High-Fat Diets in Animal Models of Alzheimer’s Disease: How Can Eating Too Much Fat Increase Alzheimer’s Disease Risk?
Abstract
High dietary intake of saturated fatty acids is a suspected risk factor for neurodegenerative diseases, including Alzheimer’s disease (AD). To decipher the causal link behind these associations, high-fat diets (HFD) have been repeatedly investigated in animal models. Preclinical studies allow full control over dietary composition, avoiding ethical concerns in clinical trials. The goal of the present article is to provide a narrative review of reports on HFD in animal models of AD. Eligibility criteria included mouse models of AD fed a HFD defined as > 35% of fat/weight and western diets containing > 1% cholesterol or > 15% sugar. MEDLINE and Embase databases were searched from 1946 to August 2022, and 32 preclinical studies were included in the review. HFD-induced obesity and metabolic disturbances such as insulin resistance and glucose intolerance have been replicated in most studies, but with methodological variability. Most studies have found an aggravating effect of HFD on brain Aβ pathology, whereas tau pathology has been much less studied, and results are more equivocal. While most reports show HFD-induced impairment on cognitive behavior, confounding factors may blur their interpretation. In summary, despite conflicting results, exposing rodents to diets highly enriched in saturated fat induces not only metabolic defects, but also cognitive impairment often accompanied by aggravated neuropathological markers, most notably Aβ burden. Although there are important variations between methods, particularly the lack of diet characterization, these studies collectively suggest that excessive intake of saturated fat should be avoided in order to lower the incidence of AD.
INTRODUCTION
Alzheimer’s disease (AD) remains the most prevalent cause of dementia, affecting over 40 million people worldwide [1–4]. From a neuropathological standpoint, two main hallmarks drive AD pathology: extracellular accumulation of amyloid peptides (Aβ) that form toxic senile plaques and the aggregation of neurofibrillary tangles (NFTs) made of hyperphosphorylated tau (ptau). Both Aβ and ptau are central for the diagnosis of AD [1, 4–7]. While these neuropathological markers are essential for a correct post-mortem diagnosis, their role on the etiopathophysiology of the disease is unclear. Decades of research has exposed the multifactorial nature of AD, which has led to a shift from targeting classical AD hallmarks separately to considering other risk factors that alter the progression of the pathology, including metabolic and cardiovascular deficits [6, 8–14]. Indeed, many clinical investigations now focus on improving brain metabolism by the implementation of insulin modulators or diets [15–18]. Though such investigations provide room for optimism, none has secured approval by health agencies.
In the last two decades, nutrition has risen to the forefront of research on neurodegenerative diseases (NDDs). Epidemiological and preclinical endeavors have provided solid evidence that an adequate nutrition may benefit cognition, at least in some population or experimental settings [19–21]. Dietary fats have received particular attention given the extent by which they are found in different human diets and because brain lipid concentrations, particularly fatty acids, can be altered by the composition of diet [20, 22–25]. In this sense, studies generally point to diets rich in poly- and monounsaturated fatty acids (PUFA, MUFA) to be associated with a lower incidence of NDDs. By contrast, diets rich in saturated fatty acids (SFA) are commonly deemed detrimental for cognition [26–28]. The link between brain function, lipid content, and diet composition has thus led to the hypothesis that dietary interventions may be used to prevent or modify disease progression in NDDs [2, 29–36].
Understanding the implications of nutritional factors on NDDs implies evaluating diets that can be preventive as well as those classically deemed detrimental for neurodegeneration. Whereas randomized controlled trials (RCTs) are essential to issue health recommendations and epidemiological studies are useful to detect associations, they remain silent on possible causal relationships. DHA for example, has been studied in RCTs of cognitive decline [29, 30, 37] and high MUFA/DHA diets are now routinely incorporated into multidomain clinical trials [2, 20, 31–33]. However, other types of fats cannot be investigated in clinical trials due to practical and ethical reasons. Dietary lipids such as SFA and trans-fatty acids cannot form part of RCTs given their possible detrimental effects on human health. In this sense, studies in animal models of NDDs offer a valuable opportunity to directly assess the impact of diets on mechanistic endpoints.
Data tell us that HFD have been extensively used to induce obesity and metabolic disease to model type 2 diabetes mellitus (T2DM) in preclinical models of AD (Table 1) [38]. However, the effect of HFD on AD related outcomes is still debatable, with a previous review already highlighting some inconsistency in the results published [39]. The present work seeks to provide a detailed overview of the HFD studies carried out in the last 30 years in animal models of AD, highlighting the consistent and less consistent results obtained. A focus will be set on cognitive endpoints and on the classical neuropathological markers of AD, but other AD-relevant outcomes will also briefly be discussed. We will emphasize the importance of detailing the nutritional content of experimental diets to generate reproducible data. Overall, this study responds to a need created by the multitude of studies published, with sometimes apparently contradictory results, carried out using a variety of methodological approaches.
ASSOCIATIONS BETWEEN DIETARY FATS AND THE INCIDENCE OF NEURODEGENERATIVE DISEASES
Saturated fatty acids
SFA are carbon chains containing a methyl group on one end of its structure and a carboxyl group at the other end. SFA, by definition, do not present double bonds in their structure and can be classified into two broad categories: long-chain saturated fatty acids (LCFA) and short-chain fatty acids (SCFA), although this is not a standardized definition [40, 41]. LCFA are typically found in dairy and red meat, but food sources contain a mixture of different fatty acids, which can influence their different physiological effects [40].
The health implications of high SFA intake have been studied mostly in respect to cardiovascular disease. Dietary saturated fats have been considered atherogenic, in part, due to their action on blood cholesterol [42, 43]. While recommendations to limit saturated fat intake have been widely accepted, recent studies conclude that saturated fats have no significant effect on cardiovascular or total mortality [40, 44]. This is particularly the case for high SFA foods such as dairy, unprocessed meat, and chocolate [40, 44]. Studies in animals also suggest that the actual processing of lipids is important for their health effects. For example, highly processed coconut oil raises serum cholesterol while virgin coconut oil does not [45, 46]. Thus, the physiological response to high SFA intake goes beyond a “black and white” scenario where different factors such as food source and processing must be considered.
Variation in the dietary uptake of fatty acids have also been implicated in risk of dementia [47–50]. Clinical cohorts, including the Chicago Health and Aging Project (CHAP), the Cardiovascular Risk Factors, Aging and Dementia (CAIDE), and the Three-City study, associate multi-nutrient diets low in PUFA and high in SFA with increased risk of dementia and cognitive deficits [47, 49, 51–53]. Further associations between multi-nutrient diets low in SFA and high in fruit, vegetables, olive oil, and fish strengthen the link between low SFA intake and better cognitive health [25, 54–64]). Albeit some exceptions [65, 66], circulating lipids including LCFA and PUFA also appear to be modulated in dementia and have been regarded as possible biomarkers of cognitive status [67–72]. Thus, lipid metabolism, including that of SFA, remains a critical aspect of dementia that should be taken into account in new interventions for neurodegenerative diseases.
Mono- and poly-unsaturated fatty acids
Contrary to SFA, monounsaturated fatty acids (MUFA) and polyunsaturated fatty acids (PUFA) have one or more carbon-carbon double bonds in their structures. Both MUFA, such as oleic acid (18 : 1), and n-3 PUFA, such as DHA and EPA, have received increasing attention in dementia research as they play fundamental roles in brain structure and function [73–75]. Associative studies consistently report a healthier lifestyle and high PUFA intake with lower risks of dementia [20, 23, 25, 27, 69, 76]. These observations have been studied in registered RCTs where results have been less conclusive [21, 33, 77–80]. Still, multi-domain interventions aiming at preventing dementia via modification of lifestyle factors include high ratios of MUFA and PUFA as part of their dietary patterns and have shown some benefits on cognitive scores [2, 31, 73, 81]. Altogether, while preventive measures based on dietary patterns including PUFA and MUFA, combined with changes in other lifestyle habits, attract a growing interest as a mode of intervention in dementia [2, 9, 10, 20, 23, 25, 27, 31, 64, 76, 81–86], there are many gaps in our understanding of their causal association with brain function that deserve further study.
ANIMAL MODELS USED IN AD RESEARCH
Animal models constitute a critical part of AD research that serve as the basis to decipher pathological mechanisms and test therapeutic targets. Thus, preclinical models that accurately modulate AD pathology are needed [87–90]. Of these, mice are the most commonly used given their relative ease of breeding and genetic manipulation [90, 91]. They also allow the control of study variables to an extent that is not possible in humans. For example, inducing obesity through HFD to determine its impact on AD pathology in humans would be unethical. Mouse models thus, provide an essential tool to bridge the gap in translating preclinical to clinical outcomes in AD research [88, 92]. That being said, one must keep in mind that, because of their short lifespans, mouse models cannot emulate the aging process as it occurs in elderly humans and thus cannot fully incorporate the main risk factor of AD, namely old age. As AD-like pathology does not spontaneously develop in mice, these transgenic mice are rather models of living brains exposed to the progressive build up of molecular hallmarks of AD. The objective behind the use of these models is not to discover what is the initiating culprit that triggers the development of AD (i.e., etiology), but rather to study how an experimental intervention impacts the classical features of AD, in a fully controlled setting. In this perspective, risk factors associated with AD are anticipated to exacerbate AD-related pathologies. Conversely, potential treatments are expected to either mitigate this pathology or delay its onset.
WHAT CONSTITUTES A GOOD MODEL FOR AD?
AD models should display neuropathological hallmarks that are central for diagnosis; that is Aβ pathology and ptau, with ideally some degree of synaptic and neuronal damage [91]. So far, no model fully achieves every feature, most recapitulating only one or a limited set of pathological hallmarks [93, 94]. Nonetheless, a milestone in the development of rodent models of AD came with the implementation of transgenic technology in the early 1990 s. Since murine Aβ and tau are not as prone to form insoluble deposits as human Aβ and tau, the introduction of human transgenes becomes necessary to generate mouse models of AD neuropathology. This made it possible to study in vivo genetic mutations known to cause AD in humans, giving rise to transgenic models bearing one or several phenotypes of the disease. These models have been valuable tools in supporting the launching of clinical trials. The database Alzforum reports 162 mouse models of AD, summarizing the numerous options available and reflecting the progression of new technologies in this area. Defining a good model for AD depends much on the hypothesis investigated. Some models are characterized by a fast induction of AD neuropathology (e.g., intracerebral infusion of Aβ) [95] while in other models this happens more progressively (e.g., chronic transgene expression) [96–98]. The following section briefly covers some of the most prominent mouse models that have been used to investigate HFD, but more extensive reviews can be found elsewhere [90–92, 99–101].
TRANSGENIC MODELS FOR AD
Transgenic models expressing human APP mutations
One of the earliest models of transgenic mice harboring a human APP mutation is the PDAPP mouse model [102, 103]. PDAPP mice exhibit cognitive deficits in spatial and recognition memory from a young age (at 4 and 6 months) [104, 105] and a 10-fold increase in APP, extracellular plaque formation, gliosis, and synaptic deficits [104]. The generation of the Tg2576 model followed PDAPP and is considered one of the most well characterized mouse models of AD [96]. This model overexpresses human APP containing the Swedish mutations (K670 N, M671 L) and develops age-associated cognitive deficits, Aβ plaques and vascular amyloid by 11-13 months [106]. However, a limitation associated with these transgenic models is the lack of extensive neuronal loss and brain atrophy that is seen in AD patients [106].
Efforts to induce a more severe neuropathological phenotype resulted in mice expressing multiple mutations of the familial form of AD (FAD). The J20 model carries two FAD mutations in the APP gene (Swedish and Indiana) and expresses high levels of Aβ [107] while 5xFAD mice harbors five mutations in the APP and PSEN1, showing an aggressive form of the pathology as early as 2 months of age [93]. Mice expressing FAD mutations can partially induce AD-pathology and are useful to study Aβ plaque formation and cognitive deficits. However, a complete recapitulation of AD still lacks in these models given the limited neurodegeneration observed and the absence of tau pathology [108, 109].
Transgenic models with tau pathology
Contrary to FAD mutations in the APP gene, genetic mutations for tau protein have not been described in AD thus far. Instead, models expressing mutations associated with frontotemporal lobar degeneration (FTLD) in the MAPT gene are used to mimic tau pathology observed in AD [110]. The JNPL3 mouse is the first model reported in this category and harbors the P301 L mutation, developing motor and behavioral deficits with age-dependent accumulation of NFTs [111]. In general, tau transgenic mice exhibit NFT-like pathology, neuronal and synaptic loss and cognitive impairment in an age-dependent manner [110]. However, the phenotype induced in these models is not entirely representative of that in human AD since NFT development in these mice requires FTLD mutations, which are not found in AD [112]. An exception is the hTau mouse, which expresses only non-mutated human tau isoforms in the absence of the murine orthologs but nonetheless develops tangles and cognitive deficits [113].
