Duch.) by HPLC-PDA-MS/MS and NMR spectroscopy. J Agric Food Chem. (2003) ;51: (10):2896–9. doi:10.1021/jf021115k |
[39] | Vrhovsek U , Guella G , Gasperotti M , Pojer E , Zancato M , Mattivi F . Clarifying the identity of the main ellagitannin in the fruit of the strawberry, Fragaria vesca and Fragaria ananassa Duch. J Agric Food Chem. (2012) ;60: (10):2507–16. doi:10.1021/jf2052256 |
[40] | Kelebek H , Selli S . Characterization of phenolic compounds in strawberry fruits by Rp-Hplc-Dad and investigation of their antioxidant capacity. J Liq Chromatogr R T. (2011) ;34: (20):2495–504. doi:Doi 10.1080/10826076.2011.591029 |
[41] | Aaby K , Ekeberg D , Skrede G . Characterization of phenolic compounds in strawberry (Fragaria x ananassa) fruits by different HPLC detectors and contribution of individual compounds to total antioxidant capacity. J Agric Food Chem. (2007) ;55: (11):4395–406. doi:10.1021/jf0702592 |
[42] | Sun J , Liu X , Yang T , Slovin J , Chen P . Profiling polyphenols of two diploid strawberry (Fragaria vesca) inbred lines using UHPLC-HRMS(n.). Food Chem. (2014) ;146: :289–98. doi:10.1016/j.foodchem.2013.08.089 |
[43] | Xie Z , Fan J , Charles MT , Charlebois D , Rolland D , Roussel D et al. Preharvest ultraviolet-C irradiation: Influence on physicochemical parameters associated with strawberry fruit quality. Plant Physiol Biochem. (2016) ;108: :337–43. |
[44] | Akhatou I , Sayago A , Gonzalez-Dominguez R , Fernandez-Recamales A . Application of targeted metabolomics to investigate optimum growing conditions to enhance bioactive content of strawberry. J Agric Food Chem. (2017) ;65: (43):9559–67. doi:10.1021/acs.jafc.7b03701 |
[45] | Akhatou I , Gonzalez-Dominguez R , Fernandez-Recamales A . Investigation of the effect of genotype and agronomic conditions on metabolomic profiles of selected strawberry cultivars with different sensitivity to environmental stress. Plant Physiol Biochem. (2016) ;101: :14–22. doi:10.1016/j.plaphy.2016.01.016 |
[46] | Harrington PdB , Vieira NE , Espinoza J , Nien JK , Romero R , Yergey AL . Analysis of variance-principal component analysis: A soft tool for proteomic discovery. Anal Chim Acta. (2005) ;544: (1-2):118–27. doi:10.1016/j.aca.2005.02.042 |
[47] | Harnly J , Chen P , Sun JH , Huang HL , Colson KL , Yuk J et al. Comparison of flow injection MS, NMR, and DNA sequencing: Methods for identification and authentication of black cohosh (Actaea racemosa). Planta Medica. (2016) ;82: (3):250–62. doi:10.1055/s-0035-1558113 |
[48] | Luthria DL , Mukhopadhyay S , Robbins RJ , Finley JW , Banuelos GS , Harnly JM . UV spectral fingerprinting and analysis of variance-principal component analysis: a useful tool for characterizing sources of variance in plant materials. J Agric Food Chem. (2008) ;56: (14):5457–62. |
[49] | Sun JH , Zhang ML , Kubzdela N , Luo YG , Harnly JM , Chen P . Determination of variance of secondary metabolites in lettuces grown under different light sources by flow injection mass spectrometric (FIMS) fingerprinting and ANOVA-PCA. J Anal Test. (2018) ;2: (4):312–21. doi:10.1007/s41664-018-0072-6 |
[50] | Chen P , Harnly JM , Lester GE . Flow injection mass spectral fingerprints demonstrate chemical differences in Rio Red grapefruit with respect to year, harvest time, and conventional versus organic farming. J Agric Food Chem. (2010) ;58: (8):4545–53. doi:10.1021/jf904324c |
[51] | Janisiewicz WJ , Takeda F , Short B , Leskey T . PhylloLux Technology for Crop Protection: alternative management of diseases and arthropods for strawberry production. Progressive Crop Consultant. (2018) ;(3):4–8. |
[52] | Janisiewicz W , Takeda F , Short BD , Leskey TC . UV-C irradiation technology: nonchemical disease and arthropods management. Program for 9th North American Strawberry Symposium, February 3-6, Orlando, FL. 2019;16. |
[53] | Peres NA , Turechek W , Gaduory DM , Stensvand A , Schnabel G . Strategic management of multiple diseases in strawberry nurseries and production fields. Program for 9th North American Strawberry Symposium, February 3-6, Orlando, FL. 2019;11. |
[54] | Stensvand A , Suthaparan A , Asalf B , Pathak R , Gislerød HR , Solhaug KA , From P . Onofre R , Peres NA , Turechek W , Bierman A , Rea M , Davidson L-C , Gadoury DM , Non-chemical control of powdery mildew in strawberry. Program for 9th North American Strawberry Symposium, February 3-6, Orlando, FL. 2019;16. |
[55] | Takeda F , Janisiewicz WJ , Smith BJ , Nichols B . A new approach for strawberry disease control. Eur J Hortic Sci. (2019) ;84: (1):3–13. doi:10.17660/Ejhs.2019/84.1.1 |
[56] | Vervoort M , Baets D , Stoffles K , Melis P , Anthonis J , Van Delem T . Autonomous UV-C application on tabletop strawberries to control mildew: optimization and compatibility with IPM. Program for 9th North American Strawberry Symposium, February 3-6, Orlando, FL. 2019;15. |
[57] | Advanced Mass Spectral Database. 2020. Available from: www.mzcloud.org/ |