Transgenic models with both Aβ and tau pathologies
A more representative way to study AD pathology is through preclinical models that express both Aβ and NFT pathology. Achieving such a task relies on the simultaneous expression of mutated APP, microtubule-associated protein tau (MAPT) and sometimes PSEN1 or PSEN2. The 3xTg-AD model combines familial mutations for AD (APP Swedish, and PSEN1 M146 V) and FTLD (MAPT P301 L) and is one of the most used transgenic models of AD [90, 109, 114, 115]. These mice develop progressive accumulation of Aβ and NFTs in the cortex and hippocampus, as well as deficits in learning and memory by 6 months [98, 116]. In addition, several sexual dimorphisms have been noted in the 3xTg-AD model as female mice tend to exhibit more pronounced Aβ pathology and age-dependent deterioration in glucose metabolism [115, 117–121]. Altogether, this transgenic model offers the opportunity to study AD in a context of both Aβ and tau pathologies while also considering a degree of metabolic dysfunction.
Knock-in models
Most of the above cited models depend not only on APP mutations to generate Aβ pathology but also in overexpression of APP driven by an exogenous promoter. Since the transgene can be integrated at random locations and in multiple copies, an artificial phenotype of AD can be induced [89, 90, 109, 122]. To overcome setbacks of APP overexpression, knock-in models were developed to produce pathogenic Aβ42 [88, 89, 92, 122–125]. In such models, Aβ is humanized by changing the three amino acids that differ between mice and humans (G676 R, F681Y, H684 R) and two FAD mutations are introduced into the App murine gene [108]. The pathology is generally less aggressive than in previous transgenic models and the timing of pathology depends on the mutation expressed (https://www.model-ad.org/resources/). These mice may represent a step towards a more physiologically accurate model of AD in mice, but defining appropriate controls in knock-in models is complicated [88]. In other words, models with overexpressed genes can be compared with their wildtype controls while it is likely that the human KI gene does not behave like its endogenous murine counterpart [126].
Other rodent models
Mouse models that introduce genetic risk factors for sporadic AD, such as the ɛ4 allele of APOE have also been developed, but many of them have not been exposed to HFD protocols yet [127, 128]. Transgenic mice expressing either human APOE ɛ3, or ɛ4 under the regulation of different promoters, including the neuro-specific enolase (NSE) and glial fibrillary acidic protein (GFAP) have been used for more than a decade [129, 130]. APOE ɛ4 mice have shown age and sex-dependent deficits in learning and high phosphorylation of tau in neurons [99, 131, 132]. The development of mice with different mutations related to AD (APP, PS1, MAPT, and APOE) has also allowed for the creation of double mutant mouse lines. Models like the 5xFAD mice and TgCRND8x, for example, harbor mutations on APP+PS1, and APP+APOE, respectively [93, 133]. Other models have made it possible to study neurovascular dynamics. Transgenic mouse strains with tamoxifen-inducible deletion of Lrp 1 (Slco1c1-CreERT2 Lrp1fl/fl mice) in brain endothelial cells have been used to study the role of LRP1 in AD pathology [134, 135]. In addition to transgenic and knock-in models, cognitive deficits can be induced in mice using Aβ peptides, streptozotocin (STZ), okadaic acid, ibotenic acid, and quinolinic acid. Of these, intracerebroventricular (ICV) injection of Aβ peptides remains the most representative, showing neuronal loss and cognitive deficits similar to AD [136]. Though these mouse models continue to be used, the development of transgenic models have progressively replaced their use in AD research as they mimic more closely the slow progression of AD pathology [100]. Of note, rat and rabbit models have also been developed, at greater cost but with the advantage of allowing for more in-depth behavioral evaluations [137–139]. Finally, many research teams have tried to identify spontaneous models of AD with relatively limited success, particularly because of their short lifespan [90, 140, 141]. When the research in progress will bear fruit, we will develop a better understanding of the impact of HFD on these promising new models.
WHICH FAT MAKES A HFD?
The typical American or European diet contains 36–40% fat by calories [142]. A human HFD contains somewhere between 50% and 60% of fats relative to total caloric intake [143] and may include other nutrients such as cholesterol, sugars, and sodium [144]. HFD in preclinical research aim to replicate as close as possible a human diet enriched in fat and a variety of HFD have been used with differences in fat percentage, fatty acid composition and fat sources [145]. In the scope of this review, mouse diets include a range between 35–60% kcal from fat (Table 1). Fatty acid composition in such diets can also vary in terms of saturation percentage and fat source. Altogether, the common consensus of the definition of a HFD is limited to an elevated percentage of kcal in fat, leaving a gap for variability in terms of fat sources and other macro- and micronutrients.
RELEVANCE OF HFD IN ANIMAL MODELS OF AD
Obesity and T2DM are major health problems that result from high consumption of fat and calories along with a sedentary lifestyle [146]. Besides affecting peripheral organs, both obesity and T2DM have been associated with higher risks of AD [147–153]. Indeed, mid-life obesity has been associated with nearly a three-fold higher risk of developing dementia and with a decreased hippocampal volume [147, 154]. Metabolic dysfunctions in glucose metabolism, insulin signaling, and inflammation resulting from T2DM have also been proposed to contribute to AD pathology while metabolic determinants are considered potential therapeutic targets in AD [8, 155–159]. Causative relations and mechanistic processes, however, are more elusive. Therefore, testing HFD in animal models of AD adds the opportunity to decipher overlapping mechanisms linking obesity, T2DM, and AD. Moreover, long-term HFD, which can be proinflammatory, may also enhance “inflammaging”, a pathway correlating aging and age-related disease with chronic inflammation that is hypothesized to be an initiator of AD [160–165]. Of note, differences regarding the way HFD modulate metabolic indices and AD-like pathology have been linked to biological sex. Male rodents fed a HFD have shown worse glucose metabolism, inflammation, anxiogenic and cognitive deficits [120, 166, 167]. On the other hand, higher inflammation and learning deficits have also been reported in female mice on a HFD [168, 169]. All in all, it is important to consider sex-specific mechanisms when implementing dietary protocols as they may be affected differently by HFD. The following section summarizes evidence from HFD studies in mouse models of AD with outcomes focused on glucose metabolism, insulin sensitivity, Aβ and tau pathology, cognition, and behavior.
EFFECTS OF HFD ON THE METABOLIC PROFILE IN THE MOUSE: RELEVANCE TO AD
Glucose metabolism
Defects of brain glucose uptake is one of the most replicated observations in AD patients and one of the most key information brought by positron emission tomography (PET) over the last decades [170–173]. Relative to its size, the energy required by the brain is high. It is estimated that 20% of energy uptake, mostly in the form of glucose, is destined for this organ [18]. Thus, any disruption of glucose homeostasis may consequently impact brain function. Deficits in normal glucose metabolism may result from the normal aging process but are more pronounced in people with dementia [18, 174–176]. Glucose intolerance and hyperglycemia are prevalent conditions among AD patients and correlate with AD pathology, even without the development of diabetes [177–180].
In mice, metabolic deficits are typically assessed through the glucose tolerance test (GTT), which involves measuring blood glucose concentration at 15-min intervals for 2 h after the administration of a bolus of glucose (1 g/kg, i.p.) to fasted mice [117, 178, 181–184]. The AUC (area under the curve) is then determined and compared between groups. Overall, these tests give an indication of the metabolized glucose during the 2 h period and serve as a tool to evaluate changes in glucose metabolism in AD mice fed a HFD. Imaging techniques such as the 18FDG-PET, and magnetic resonance imaging (MRI), including chemical exchange saturation transfer (CEST)-MRI, have been used in mouse models to assess 2D-glucose uptake, and should be further explored to assess the impact of dietary lipids in glucose metabolism [185–188].
HFD typically induces deficits in peripheral glucose metabolism such as hyperglycemia and aggravated glucose tolerance across distinct AD models (Table 1) [189–194]. In addition, abnormal Aβ peptide metabolism in the brain and periphery of AD mice may also contribute to metabolic disturbances. For example, the genetic induction of AD-like pathology in the brain has been shown to lead to age-dependent deterioration of peripheral glucose tolerance in female 3xTgAD mice at 14 months in comparison to NonTg mice [117, 178, 195], reflecting an overlap between glucose dysmetabolism and AD pathology.
Insulin
While the main role of insulin in peripheral tissues is that of facilitating glucose uptake, its physiological role in the CNS goes beyond maintaining metabolic homeostasis. Insulin receptors in the hippocampus may modulate central pathways involved in neuronal plasticity, learning and memory [196]. Both central and systemic deficits in insulin signaling have been suggested to contribute to AD pathology with therapeutic efforts focusing on improving insulin metabolism in the brain [197–202]. In addition, preclinical and clinical trials show that insulin may improve cognitive functions [159, 178, 197, 203–206], making it an important target linking metabolism and cognition.
Different techniques may be utilized to evaluate insulin sensitivity in animal models. The “gold-standard” to carefully evaluate insulin sensitivity is the hyperinsulinemic-euglycemic (HIE) clamp [207]. When combined with radioactive hexose tracers, the HIE clamp allows to assess whole-body hepatic and peripheral insulin sensitivity in awake mice [208]. This is the only technique that allows the simultaneous determination of both hepatic and peripheral insulin sensitivity in vivo. However, such methodology is invasive and requires specialized technical skills, limiting its implementation in animal models of AD, which require aging [210].
The insulin tolerance test (ITT) and the homeostatic model assessment-IR (HOMA-IR) are simpler alternatives to evaluate insulin action in mice. ITT measures the rate of fall of glucose every 15 min during 1 h after an i.p. injection of an insulin bolus (1 U/kg) [210]. The drop in glucose levels reflects the action of insulin in peripheral tissues, indicating an index of insulin sensitivity [211]. Furthermore, the HOMA-IR assesses β-cell function and insulin resistance from basal glucose and insulin or C-peptide concentrations [212]. Both insulin and C-peptide are produced in equimolar concentrations by pancreatic β-cells and can thus inform on insulin production [213]. However, reproducible results rely on careful manipulation of mice to avoid causing stress and anxiety as well as having exact experimental conditions. Age and sexual dimorphisms have also been reported and it is thus recommended to use age- and sex-matched mice in these tests [211, 214].
Besides deficits in glucose metabolism, high fat intake typically induces deficits in insulin metabolism (hyperinsulinemia and insulin resistance) across mouse models of AD (Table 1) [178, 195, 215, 216]. However, this is less frequently seen in mice expressing tau pathology [217, 218]. Of note, it has been reported that tau can modulate both brain and peripheral insulin metabolism [219, 220], suggesting common pathophysiological pathways between T2DM and tau pathology.
Table 1
Summary of rodent’s studies and one human study investigating the effect of HFD on AD-related outcomes
HFD | Diet comp. | AD model | Metabolism | Cognition / behavior | Neuropathology | Synaptic / Neuroinflamm. | Ref. |
APOE Knock in mouse models | |||||||
WD (45% cal fat, 17% sugar) for 13 weeks | No | APOE ɛ3- and ɛ4-FAD females | ↓ Glucose tolerance (ɛ3FAD) ↑ Adiposity (ɛ3- and ɛ4FAD) ↑ Leptin (ɛ4 > ɛ3FAD) | ↓ Memory (ɛ3FAD) | ↑ Aβ pathology (ɛ3FAD only) pTau: ND | No change in microglia | [285] |
WD (45% kcal fat, 17% sucrose) for 12 weeks | No | APOE ɛ3- and ɛ4-FAD males | Metabolic impairment | ND | ↑ Aβ pathology (ɛ4FAD only) pTau: ND | ↑ Glial cells in ɛ4FAD-WD | [286] |
HFD (60% kcal fat) for 32 weeks | No | APOE-KO, APOE ɛ3- and ɛ4-trangenic males | Metabolic impairment | ND | Aβ pathology: No change ↓pTau (pSer396,pSer202/Thr205pThr23) | ND | [254] |
Mice carrying human APP mutations | |||||||
Atherogenic diet (15.75% fat, 1.25% chol. and 0.5% Na-cholate) for 4 months | No | Tg2576 | ↑Chol. (High LDL/Low HDL) | ↓ Learning | ↑ Aβ pathology pTau: ND | ND | [240] |
HFD (60% kcal fat) for 5 months | No | Tg2576 | Metabolic impairment | ↓ Learning | ↑ Aβ40/42 pTau: ND | ND | [193] |
HFHC (1.25% Chol., 0.5% cholic acid and 15% fat) 7–10 months | No | TgAPPsw females | ↑Chol. (VLDL) ↑APOE and APOEB | ND | ↑ Aβ pathology pTau: ND | ↑ Microglia activation | [277] |
4 diets including HFD (60% kcal fat) for 14 weeks | Yes | TgCRND8 | Metabolic impairment | ND | ↑ Aβ pathology pTau: ND | ND | [239] |
HFD (60% kcal fat) for 20 weeks | No | APP/PS1 | Metabolic impairment ↑ Chol., HDL-c | ↓ Memory | ↑Aβ pathology pTau: ND | ND | [189, 190] |
HFD (56.7% kcal fat) for 2 months | Yes | APP/PS1 | Metabolic impairment ↑ Chol. | ↓ Memory | Aβ pathology: no change pTau: ND | ↑ Microglia activation | [191] |
HFD (60% kcal fat) for 23 weeks | No | APP/PS1 | Metabolic impairment ↑ Chol. | ↓ Learning | ↑ Aβ pathology ↑ pTau: No change | No change in synaptic markers ↑ Microglia activation | [242] |
HFD (45% kcal fat) for 5 months | No | APP/PS1 males | Metabolic impairment ↑ Triglycerides | ↓ Memory | ↑ Aβ pathology ↑ pTau (only in NonTg) | ND | [248] |
HFD (45% kcal fat) for 3 months | No | APP/PS1 | Metabolic impairment ↑ Triglycerides | ↓ Memory | ↑ Aβ pathology pTau: no change | No change | [279] |
WD (42% kcal fat, 34% sugar) for 4 months | No | APP/PS1 males | Metabolic impairment | ↓ Memory | ↑ Aβ pathology (microvessels) pTau: ND | ND | [278] |
HFD (60% kcal fat) 7.5–10 months | Yes | APP/PS1 | Metabolic impairment | ↓ Memory/ locomotion | ↑ Aβ pathology pTau: no change | ↑ GFAP and TNF-α | [192] |
HFD (42% kcal fat) 15–17 months | No | APP/PS1 | ND | No change | ↑ Aβ pathology pTau: ND | ↑ Microglial activation | [222] |
HFD (60% kcal fat) for 10 weeks | No | 5xFAD | Metabolic impairment (only in NonTg mice) | ↓ Memory | ↑ Aβ pathology pTau: ND | ND | [283] |
WD (16.4% kcal fat, 57.6% sugars) for 8 months | Yes | APP/PS1 | ↑ Fasting glucose (only in females) | ND | ↑ Aβ pathology pTau: ND | ↑ Astrocytes and microglia (GFAP and IBA1) | [284] |
HFD (40% kcal fat) for 12 months | No | APPNL - F/NL - F males | Metabolic impairment | ↓ Spatial memory | ↑ Aβ pathology pTau: no change | ↑ Microglial activation ↓ PSD95 | [241] |
HFD (60% kcal fat) for 3.5 months then HFD (45% kcal fat) with various ω6/ω3 ratios (1 : 1, 5 : 1 or 16 : 1) | Yes | APP/PS1 males | Metabolic impairment ↑ Serum TC, LDL-c, and HDL-c | No change | ↑ Soluble Aβ40/42 and ↓ Aggregated Aβ40/42 pTau: ND | ↑ NF-kB P65 | [226] |
Mice developing tauopathies | |||||||
HFD (59% kcal fat) for 20 weeks | Non | THY-Tau22 males | Metabolic impairment | ↓ Spatial learning | ↑ pTau (Ser214,Ser404,Ser422only) Aβ: ND | No change in spinophilin or syp. | [217] |
HFD (15.3% fat) for 8.5 months | Yes | PS19 females | Metabolic impairment ↑ Triglycerides, leptin | ND | ↑ pTau (insolublepSer202/Thr205) Aβ: ND | ↓ Syntaxin ↑ Microglia activation | [249] |
5 diets including WD (45% kcal fat, 15% kcal fructose in water) and HFD (45% kcal fat) for 6 months | Yes | hTau | Obesity No change in glucose/ insulin | ND | pTau: no change Aβ: ND | ND | [218] |
HFD (35% wt/ fat, 5% wt sucrose) for 5 months | No | Tg601 females | Metabolic impairment | ↓ Spatial learning and memory | ↑ pTau (pSer214) Aβ: ND | ND | [251] |
Mice carrying mutations on both human APP and MAPT (tau) | |||||||
HFD (60% kcal fat) for 3 months | No | APPSwe-Tau females | Metabolic impairment ↑ Triglycerides ↓ Adiponectin | No change | Aβ: ND pTau: no change | ND | [253] |
3 diets: HF/low ω3: ω 6 ratio (35% wt/ fat) for 9 months | Yes | 3xTg-AD | Metabolic impairment ↓ DHA levels in the brain | ↓ Memory | ↑ Aβ pathology ↑pTau (totaltau) | ↑ GFAP ↓ Drebrin (unchanged syp., SNAP25 and syntaxin-3) | [250] |
HFD (60% kcal fat) for 4 months | Yes | 3xTg-AD | Metabolic impairment (males) ↑ Adiposity (males) | ↓ Exploration (males), ↓ Alterations | ↑ Aβ pathology pTau: no change | ND | [120] |
HFD (60% kcal fat) for 9 months | Yes | 3xTg-AD females | Metabolic impairment ↑ Adiposity | ↓ Memory (no change in anxiety/ locomotion) | ↑ Aβ pathology pTau: no change | No change in drebrin, SNAP25 or syp. | [178] |
HFD (60% kcal fat), starts 2 months of age until 3–4/7–8/11–12 or 15–16 months | No | 3xTg-AD males | Metabolic impairment | ↓ Learning with aging | Aβ: no change pTau: no change | ↑ Microglial activation | [195] |
HFD (60% kcal fat) for 16 weeks | No | 3xTg-AD females | ND | ↓ Memory | Aβ: no change pTau: no change | ND | [223] |
HFD (58% kcal fat) for 6–12 months | No | 3xTg-AD males | Metabolic impairment ↓ Leptin | ↓ Memory | ND | ND | [224] |
HFD (60% kcal fat) for 4 months | No | 3xTg-AD MxD | Metabolic impairment | ↓ Spatial learning/ memory (females) | Aβ: no change pTau: no change | No change | [252] |
HFHC (20% kcal fat, 1.25% chol.) for 60 days | Yes | 3xTg-AD | Obesity, ↑ chol. | ↑ Locomotion unchanged memory | Aβ: ND pTau: ND | ND | [225] |
Human study | |||||||
HFD (45% kcal fat) for 6 weeks | No | Healthy twins (n = 92), 31D±14) years old | ND | ↓ Memory (AVLT in AA carriers) | ND | ND | [305] |
Most studies in rodents have investigated the effect of HFD on metabolic endpoints, behavior, and brain amyloid-β load. Some have studied the effect on tau pathology, synaptic and/or neuroinflammatory markers. Diets were various but most included≥40% kcal from fat. Diet composition enabling replication was available in about 33% of studies. Metabolic impairments were confirmed in a majority of studies, with assessment of weight and deficits in peripheral glucose/insulin metabolism. AA, Homozygous carriers of the rs9472159 polymorphism in SLC2A1 gene; Aβ, amyloid-beta; AVLT, Auditory verbal learning task; Chol., Cholesterol; Comp., Composition; GFAP, Glial fibrillary acidic protein; HDL, High density lipoprotein cholesterol; HF/HC, High Fat+High Cholesterol; HFD, High Fat Diet; IBA-1, Ionized calcium-binding adapter molecule 1; LDL, Low-density lipoprotein cholesterol; LFD, Low Fat Diet; ND, Non-determined; pTau, phosphorylated tau; Neuroinflamm., Neuroinflammation; Syp, synaptophysin; TC, total cholesterol; VLDL, Very low density lipoprotein cholesterol. Mouse models cited: Tg2576 and TgAPPSwe: mice overexpressing a mutant form of APP (APPSwedish); TgCRND8: mice overexpressing 3 human mutations in the APP gene (K670M/N671 L and V171F); APP/PS1: double transgenic mice expressing a chimeric mouse/human amyloid precursor protein (Mo/HuAPP695swe) and a mutant human presenilin 1 (PS1-dE9); THY-Tau 22: model of tauopathy with Tau 2 mutations (G272 V and P301 S); PS19: model of tauopathy with hMAPT mutation on P301 S; hTau: mice lack endogenous murine microtubule-associated protein tau (Mapt) gene expression, and express all six isoforms (including both 3 R and 4 R forms) of hMAPT; APPSwe-Tau: Tg2576/Tau(P301 L) generated by crossing Tg2576 mice, which have the transgene for human APP (isoform 695) carrying the Swedish mutation with JNPL3 mice expressing human MAPT (4 repeat) with the P301 L mutation.; 3xTg-AD: Triple transgenic mice expressing 3 human mutations APPswe, PSIMI46 V on APP and tauP301 L hMAPT to generate Aβ and tau pathologies; MxD: Carotid artery occlusion model.
EFFECTS ON BEHAVIOR AND COGNITION
The effect on cognitive behavior is more challenging to detect in animal models that are already impaired due to a threshold effect and is further complicated by the weight gained after HFD. Obese mice may perform differently in tests requiring movement and exploration such as the Morris Water Maze or the open field test, imposing an “obesity” bias [221]. Thus, the aggravating effects of HFD on cognition and behavior can be hard to demonstrate. For instance, transgenic APP/PS1 mice show impairment in short-term memory, irrespective of diet, while HFD seems to drive impaired memory in wild-type animals [222]. Memory deficits caused by HFD are more pronounced in 3xTg-AD mice, as shown by higher escape latency in comparison to other groups [223]. In addition, the strongly decreased scores in various behavioral tests, such as the Y-maze, Novel Object Recognition, Morris Water Maze, and Barnes maze, observed in 3xTg-AD mice with aging [195], were worsened by a HFD [178, 223, 224]. In some occasions, these changes happened without aggravating Aβ or tau pathology [195, 223]. Overall, albeit some exceptions [222, 225, 226], most studies listed in Table 1 report some sort of additional cognitive and behavioral impairment, but results should be interpreted carefully when working with obese mice.
EFFECTS OF A HFD ON AD NEUROPATHOLOGY
Amyloid-β
Genetic data provide a strong support for the hypothesis that accumulation of Aβ peptides is one of the initiators of AD pathology [227–232]. Increased production and reduced clearance of Aβ result in toxic aggregates of Aβ42 and Aβ40 and may promote the onset of the disease [233–236]. Other data point to Aβ accumulation as the consequence from a complex interplay between neurons, astrocytes, microglia, and vasculature that drives neurodegeneration [6, 237]. From a neuropathological perspective, the extent of neuritic plaques and Aβ aggregates in the brain remain the cornerstone of AD diagnosis, which make Aβ levels an inescapable parameter when assessing AD pathology [1, 238].
Perhaps the most consistently reported impact of a HFD is an aggravation of Aβ pathology in the brain (Table 1). Soluble and insoluble forms of Aβ42, Aβ40, the Aβ42/40 ratio, and plaques increased in the hippocampus or cortex after high-fat consumption [239–242]. Still, as shown in Table 1, HFD may not always induce such changes. Impairments in learning and memory were reported in AD-HFD mice without significant changes on Aβ proteins [191, 195, 223]. Data in animal models support an aggravating effect of HFD on several forms of Aβ but its deleterious impact on cognitive function may also stem from additional mechanisms that are not limited to Aβ pathology.
Hyperphosphorylated tau
Tau is a microtubule associated protein with high susceptibility to post-translational modifications, one of which is phosphorylation [243–245]. Accumulation of tau aggregates that include ptau and NFTs is a classical feature observed in AD brains and strongly correlated with cognitive function [232, 243, 246, 247]. Results on the effect of HFD on tau pathology in mice are more equivocal than with Aβ. As seen in Table 1, some studies report increased forms of ptau in HFD-fed mice, notably in mice expressing transgenes-induced tau pathologies [217, 248–250]. HFD also leads to slight increases limited to specific forms of tau, like cortical ptau, [242], certain tau epitopes [217, 251], and insoluble [249] or total human tau [250]. Additional studies in models carrying both APP and tau mutations show that tau phosphorylation is not markedly affected by HFD consumption or is even decreased (Table 1) [120, 178, 195, 223, 252–254]. Overall, given the dynamic nature of tau, data on tau pathology is highly variable with differences in genotypes, protein forms and anatomical regions. Further studies, with more controlled settings, are thus necessary.
Synaptic markers
Synaptic dysfunction is a common pathological feature in AD that correlates with cognitive decline [232, 243, 255–257]. Synaptophysin, drebrin, PSD-95, SNAP25, and syntaxin are often reported to be downregulated in the AD brain, with synaptophysin distinguishing mild cognitive impairment and AD cases from controls [243, 258, 259]. Prominent decreases in postsynaptic drebrin and PSD-95 have also been reported in the cortex of AD patients [258, 260] while biomarkers of synapse dysfunction have surged as promising measures of cognitive status [255, 261–263]. There is also some evidence linking pathological Aβ and tau to synaptic loss [264–266], further making synaptic dysfunction a key aspect of AD physiopathology.
When reported, high fat intake does not consistently induce significant changes on synaptic proteins (Table 1) [178, 217, 242, 250]. Still, reduced levels of drebrin, synaptophysin, or syntaxin have been reported after high fat consumption [249, 250, 260, 267]. Of note, drebrin is also markedly reduced after n-3 PUFA deprivation [258] and upregulated by dietary DHA in the hippocampus [268] and the cortex [258], perhaps revealing a susceptibility of this protein to specific dietary fats. Though synaptic dysfunction is not routinely included as an endpoint in the studies reviewed, data suggest a tendency for individual synaptic markers to be more strongly affected by the type of fat in diets. It is important to consider that HFD have different ratios of n-3 PUFA that, although found in low levels, can modulate synaptic markers. Thus, it is the proportion of each fatty acid in a diet, rather than their total amount, that appears to exert an influence on synaptic proteins.
Neuroinflammatory markers and oxidative damage
Neuroinflammation and oxidative damage are important features in NDD [269–271]. In the AD brain, microglia bind to misfolded proteins that trigger neuroinflammatory responses, resulting in elevated biomarkers of lipid peroxidation in postmortem brain tissues of AD patients [272, 273]. Obesity and metabolic syndrome are associated with higher markers of inflammation in the periphery while the opposite seems to be true for diets low in fat or carbohydrate [274, 275]. This is consistent with mice fed HFD where inflammatory responses are activated, both in the brain and in peripheral organs (Table 1). APP/PS1 mice fed a HFD have a greater increase in hippocampal microglial activation than transgenic mice fed a normal diet or non-transgenics fed a HFD [191, 192]. Other studies have also reported elevated inflammatory markers in the cortex or hippocampus of mice as a response to HFD and irrespective of genotype [195, 242, 249, 250, 276–278]. Oxidative damage is also aggravated in different organs following a HFD, including the microvasculature [278], cortex [223], and liver [192, 279]. In essence, inflammatory, and oxidative responses in HFD-fed mice remain underlying mechanisms linking nutrition, metabolic dysfunction and AD pathology, but that can be hard to pinpoint methodologically.
HFD WITH SUGAR OR SODIUM
A distinction should be made between diets with a high content of saturated fat only and those that include other nutrients such as salt or sugar. Indeed, besides being high in saturated fat, westernized diets (WD) are associated with overprocessed foods and may incorporate a high content of certain types of proteins, trans fats, refined grains, sugar, alcohol, salt, and high-fructose syrup with a reduction in fruits and vegetables [280]. To recapitulate a more accurate version of a WD in humans, HFD used in animals sometimes include elevated quantities of sugar or sodium [184, 218, 281, 282]. However, the incorporation of these two nutrients may inevitably impose an additional variable for the outcomes seen on AD mice. For example, in 5xFAD mice, a WD led to higher Aβ deposits in hippocampal microvessels with no difference in parenchymal Aβ pathology [283]. Meanwhile, a WD containing 57% kcal in sugars increased hippocampal Aβ42 in APP/PS1 mice [284]. Genotype and sex differences may also be present as WD-fed E3FAD female mice showed higher hippocampal Aβ load whereas no alterations in Aβ pathology were seen in E4FAD mice [285]. The opposite seems to be true for males where a worsening effect of Aβ burden by WD is seen in E4FAD versus the E3FAD genotype [286]. Altogether, when comparing the effect of various HFD on AD endpoints, it is important to consider the inclusion of other nutrients frequently found in WD.
HOW CAN SFA CONTRIBUTE TO AD PATHOLOGY?
Direct effect on the brain
Fifty-five percent (dry weight) of the human brain is composed of lipids. In mammals, PUFA accounts for 30% of brain fatty acids, at least half from DHA, while SFA and MUFA make up the rest (70%) [287–291]. Although the composition in PUFA in the brain was repeatedly shown to be altered by dietary shifts in fatty acids, brain SFA composition is more stable and less dependent on the relative dietary intake [19, 292–295]. Still, specific dietary manipulations high in SFA have shown to increase SFA levels in the cortex and hippocampus [250, 296, 297], showing a degree of susceptibility of brain SFA to dietary manipulations. However, it is not clear yet whether such small changes in membrane SFA can influence physiological properties of neurons, such as those observed with PUFA and MUFA [73, 298–303]. Evidence gathered over the years suggest that HFD may impact the endothelium of brain microvessels, cerebral blood flow, and neuroinflammation while disrupting the equilibrium between the uptake of nutrients versus clearance of toxic proteins. As the brain’s energy requirement is relatively high compared to other organs, any defects in energy uptake would also compromise brain functionality [18]. It has been shown that HFD can downregulate cerebrovascular levels of the glucose transporter-1 (GLUT-1) and decrease insulin degrading enzyme (IDE), resulting in blunted brain glucose uptake and accumulation of toxic Aβ aggregates [155, 304–306]. HFD may also promote central insulin resistance, which is detected in the brain itself in AD, particularly at the vascular level [157, 159, 202] and negatively affect neurovascular coupling and cerebrovascular function even in the absence of dyslipidemia [307]. These metabolic abnormalities would then induce alterations in neuronal morphology and physiology that translate in decreased long-term potentiation and reduced markers of synaptic plasticity [41]. Several lines of studies also suggest that HFD generate a proinflammatory environment, which may further favor Aβ deposition and tau phosphorylation and negatively affect synaptic plasticity [41, 155, 308–312]. Increased lipid peroxidation and reduced autophagy have also been reported in the brain of obese mice while mitochondrial regulators including SIRT3 and PGC1α are reduced by saturated fats and cholesterol consumption, which may promote the accumulation of neurotoxic proteins and lead to neuronal death [313–316]. Furthermore, serotonergic and dopaminergic pathways are susceptible to the excess of fat and sugar intake which can induce in depressive-like behaviors [317–319]. Alterations in insulin metabolism [318] and neuroinflammatory responses in the hypothalamus and hippocampus have been described as likely mechanisms [320–324]. Altogether, changes in the brain lipid profile and downstream metabolites, alterations in central glucose metabolism, and neuroinflammatory responses, all caused by high fat intake, can form part of a pathological loop linking fatty acid consumption and neurodegeneration in AD (Fig. 1).
Fig. 1
High-fat diets (HFD) have significant metabolic effects and can contribute to Alzheimer’s disease (AD) pathology. Possible mechanisms by which HFD can modulate AD pathology are shown, whether directly in the brain or indirectly in the periphery. Data suggest that HFD may promote insulin resistance and decrease GLUT-1 and IDE at the cerebrovascular level while also triggering an inflammatory response in the brain. In the periphery, the alteration of insulin metabolism, adipokines and gut microbiota can indirectly affect brain processes. Finally, the majority of reports indicate that a prolonged exposure to a HFD increases brain Aβ levels, whether its effects in tau pathology is less clear. Aβ, amyloid peptides; BBB, blood-brain barrier; GLUT1, glucose transporter 1; IDE, insulin degrading enzyme; INSR, insulin receptor; SFA, saturated fatty acids.
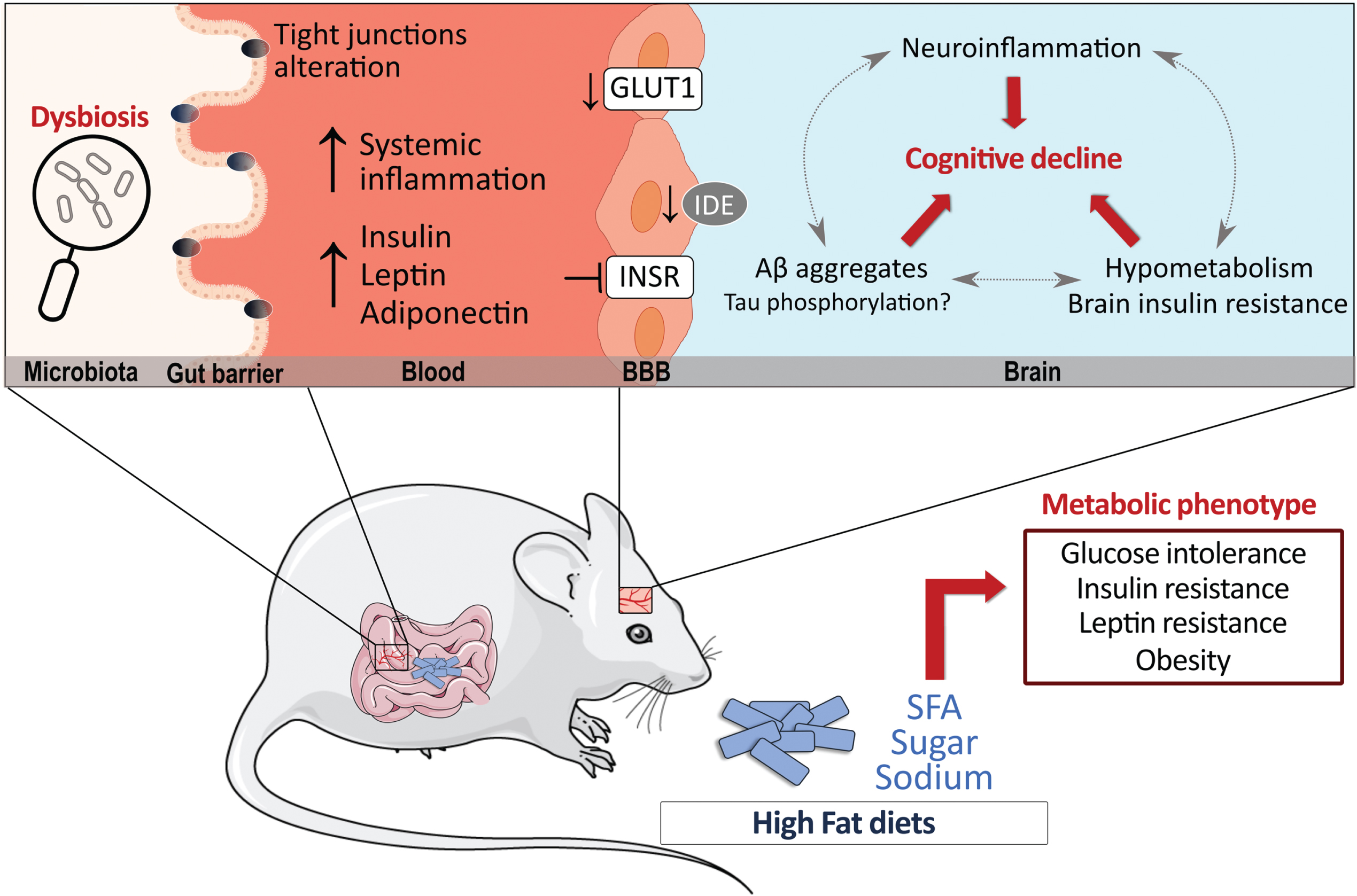
Indirect effect via the periphery
HFD can also impact the brain indirectly through its effect on the periphery [319]. As evidenced by Table 1, when correctly assessed, insulin resistance is often detected in HFD-fed animal models of AD (Table 1). Notably, insulin administration reversed HFD-induced metabolic and cognitive deficits in 3xTg-AD mice while age-dependent deficits in insulin and glucose metabolism have been reported in the 3xTgAD mice, suggesting a vicious cycle between insulin metabolism and AD pathology [117, 178]. The altered metabolism of other adipokines including adiponectin and leptin also stand as key pathways linking T2DM and AD pathology (reviewed elsewhere: [325–327]). Furthermore, specific nutrients have the potential to change gut microbiota and influence CNS diseases upward through the gut-brain axis [328–334]. For example, SFA metabolism has been reported to increase intestinal permeability by affecting tight junction proteins and inducing dysbiosis of gut microbiota, processes that have been implicated in the pathology of AD [335–342]. Therefore, it is likely that exaggerated intake of HFD disrupts energy-regulating processes in the periphery that potentiate the detrimental effects on metabolic, cognitive, and neuropathological dysfunction in AD [8, 18, 73, 178, 197, 343–345].
VARIATIONS IN HFD COMPOSITION AS COFOUNDING FACTORS IN PRECLINICAL STUDIES
The present review highlights some inconsistency in results regarding the effect of HFD on AD-relevant endpoints in animal models (Table 1). An important factor to consider when comparing preclinical studies is the difference between HFD in terms of fat percentage and fat composition. Fat content in HFD can vary from 45% fat kcal to 60% fat kcal [120, 218]. As the definition of HFD is limited to a fat content between 40–60% kcal in fat, using different percentages of fat in diets may inevitably induce different degrees of metabolic deficits in mice, which could affect cognitive, behavioral, and neuropathological markers differently. We have found that complete characterization of the HFD used is reported in a little less than half of published papers, making comparisons even more difficult.
Fat source and composition are also important to consider when comparing HFD studies. HFD are typically made up of a combination of SFA, MUFA, and PUFA, which often varies between diets. For instance, Bracko et al. report a SFA:MUFA:PUFA proportion of 62% : 27% : 4.7%, respectively [222], whereas another HFD rather comprises 22% : 66% : 10.4% of each FA species [191]. Fat source in HFD further contributes to this variability [40]. Such variability in the proportion of fatty acids is critical when using HFD in AD models as PUFA intake, such as DHA, is typically associated with beneficial effects on cognition [20, 23, 25, 27, 76] and MUFA could play a similar role [300, 346]. Thus, a HFD composed mostly of high SFA will yield different results than a HFD based on elevated MUFA or PUFA. Lastly, the impact of dietary treatment on actual fatty acid content in the brain is rarely disclosed in studies. HFD can change the lipid profile in the brain [250, 293, 294, 296, 297, 347], which remains an essential information to draw mechanistic conclusions at the molecular level. Overall, to fully benefit from the advantages of preclinical studies, a complete characterization of the fatty acid profiles both in the diet and in target tissues is necessary and should always be recommended.
LIMITATIONS
The present study provides an overview of the impact of HFD in animal models of AD, but is limited first by the information available in published papers. Although studies that included only female or only male mice were detailed in Table 1, the effects reported did not account for sex-specific effects in many instances. When a study does not report sex-specific interactions, it is difficult to know if they were non-existent or because male and females were not separated in the analysis. However, considerations were made in the text regarding sex-specific effects. Secondly, while we report the duration of diet in Table 1, the age of mice when starting diet consumption was not included, making comparisons between age groups more challenging. Lastly, our focus was on studies in the mouse, but we are aware that there might be HFD studies in other mammals, such as rats, or smaller organisms that were outside the scope of this review. Future studies that aim to fill these gaps would be valuable.
CONCLUSIONS, IMPLICATIONS FOR THE HUMAN CONDITION AND FUTURE STUDIES
Nutrition has become an important factor to consider when developing therapeutic strategies in AD. Diets have the potential to play a protective role, alter positively or negatively disease progression or interact with treatment. Consumption of high amounts of SFA and other energy-dense nutrients is highly prevalent in our society, and this has contributed to higher incidence of not only obesity and T2DM, but also neurodegenerative diseases. Controlled, RCT would be essential to issue recommendations regarding mono- and poly-unsaturated fatty acids. However, testing the effect of HFD in humans would not only be unethical, but would also be plagued by many confounding variables. On the other hand, these diets can be investigated in animal models in fully controlled settings in a shorter timeframe. There are over 30 reports of HFD in animal models of AD that can be found on PubMed since 2002. In rodent models, the intake of a HFD generally aggravates cognitive performance, but most study paradigms can be biased by the weight gain inevitably associated with it. The majority of studies have found an aggravating effect of a HFD intake on brain Aβ pathology. Tau has been much less studied, and results are more equivocal due to the complexity of post-transcriptional changes, while other key AD markers have been even less consistently reported. Discrepancies between studies can be explained by differences in the diet composition, the age and duration of exposure, the exact model used and endpoints assessed, among other variables discussed above. We have found that more than half of reports do not even fully disclose the diets used. Future studies should consider that distinct fatty acids, despite small chemical differences, may modulate pathophysiological pathways very differently. More specifically, when considering HFD protocols, forthcoming studies need to document percentages, sources, and types of fatty acids; aspects that are not consistently reported across laboratories. These are important steps towards harmonizing data regarding the effect of HFD on the different animals in AD, which is pivotal to issue sound conclusions, and to generate evidence that can be compared and replicated, serving as a basis for the launching of clinical trials and discovery of new preventive strategies and therapies in AD.
ACKNOWLEDGMENTS
The authors are grateful to Vincent Émond for his critical comments on the manuscript.
FUNDING
This work was supported by grants from the Canadian Institutes of Health Research (CIHR) to F.C. [grant numbers PJT 168927, PJT 156054]. F.C. is a Fonds de recherche du Québec-Santé (FRQ-S) senior research scholar. J. V-E. was supported by scholarships from Fonds d’Enseignement et de la Recherche (FER) from the Faculty of Pharmacy, Laval University, from the Fondation du CHU de Québec, from the Fondation Lemaire and the Consortium pour l’Identification Précoce de la Maladie d’Alzheimer (CIMA-Q). M.L. was supported by scholarships from the Fondation du CHU de Québec and from FRQ-S.
CONFLICT OF INTEREST
The authors have no conflict of interest to report.
REFERENCES
[1] | Jack CR Jr. , Bennett DA , Blennow K , Carrillo MC , Dunn B , Haeberlein SB , Holtzman DM , Jagust W , Jessen F , Karlawish J , Liu E , Molinuevo JL , Montine T , Phelps C , Rankin KP , Rowe CC , Scheltens P , Siemers E , Snyder HM , Sperling R , Contributors ((2018) ) NIA-AA Research Framework: Toward a biological definition of Alzheimer’s disease. Alzheimers Dement 14: , 535–562. |
[2] | Kivipelto M , Mangialasche F , Snyder HM , Allegri R , Andrieu S , Arai H , Baker L , Belleville S , Brodaty H , Brucki SM , Calandri I , Caramelli P , Chen C , Chertkow H , Chew E , Choi SH , Chowdhary N , Crivelli L , Torre R , Du Y , Dua T , Espeland M , Feldman HH , Hartmanis M , Hartmann T , Heffernan M , Henry CJ , Hong CH , Hakansson K , Iwatsubo T , Jeong JH , Jimenez-Maggiora G , Koo EH , Launer LJ , Lehtisalo J , Lopera F , Martinez-Lage P , Martins R , Middleton L , Molinuevo JL , Montero-Odasso M , Moon SY , Morales-Perez K , Nitrini R , Nygaard HB , Park YK , Peltonen M , Qiu C , Quiroz YT , Raman R , Rao N , Ravindranath V , Rosenberg A , Sakurai T , Salinas RM , Scheltens P , Sevlever G , Soininen H , Sosa AL , Suemoto CK , Tainta-Cuezva M , Velilla L , Wang Y , Whitmer R , Xu X , Bain LJ , Solomon A , Ngandu T , Carrillo MC ((2020) ) World-Wide FINGERS Network: A global approach to risk reduction and prevention of dementia. Alzheimers Dement 16: , 1078–1094. |
[3] | Frisoni GB , Boccardi M , Barkhof F , Blennow K , Cappa S , Chiotis K , Démonet JF , Garibotto V , Giannakopoulos P , Gietl A , Hansson O , Herholz K , Jack CR Jr. , Nobili F , Nordberg A , Snyder HM , Ten Kate M , Varrone A , Albanese E , Becker S , Bossuyt P , Carrillo MC , Cerami C , Dubois B , Gallo V , Giacobini E , Gold G , Hurst S , Lönneborg A , Lovblad KO , Mattsson N , Molinuevo JL , Monsch AU , Mosimann U , Padovani A , Picco A , Porteri C , Ratib O , Saint-Aubert L , Scerri C , Scheltens P , Schott JM , Sonni I , Teipel S , Vineis P , Visser PJ , Yasui Y , Winblad B ((2017) ) Strategic roadmap for an early diagnosisof Alzheimer’s disease based on biomarkers. Lancet Neurol 16: , 661–676. |
[4] | Scheltens P , Blennow K , Breteler MMB , de Strooper B , Frisoni GB , Salloway S , Van der Flier WM ((2016) ) Alzheimer’s disease. Lancet 388: , 505–517. |
[5] | Guo T , Noble W , Hanger DP ((2017) ) Roles of tau protein in health and disease. Acta Neuropathol 133: , 665–704. |
[6] | Golde TE ((2022) ) Alzheimer’s disease - the journey of a healthy brain into organ failure. Mol Neurodegener 17: , 18. |
[7] | Arvanitakis Z , Shah RC , Bennett DA ((2019) ) Diagnosis and management of dementia: Review. JAMA 322: , 1589–1599. |
[8] | Tournissac M , Leclerc M , Valentin-Escalera J , Vandal M , Bosoi CR , Planel E , Calon F ((2021) ) Metabolic determinants of Alzheimer’s disease: A focus on thermoregulation. Ageing Res Rev 72: , 101462. |
[9] | Moll van Charante EP , Richard E , Eurelings LS , van Dalen JW , Ligthart SA , van Bussel EF , Hoevenaar-Blom MP , Vermeulen M , van Gool WA ((2016) ) Effectiveness of a 6-year multidomain vascular care intervention to prevent dementia (preDIVA): A cluster-randomised controlled trial. Lancet 388: , 797–805. |
[10] | Ngandu T , Lehtisalo J , Solomon A , Levalahti E , Ahtiluoto S , Antikainen R , Backman L , Hanninen T , Jula A , Laatikainen T , Lindstrom J , Mangialasche F , Paajanen T , Pajala S , Peltonen M , Rauramaa R , Stigsdotter-Neely A , Strandberg T , Tuomilehto J , Soininen H , Kivipelto M ((2015) ) A 2 year multidomain intervention of diet, exercise, cognitive training, and vascular risk monitoring versus control to prevent cognitive decline in at-risk elderly people (FINGER): A randomised controlled trial. Lancet 385: , 2255–2263. |
[11] | Cummings J , Lee G , Ritter A , Sabbagh M , Zhong K ((2020) ) Alzheimer’s disease drug development pipeline: 2020. Alzheimers Dement (N Y) 6: , e12050. |
[12] | Wahl D , Solon-Biet SM , Cogger VC , Fontana L , Simpson SJ , Le Couteur DG , Ribeiro RV ((2019) ) Aging, lifestyle and dementia. Neurobiol Dis 130: , 104481. |
[13] | Scheltens P , De Strooper B , Kivipelto M , Holstege H , Chetelat G , Teunissen CE , Cummings J , van der Flier WM ((2021) ) Alzheimer’s disease. Lancet 397: , 1577–1590. |
[14] | Royea J , Hamel E ((2020) ) Brain angiotensin II and angiotensin IV receptors as potential Alzheimer’s disease therapeutic targets. Geroscience 42: , 1237–1256. |
[15] | Burns DK , Alexander RC , Welsh-Bohmer KA , Culp M , Chiang C , O’Neil J , Evans RM , Harrigan P , Plassman BL , Burke JR , Wu J , Lutz MW , Haneline S , Schwarz AJ , Schneider LS , Yaffe K , Saunders AM , Ratti E ((2021) ) Safety and efficacy of pioglitazone for the delay of cognitive impairment in people at risk of Alzheimer’s disease (TOMMORROW): A prognostic biomarker study and a phase 3, randomised, double-blind, placebo-controlled trial. Lancet Neurol 20: , 537–547. |
[16] | Luchsinger JA , Perez T , Chang H , Mehta P , Steffener J , Pradabhan G , Ichise M , Manly J , Devanand DP , Bagiella E ((2016) ) Metformin in amnestic mild cognitive impairment: Results of a pilot randomized placebo controlled clinical trial. J Alzheimers Dis 51: , 501–514. |
[17] | Koenig AM , Mechanic-Hamilton D , Xie SX , Combs MF , Cappola AR , Xie L , Detre JA , Wolk DA , Arnold SE ((2017) ) Effects of the insulin sensitizer metformin in Alzheimer disease: Pilot data from a randomized placebo-controlled crossover study. Alzheimer Dis Assoc Disord 31: , 107–113. |
[18] | Cunnane SC , Trushina E , Morland C , Prigione A , Casadesus G , Andrews ZB , Beal MF , Bergersen LH , Brinton RD , de la Monte S , Eckert A , Harvey J , Jeggo R , Jhamandas JH , Kann O , la Cour CM , Martin WF , Mithieux G , Moreira PI , Murphy MP , Nave KA , Nuriel T , Oliet SHR , Saudou F , Mattson MP , Swerdlow RH , Millan MJ ((2020) ) Brain energy rescue: An emerging therapeutic concept for neurodegenerative disorders of ageing. Nat Rev Drug Discov 19: , 609–633. |
[19] | Kerdiles O , Layé S , Calon F ((2017) ) Omega-3 polyunsaturated fattyacids and brain health: Preclinical evidence for the prevention ofneurodegenerative diseases. Trends Food Sci Technol 69: , 203–213. |
[20] | Scarmeas N , Anastasiou CA , Yannakoulia M ((2018) ) Nutrition and prevention of cognitive impairment. Lancet Neurol 17: , 1006–1015. |
[21] | Joffre C , Nadjar A , Lebbadi M , Calon F , Laye S ((2014) ) n-3 LCPUFA improves cognition: The young, the old and the sick. Prostaglandins Leukot Essent Fatty Acids 91: , 1–20. |
[22] | Morris MC , Tangney CC ((2014) ) Dietary fat composition and dementia risk. , S. Neurobiol Aging 35 Suppl 2: , 59–64. |
[23] | Dhana K , Evans DA , Rajan KB , Bennett DA , Morris MC ((2020) ) Healthy lifestyle and the risk of Alzheimer dementia: Findings from 2 longitudinal studies. Neurology 95: , e374–e383. |
[24] | Flanagan E , Lamport D , Brennan L , Burnet P , Calabrese V , Cunnane SC , de Wilde MC , Dye L , Farrimond JA , Emerson Lombardo N , Hartmann T , Hartung T , Kalliomäki M , Kuhnle GG , La Fata G , Sala-Vila A , Samieri C , Smith AD , Spencer JPE , Thuret S , Tuohy K , Turroni S , Vanden Berghe W , Verkuijl M , Verzijden K , Yannakoulia M , Geurts L , Vauzour D ((2020) )Nutrition and the ageing brain: Moving towards clinical applications. Ageing Res Rev 62: , 101079. |
[25] | Samieri C , Morris MC , Bennett DA , Berr C , Amouyel P , Dartigues JF , Tzourio C , Chasman DI , Grodstein F ((2018) ) Fish intake, genetic predisposition to Alzheimer disease, and decline in global cognition and memory in 5 cohorts of older persons. Am J Epidemiol 187: , 933–940. |
[26] | Molsberry S , Bjornevik K , Hughes KC , Healy B , Schwarzschild M , Ascherio A ((2020) ) Diet pattern and prodromal features of Parkinson disease. Neurology 95: , e2095–e2108. |
[27] | Barbaresko J , Lellmann AW , Schmidt A , Lehmann A , Amini AM , Egert S , Schlesinger S , Nothlings U ((2020) ) Dietary factors and neurodegenerative disorders: An umbrella review of meta-analyses of prospective studies. Adv Nutr 11: , 1161–1173. |
[28] | Metcalfe-Roach A , Yu AC , Golz E , Cirstea M , Sundvick K , Kliger D , Foulger LH , Mackenzie M , Finlay BB , Appel-Cresswell S ((2021) ) MIND and Mediterranean diets associated with later onset of Parkinson’s disease. Mov Disord 36: , 977–984. |
[29] | Arellanes IC , Choe N , Solomon V , He X , Kavin B , Martinez AE , Kono N , Buennagel DP , Hazra N , Kim G , D’Orazio LM , McCleary C , Sagare A , Zlokovic BV , Hodis HN , Mack WJ , Chui HC , Harrington MG , Braskie MN , Schneider LS , Yassine HN ((2020) ) Brain delivery of supplemental docosahexaenoic acid (DHA): A randomized placebo-controlled clinical trial. EBioMedicine 59: , 102883. |
[30] | Maltais M , Lorrain D , Léveillé P , Viens I , Vachon A , Houeto A , Presse N , Plourde M ((2022) ) Long-chain Omega-3 fatty acidssupplementation and cognitive performance throughout adulthood: A6-month randomized controlled trial. Prostaglandins LeukotEssent Fatty Acids 178: , 102415. |
[31] | Kivipelto M , Mangialasche F , Ngandu T ((2018) ) Lifestyle interventions to prevent cognitive impairment, dementia and Alzheimer disease. Nat Rev Neurol 14: , 653–666. |
[32] | Tabue-Teguo M , Barreto de Souza P , Cantet C , Andrieu S , Simo N , Fougère B , Dartigues JF , Vellas B ((2018) ) Effect of multidomain intervention, omega-3 polyunsaturated fatty acids supplementation or their combination on cognitive function in non-demented older adults according to frail status: Results from the MAPT Study. J Nutr Health Aging 22: , 923–927. |
[33] | Hoscheidt S , Sanderlin AH , Baker LD , Jung Y , Lockhart S , Kellar D , Whitlow CT , Hanson AJ , Friedman S , Register T , Leverenz JB , Craft S ((2022) ) Mediterranean and Western diet effects on Alzheimer’s disease biomarkers, cerebral perfusion, and cognition in mid-life: A randomized trial. Alzheimers Dement 18: , 457–468. |
[34] | Liu YH , Gao X , Na M , Kris-Etherton PM , Mitchell DC , Jensen GL ((2020) ) Dietary pattern, diet quality, and dementia: A systematic review and meta-analysis of prospective cohort studies. J Alzheimers Dis 78: , 151–168. |
[35] | Dalile B , Kim C , Challinor A , Geurts L , Gibney ER , Galdos MV , La Fata G , Laye S , Mathers JC , Vauzour D , Verkuyl JM , Thuret S ((2022) ) The EAT-Lancet reference diet and cognitive function across the life course. Lancet Planet Health 6: , e749–e759. |
[36] | Yassine HN , Samieri C , Livingston G , Glass K , Wagner M , Tangney C , Plassman BL , Ikram MA , Voigt RM , Gu Y , O’Bryant S , Minihane AM , Craft S , Fink HA , Judd S , Andrieu S , Bowman GL , Richard E , Albensi B , Meyers E , Khosravian S , Solis M , Carrillo M , Snyder H , Grodstein F , Scarmeas N , Schneider LS ((2022) ) Nutrition state of science and dementia prevention: Recommendations of the Nutrition for Dementia Prevention Working Group. Lancet Healthy Longev 3: , e501–e512. |
[37] | Tomaszewski N , He X , Solomon V , Lee M , Mack WJ , Quinn JF , Braskie MN , Yassine HN ((2020) ) Effect of APOE genotype on plasma docosahexaenoic acid (DHA), eicosapentaenoic acid, arachidonic acid, and hippocampal volume in the Alzheimer’s Disease Cooperative Study-Sponsored DHA Clinical Trial. J Alzheimers Dis 74: , 975–990. |
[38] | Heydemann A ((2016) ) An overview of murine high fat diet as a model for type 2 diabetes mellitus. J Diabetes Res 2016: , 2902351. |
[39] | Amelianchik A , Sweetland-Martin L , Norris EH ((2022) ) The effect of dietary fat consumption on Alzheimer’s disease pathogenesis in mouse models. Transl Psychiatry 12: , 293. |
[40] | Astrup A , Magkos F , Bier DM , Brenna JT , de Oliveira Otto MC , Hill JO , King JC , Mente A , Ordovas JM , Volek JS , Yusuf S , Krauss RM ((2020) ) Saturated fats and health: A reassessment and proposal for food-based recommendations: JACC State-of-the-Art Review. J Am Coll Cardiol 76: , 844–857. |
[41] | Sanchez-Alegria K , Arias C ((2022) ) Functional consequences of brain exposure to saturated fatty acids: From energy metabolism and insulin resistance to neuronal damage. Endocrinol Diabetes Metab 6: , e386. |
[42] | González-Becerra K , Ramos-Lopez O , Barrón-Cabrera E , Riezu-Boj JI , Milagro FI , Martínez-López E , Martínez JA ((2019) ) Fatty acids, epigenetic mechanisms and chronic diseases: Asystematic review. Lipids Health Dis 18: , 178. |
[43] | Handelsman Y , Shapiro MD ((2017) ) Triglycerides, atherosclerosis, and cardiovascular outcome studies: Focus on omega-3 fatty acids. Endocr Pract 23: , 100–112. |
[44] | Astrup A , Bertram HC , Bonjour JP , de Groot LC , de Oliveira Otto MC , Feeney EL , Garg ML , Givens I , Kok FJ , Krauss RM , Lamarche B , Lecerf JM , Legrand P , McKinley M , Micha R , Michalski MC , Mozaffarian D , Soedamah-Muthu SS ((2019) ) WHO draft guidelines on dietary saturated and trans fatty acids: Time for a new approach? BMJ 366: , l4137. |
[45] | Arunima S , Rajamohan T ((2014) ) Influence of virgin coconut oil-enriched diet on the transcriptional regulation of fatty acid synthesis and oxidation in rats - a comparative study. Br J Nutr 111: , 1782–1790. |
[46] | Brenna JT , Kothapalli KS ((2014) ) Commentary on ‘Influence of virgin coconut oil-enriched diet on the transcriptional regulation of fatty acid synthesis and oxidation in rats–a comparative study’ by Sakunthala Arunima and Thankappan Rajamohan. Br J Nutr 112: , 1425–1426. |
[47] | Eskelinen MH , Ngandu T , Helkala EL , Tuomilehto J , Nissinen A , Soininen H , Kivipelto M ((2008) ) Fat intake at midlife and cognitive impairment later in life: A population-based CAIDE study. Int J Geriatr Psychiatry 23: , 741–747. |
[48] | Morris MC , Evans DA , Bienias JL , Tangney CC , Wilson RS ((2004) ) Dietary fat intake and 6-year cognitive change in an older biracial community population. Neurology 62: , 1573–1579. |
[49] | Cao GY , Li M , Han L , Tayie F , Yao SS , Huang Z , Ai P , Liu YZ , Hu YH , Xu B ((2019) ) Dietary fat intake and cognitivefunction among older populations: A systematic review and meta-analysis. J Prev Alzheimers Dis 6: , 204–211. |
[50] | Khan NA , Raine LB , Drollette ES , Scudder MR , Hillman CH ((2015) ) The relation of saturated fats and dietary cholesterol to childhood cognitive flexibility. Appetite 93: , 51–56. |
[51] | Kalmijn S , Launer LJ , Ott A , Witteman JC , Hofman A , Breteler MM ((1997) ) Dietary fat intake and the risk of incident dementia in the Rotterdam Study. Ann Neurol 42: , 776–782. |
[52] | Amadieu C , Lefevre-Arbogast S , Delcourt C , Dartigues JF , Helmer C , Feart C , Samieri C ((2017) ) Nutrient biomarker patterns and long-term risk of dementia in older adults. Alzheimers Dement 13: , 1125–1132. |
[53] | Morris MC , Evans DA , Bienias JL , Tangney CC , Bennett DA , Aggarwal N , Schneider J , Wilson RS ((2003) ) Dietary fats and the risk of incident Alzheimer disease. Arch Neurol 60: , 194–200. |
[54] | Otaegui-Arrazola A , Amiano P , Elbusto A , Urdaneta E , Martinez-Lage P ((2014) ) Diet, cognition, and Alzheimer’s disease: Food for thought. Eur J Nutr 53: , 1–23. |
[55] | Barnard ND , Bunner AE , Agarwal U ((2014) ) Saturated and trans fats and dementia: A systematic review. Neurobiol Aging 35 Suppl 2: , S65–73. |
[56] | Solfrizzi V , Panza F , Frisardi V , Seripa D , Logroscino G , Imbimbo BP , Pilotto A ((2011) ) Diet and Alzheimer’s disease risk factors or prevention: The current evidence. Expert Rev. Neurother 11: , 677–708. |
[57] | Morris MC ((2016) ) Nutrition and risk of dementia: Overview and methodological issues. Ann N Y Acad Sci 1367: , 31–37. |
[58] | Prinelli F , Fratiglioni L , Kalpouzos G , Musicco M , Adorni F , Johansson I , Marseglia A , Xu W ((2019) ) Specific nutrient patterns are associated with higher structural brain integrity in dementia-free older adults. Neuroimage 199: , 281–288. |
[59] | Pistollato F , Iglesias RC , Ruiz R , Aparicio S , Crespo J , Lopez LD , Manna PP , Giampieri F , Battino M ((2018) ) Nutritional patterns associated with the maintenance of neurocognitive functions and the risk of dementia and Alzheimer’s disease: A focus on human studies. Pharmacol Res 131: , 32–43. |
[60] | Vannice G , Rasmussen H ((2014) ) Position of the academy of nutrition and dietetics: Dietary fatty acids for healthy adults. J Acad Nutr Diet 114: , 136–153. |
[61] | Mottaghi T , Amirabdollahian F , Haghighatdoost F ((2018) ) Fruit and vegetable intake and cognitive impairment: A systematic review and meta-analysis of observational studies. Eur J Clin Nutr 72: , 1336–1344. |
[62] | Tsurumaki N , Zhang S , Tomata Y , Abe S , Sugawara Y , Matsuyama S , Tsuji I ((2019) ) Fish consumption and risk of incident dementia in elderly Japanese: The Ohsaki cohort 2006 study. Br J Nutr 122: , 1182–1191. |
[63] | Galbete C , Toledo E , Toledo JB , Bes-Rastrollo M , Buil-Cosiales P , Marti A , Guillén-Grima F , Martínez-González MA ((2015) ) Mediterranean diet and cognitive function: The SUN project. JNutr Health Aging 19: , 305–312. |
[64] | van den Brink AC , Brouwer-Brolsma EM , Berendsen AAM , van de Rest O ((2019) ) The Mediterranean, Dietary Approaches to Stop Hypertension (DASH), and Mediterranean-DASH Intervention for Neurodegenerative Delay (MIND) diets are associated with less cognitive decline and a lower risk of Alzheimer’s disease-a review. Adv Nutr 10: , 1040–1065. |
[65] | Ammann EM , Pottala JV , Harris WS , Espeland MA , Wallace R , Denburg NL , Carnahan RM , Robinson JG ((2013) ) ω-3fatty acids and domain-specific cognitive aging: Secondary analyses of data from WHISCA. Neurology 81: , 1484–1491. |
[66] | Chiu CC , Frangou S , Chang CJ , Chiu WC , Liu HC , Sun IW , Liu SI , Lu ML , Chen CH , Huang SY , Dewey ME , Stewart R ((2012) ) Associations between n-3 PUFA concentrations and cognitive function after recovery from late-life depression. Am J Clin Nutr 95: , 420–427. |
[67] | Fiocco AJ , Krieger L , D’Amico D , Parrott MD , Laurin D , Gaudreau P , Greenwood C , Ferland G ((2019) ) A systematic review of existing peripheral biomarkers of cognitive aging: Is there enough evidence for biomarker proxies in behavioral modification interventions?: An initiative in association with the nutrition, exercise and lifestyle team of the Canadian Consortium on Neurodegeneration in Aging. Ageing Res Rev 52: , 72–119. |
[68] | Cisbani G , Bazinet RP ((2021) ) The role of peripheral fatty acids as biomarkers for Alzheimer’s disease and brain inflammation. Prostaglandins Leukot Essent Fatty Acids 164: , 102205. |
[69] | van der Lee SJ , Teunissen CE , Pool R , Shipley MJ , Teumer A , Chouraki V , Melo van Lent D , Tynkkynen J , Fischer K , Hernesniemi J , Haller T , Singh-Manoux A , Verhoeven A , Willemsen G , de Leeuw FA , Wagner H , van Dongen J , Hertel J , Budde K , Willems van Dijk K , Weinhold L , Ikram MA , Pietzner M , Perola M , Wagner M , Friedrich N , Slagboom PE , Scheltens P , Yang Q , Gertzen RE , Egert S , Li S , Hankemeier T , van Beijsterveldt CEM , Vasan RS , Maier W , Peeters CFW , Jörgen Grabe H , Ramirez A , Seshadri S , Metspalu A , Kivimäki M , Salomaa V , Demirkan A , Boomsma DI , van der Flier WM , Amin N , van Duijn CM ((2018) ) Circulating metabolites and general cognitive ability and dementia: Evidence from 11 cohort studies. Alzheimers Dement 14: , 707–722. |
[70] | Bowman GL , Dodge HH , Mattek N , Barbey AK , Silbert LC , Shinto L , Howieson DB , Kaye JA , Quinn JF ((2013) ) Plasmaomega-3 PUFA and white matter mediated executive decline in older adults. Front Aging Neurosci 5: , 92. |
[71] | D’Ascoli TA , Mursu J , Voutilainen S , Kauhanen J , Tuomainen TP , Virtanen JK ((2016) ) Association between serum long-chain omega-3 polyunsaturated fatty acids and cognitive performance in elderly men and women: The Kuopio Ischaemic Heart Disease Risk Factor Study. Eur J Clin Nutr 70: , 970–975. |
[72] | Zamroziewicz MK , Paul EJ , Rubin RD , Barbey AK ((2015) ) Anterior cingulate cortex mediates the relationship between O3PUFAs and executive functions in APOE e4 carriers. Front Aging Neurosci 7: , 87. |
[73] | Leclerc M , Dudonné S , Calon F ((2021) ) Can natural products exertneuroprotection without crossing the blood-brain barrier? Int JMol Sci 22: , 3356. |
[74] | Lacombe RJS , Chouinard-Watkins R , Bazinet RP ((2018) ) Brain docosahexaenoic acid uptake and metabolism. Mol Aspects Med 64: , 109–134. |
[75] | Bianchi VE , Herrera PF , Laura R ((2021) ) Effect of nutrition on neurodegenerative diseases. A systematic review. Nutr Neurosci 24: , 810–834. |
[76] | Jennings A , Cunnane SC , Minihane AM ((2020) ) Can nutrition support healthy cognitive ageing and reduce dementia risk? BMJ 369: , m2269. |
[77] | Vlachos GS , Scarmeas N ((2019) ) Dietary interventions in mild cognitive impairment and dementia. Dialogues Clin Neurosci 21: , 69–82. |
[78] | McGrattan AM , McEvoy CT , McGuinness B , McKinley MC , Woodside JV ((2018) ) Effect of dietary interventions in mild cognitive impairment: A systematic review. Br J Nutr 120: , 1388–1405. |
[79] | Quinn JF , Raman R , Thomas RG , Yurko-Mauro K , Nelson EB , Van Dyck C , Galvin JE , Emond J , Jack CR Jr. , Weiner M , Shinto L , Aisen PS ((2010) ) Docosahexaenoic acid supplementation and cognitive decline in Alzheimer disease: A randomized trial. JAMA 304: , 1903–1911. |
[80] | Frautschy SA , Cole GM ((2011) ) What was lost in translation in the DHA trial is whom you should intend to treat. Alzheimers Res Ther 3: , 2. |
[81] | Livingston G , Huntley J , Sommerlad A , Ames D , Ballard C , Banerjee S , Brayne C , Burns A , Cohen-Mansfield J , Cooper C , Costafreda SG , Dias A , Fox N , Gitlin LN , Howard R , Kales HC , Kivimäki M , Larson EB , Ogunniyi A , Orgeta V , Ritchie K , Rockwood K , Sampson EL , Samus Q , Schneider LS , Selbæk G , Teri L , Mukadam N ((2020) ) Dementiaprevention, intervention, and care: 2020 report of the LancetCommission. Lancet 396: , 413–446. |
[82] | Anastasiou CA , Yannakoulia M , Kontogianni MD , Kosmidis MH , Mamalaki E , Dardiotis E , Hadjigeorgiou G , Sakka P , Tsapanou A , Lykou A , Scarmeas N ((2018) ) Mediterranean lifestyle in relation to cognitive health: Results from the HELIAD Study. Nutrients 10: , 1557. |
[83] | Angeloni C , Businaro R , Vauzour D ((2020) ) The role of diet in preventing and reducing cognitive decline. Curr Opin Psychiatry 33: , 432–438. |
[84] | Andrieu S , Guyonnet S , Coley N , Cantet C , Bonnefoy M , Bordes S , Bories L , Cufi M-N , Dantoine T , Dartigues J-F , Desclaux F , Gabelle A , Gasnier Y , Pesce A , Sudres K , Touchon J , Robert P , Rouaud O , Legrand P , Payoux P , Caubere J-P , Weiner M , Carrié I , Ousset P-J , Vellas B , Vellas B , Guyonnet S , Carrié I , Brigitte L , Faisant C , Lala F , Delrieu J , Villars H , Combrouze E , Badufle C , Zueras A , Andrieu S , Cantet C , Morin C , Van Kan GA , Dupuy C , Rolland Y , Caillaud C , Ousset P-J , Fougère B , Willis S , Belleville S , Gilbert B , Fontaine F , Dartigues J-F , Marcet I , Delva F , Foubert A , Cerda S , Noëlle-Cuffi M , Costes C , Rouaud O , Manckoundia P , Quipourt V , Marilier S , Franon E , Bories L , Pader M-L , Basset M-F , Lapoujade B , Faure V , Li M , Tong Y , Malick-Loiseau C , Cazaban-Campistron E , Desclaux F , Blatge C , Dantoine T , Laubarie-Mouret C , Saulnier I , Clément J-P , Picat M-A , Bernard-Bourzeix L , Willebois S , Désormais I , Cardinaud N , Bonnefoy M , Livet P , Rebaudet P , Gédéon C , Burdet C , Terracol F , Pesce A , Roth S , Chaillou S , Louchart S , Sudres K , Lebrun N , Barro-Belaygues N , Touchon J , Bennys K , Gabelle A , Romano A , Touati L , Marelli C , Pays C , Robert P , Le Duff F , Gervais C , Gonfrier S , Gasnier Y , Bordes S , Begorre D , Carpuat C , Khales K , Lefebvre J-F , El Idrissi SM , Skolil P , Salles J-P , Dufouil C , Lehéricy S , Chupin M , Mangin J-F , Bouhayia A , Allard M , Ricolfi F , Dubois D , Paule M , Martel B , Cotton F , Bonafé A , Chanalet S , Hugon F , Bonneville F , Cognard C , Chollet F , Payoux P , Voisin T , Peiffer S , Hitzel A , Allard M , Zanca M , Monteil J , Darcourt J , Molinier L , Derumeaux H , Costa N , Vincent C , Perret B , Vinel C , Olivier-Abbal P ((2017) ) Effect of long-term omega 3 polyunsaturatedfatty acid supplementation with or without multidomain interventionon cognitive function in elderly adults with memory complaints(MAPT): A randomised, placebo-controlled trial. Lancet Neurol 16: , 377–389. |
[85] | Delrieu J , Payoux P , Carrie I , Cantet C , Weiner M , Vellas B , Andrieu S ((2019) ) Multidomain intervention and/or omega-3 in nondemented elderly subjects according to amyloid status. Alzheimers Dement 15: , 1392–1401. |
[86] | Baker LD , Espeland MA , Kivipelto M , Whitmer RA , Snyder HM , Carrillo MC , Antkowiak S , Chavin M , Cleveland M , Day CE , Desai P , Elbein R , Farias ST , Gitelman D , Katula JA , Lambert K , Leng XI , Lovato L , Morris MC , Ngandu T , Papp KV , Pavlik VN , Raman R , Robertson J , Rushing S , Salloway S , Solomon A , Su J , Ventrelle J , Williams BJ , Williamson JD , Woolard N , Yu M ((2020) ) U.S. Pointer (USA). Alzheimers Dement 16: (Suppl 10), e046951. |
[87] | King A ((2018) ) The search for better animal models of Alzheimer’s disease. Nature 559: , S13–S15. |
[88] | Martini AC , Forner S , Trujillo-Estrada L , Baglietto-Vargas D , LaFerla FM ((2018) ) Past to future: What animal models have taught us about Alzheimer’s disease. J Alzheimers Dis 64: , S365–S378. |
[89] | Oblak AL , Forner S , Territo PR , Sasner M , Carter GW , Howell GR , Sukoff-Rizzo SJ , Logsdon BA , Mangravite LM , Mortazavi A , Baglietto-Vargas D , Green KN , MacGregor GR , Wood MA , Tenner AJ , LaFerla FM , Lamb BT ((2020) ) Model organism development and evaluation for late-onset Alzheimer’s disease: MODEL-AD. Alzheimers Dement (N Y) 6: , e12110. |
[90] | Trujillo-Estrada L , Sanchez-Mejias E , Sanchez-Varo R , Garcia-Leon JA , Nuñez-Diaz C , Davila JC , Vitorica J , LaFerla FM , Moreno-Gonzalez I , Gutierrez A , Baglietto-Vargas D ((2022) ) Animal andcellular models of Alzheimer’s disease: Progress, promise, andfuture approaches. Neuroscientist 28: , 572–593. |
[91] | Gotz J , Bodea LG , Goedert M ((2018) ) Rodent models for Alzheimer disease. Nat Rev Neurosci 19: , 583–598. |
[92] | Scearce-Levie K , Sanchez PE , Lewcock JW ((2020) ) Leveraging preclinical models for the development of Alzheimer disease therapeutics. Nat Rev Drug Discov 19: , 447–462. |
[93] | Oakley H , Cole SL , Logan S , Maus E , Shao P , Craft J , Guillozet-Bongaarts A , Ohno M , Disterhoft J , Van Eldik L , Berry R , Vassar R ((2006) ) Intraneuronal beta-amyloid aggregates, neurodegeneration, and neuron loss in transgenic mice with five familial Alzheimer’s disease mutations: Potential factors in amyloid plaque formation. J Neurosci 26: , 10129–10140. |
[94] | Grueninger F , Bohrmann B , Czech C , Ballard TM , Frey JR , Weidensteiner C , von Kienlin M , Ozmen L ((2010) ) Phosphorylation of tau at S422 is enhanced by Abeta in TauPS2APP triple transgenic mice. Neurobiol Dis 37: , 294–306. |
[95] | Frautschy SA , Yang F , Calderón L , Cole GM ((1996) ) Rodent models of Alzheimer’s disease: Rat A beta infusion approaches to amyloid deposits. Neurobiol Aging 17: , 311–321. |
[96] | Hsiao K , Chapman P , Nilsen S , Eckman C , Harigaya Y , Younkin S , Yang F , Cole G ((1996) ) Correlative memory deficits, Aβ elevation, and amyloid plaques in transgenic mice. Science 274: , 99–102. |
[97] | Radde R , Bolmont T , Kaeser SA , Coomaraswamy J , Lindau D , Stoltze L , Calhoun ME , Jäggi F , Wolburg H , Gengler S , Haass C , Ghetti B , Czech C , Hölscher C , Mathews PM , Jucker M ((2006) ) Abeta42-driven cerebral amyloidosis in transgenic mice reveals early and robust pathology. EMBO Rep 7: , 940–946. |
[98] | Oddo S , Caccamo A , Shepherd JD , Murphy MP , Golde TE , Kayed R , Metherate R , Mattson MP , Akbari Y , LaFerla FM ((2003) ) Triple-transgenic model of Alzheimer’s disease with plaques and tangles. Neuron 39: , 409–421. |
[99] | Esquerda-Canals G , Montoliu-Gaya L , Güell-Bosch J , Villegas S ((2017) ) Mouse models of Alzheimer’s disease. J Alzheimers Dis 57: , 1171–1183. |
[100] | Mullane K , Williams M ((2019) ) Preclinical models of Alzheimer’s disease: Relevance and translational validity. Curr Protoc Pharmacol 84: , e57. |
[101] | Zeiss CJ ((2020) ) Utility of spontaneous animal models of Alzheimer’s disease in preclinical efficacy studies. Cell Tissue Res 380: , 273–286. |
[102] | Games D , Adams D , Alessandrini R , Barbour R , Berthelette P , Blackwell C , Carr T , Clemens J , Donaldson T , Gillespie F , Guido T , Hagopian S , Johnson-Wood K , Khan K , Lee M , Leibowitz P , Lieberburg I , Little S , Masliah E , McConlogue L , Montoya-Zavala M , Mucke L , Paganini L , Penniman E , Power M , Schenk D , Seubert P , Snyder B , Soriano F , Tan H , Vitale J , Wadsworth S , Wolozin B , Zhao J ((1995) ) Alzheimer-type neuropathology in transgenic mice overexpressing V717F beta-amyloid precursor protein. Nature 373: , 523–527. |
[103] | Rockenstein EM , McConlogue L , Tan H , Power M , Masliah E , Mucke L ((1995) ) Levels and alternative splicing of amyloid beta protein precursor (APP) transcripts in brains of APP transgenic mice and humans with Alzheimer’s disease. J Biol Chem 270: , 28257–28267. |
[104] | Hartman RE , Izumi Y , Bales KR , Paul SM , Wozniak DF , Holtzman DM ((2005) ) Treatment with an amyloid-beta antibody ameliorates plaque load, learning deficits, and hippocampal long-term potentiation in a mouse model of Alzheimer’s disease. J Neurosci 25: , 6213–6220. |
[105] | Dodart JC , Meziane H , Mathis C , Bales KR , Paul SM , Ungerer A ((1999) ) Behavioral disturbances in transgenic mice overexpressing the V717F beta-amyloid precursor protein. Behav Neurosci 113: , 982–990. |
[106] | Irizarry MC , McNamara M , Fedorchak K , Hsiao K , Hyman BT ((1997) ) APPSw transgenic mice develop age-related A beta deposits and neuropil abnormalities, but no neuronal loss in CA1. J Neuropathol Exp Neurol 56: , 965–973. |
[107] | Mucke L , Masliah E , Yu GQ , Mallory M , Rockenstein EM , Tatsuno G , Hu K , Kholodenko D , Johnson-Wood K , McConlogue L ((2000) ) High-level neuronal expression of abeta 1-42 in wild-type human amyloid protein precursor transgenic mice: Synaptotoxicity without plaque formation. J Neurosci 20: , 4050–4058. |
[108] | Sasaguri H , Nilsson P , Hashimoto S , Nagata K , Saito T , De Strooper B , Hardy J , Vassar R , Winblad B , Saido TC ((2017) ) APP mouse models for Alzheimer’s disease preclinical studies. EMBO J 36: , 2473–2487. |
[109] | Wirths O , Zampar S ((2020) ) Neuron loss in Alzheimer’s disease: Translation in transgenic mouse models. Int J Mol Sci 21: , 8144. |
[110] | Kitazawa M , Medeiros R , LaFerla FM ((2012) ) Transgenic mouse models of Alzheimer’s disease: Developing a better model as a tool for therapeutic interventions. Curr Pharm Des 18: , 1131–1147. |
[111] | Lewis J , McGowan E , Rockwood J , Melrose H , Nacharaju P , Van Slegtenhorst M , Gwinn-Hardy K , Paul Murphy M , Baker M , Yu X , Duff K , Hardy J , Corral A , Lin WL , Yen SH , Dickson DW , Davies P , Hutton M ((2000) ) Neurofibrillary tangles, amyotrophy and progressive motor disturbance in mice expressing mutant (P301L) tau protein. Nat Genet 25: , 402–405. |
[112] | Myers A , McGonigle P ((2019) ) Overview of transgenic mouse models for Alzheimer’s disease. Curr Protoc Neurosci 89: , e81. |
[113] | Andorfer C , Kress Y , Espinoza M , de Silva R , Tucker KL , Barde YA , Duff K , Davies P ((2003) ) Hyperphosphorylation and aggregation of tau in mice expressing normal human tau isoforms. J Neurochem 86: , 582–590. |
[114] | Belfiore R , Rodin A , Ferreira E , Velazquez R , Branca C , Caccamo A , Oddo S ((2019) ) Temporal and regional progression of Alzheimer’s disease-like pathology in 3xTg-AD mice. Aging Cell 18: , e12873. |
[115] | Dennison JL , Ricciardi NR , Lohse I , Volmar CH , Wahlestedt C ((2021) ) Sexual dimorphism in the 3xTg-AD mouse model and its impact on pre-clinical research. J Alzheimers Dis 80: , 41–52. |
[116] | Stover KR , Campbell MA , Van Winssen CM , Brown RE ((2015) ) Early detection of cognitive deficits in the 3xTg-AD mouse model of Alzheimer’s disease. Behav Brain Res 289: , 29–38. |
[117] | Vandal M , White PJ , Chevrier G , Tremblay C , St-Amour I , Planel E , Marette A , Calon F ((2015) ) Age-dependent impairment of glucose tolerance in the 3xTg-AD mouse model of Alzheimer’s disease. FASEB J 29: , 4273–4284. |
[118] | Bories C , Guitton MJ , Julien C , Tremblay C , Vandal M , Msaid M , De Koninck Y , Calon F ((2012) ) Sex-dependent alterations in social behaviour and cortical synaptic activity coincide at different ages in a model of Alzheimer’s disease. PLoS One 7: , e46111. |
[119] | Arsenault D , Tremblay C , Emond V , Calon F ((2020) ) Sex-dependent alterations in the physiology of entorhinal cortex neurons in old heterozygous 3xTg-AD mice. Biol Sex Differ 11: , 63. |
[120] | Barron AM , Rosario ER , Elteriefi R , Pike CJ ((2013) ) Sex-specific effects of high fat diet on indices of metabolic syndrome in 3xTg-AD mice: Implications for Alzheimer’s disease. PLoS One 8: , e78554. |
[121] | Clinton LK , Billings LM , Green KN , Caccamo A , Ngo J , Oddo S , McGaugh JL , LaFerla FM ((2007) ) Age-dependent sexual dimorphism in cognition and stress response in the 3xTg-AD mice. Neurobiol Dis 28: , 76–82. |
[122] | Saito T , Matsuba Y , Mihira N , Takano J , Nilsson P , Itohara S , Iwata N , Saido TC ((2014) ) Single App knock-in mouse models of Alzheimer’s disease. Nat Neurosci 17: , 661–663. |
[123] | LaFerla FM , Green KN ((2012) ) Animal models of Alzheimer disease. Cold Spring Harb Perspect Med 2: , a006320. |
[124] | Mehla J , Lacoursiere SG , Lapointe V , McNaughton BL , Sutherland RJ , McDonald RJ , Mohajerani MH ((2019) ) Age-dependent behavioral and biochemical characterization of single APP knock-in mouse (APP(NL-G-F/NL-G-F)) model of Alzheimer’s disease. Neurobiol Aging 75: , 25–37. |
[125] | Baglietto-Vargas D , Forner S , Cai L , Martini AC , Trujillo-Estrada L , Swarup V , Nguyen MMT , Do Huynh K , Javonillo DI , Tran KM , Phan J , Jiang S , Kramár EA , Nuñez-Diaz C , Balderrama-Gutierrez G , Garcia F , Childs J , Rodriguez-Ortiz CJ , Garcia-Leon JA , Kitazawa M , Shahnawaz M , Matheos DP , Ma X , Da Cunha C , Walls KC , Ager RR , Soto C , Gutierrez A , Moreno-Gonzalez I , Mortazavi A , Tenner AJ , MacGregor GR , Wood M , Green KN , LaFerla FM ((2021) ) Generation of a humanizedAβ expressing mouse demonstrating aspects of Alzheimer’sdisease-like pathology. Nat Commun 12: , 2421. |
[126] | De Strooper B , Simons M , Multhaup G , Van Leuven F , Beyreuther K , Dotti CG ((1995) ) Production of intracellular amyloid-containing fragments in hippocampal neurons expressing human amyloid precursor protein and protection against amyloidogenesis by subtle amino acid substitutions in the rodent sequence. EMBO J 14: , 4932–4938. |
[127] | Foley KE , Hewes AA , Garceau DT , Kotredes KP , Carter GW , Sasner M , Howell GR ((2022) ) The APOE (epsilon3/epsilon4) genotype drives distinct gene signatures in the cortex of young mice. Front Aging Neurosci 14: , 838436. |
[128] | Oblak AL , Kotredes KP , Pandey RS , Reagan AM , Ingraham C , Perkins B , Lloyd C , Baker D , Lin PB , Soni DM , Tsai AP , Persohn SA , Bedwell AA , Eldridge K , Speedy R , Meyer JA , Peters JS , Figueiredo LL , Sasner M , Territo PR , Sukoff Rizzo SJ , Carter GW , Lamb BT , Howell GR ((2022) ) Plcg2(M28L) interacts with high fat/high sugar diet to accelerate Alzheimer’s disease-relevant phenotypes in mice. Front Aging Neurosci 14: , 886575. |
[129] | Sullivan PM , Mezdour H , Aratani Y , Knouff C , Najib J , Reddick RL , Quarfordt SH , Maeda N ((1997) ) Targeted replacement of the mouse apolipoprotein E gene with the common human APOE3 allele enhances diet-induced hypercholesterolemia and atherosclerosis. J Biol Chem 272: , 17972–17980. |
[130] | Lewandowski CT , Maldonado Weng J , LaDu MJ ((2020) ) Alzheimer’s disease pathology in APOE transgenic mouse models: The who, what, when, where, why, and how. Neurobiol Dis 139: , 104811. |
[131] | Tesseur I , Van Dorpe J , Spittaels K , Van den Haute C , Moechars D , Van Leuven F ((2000) ) Expression of human apolipoprotein E4 in neurons causes hyperphosphorylation of protein tau in the brains of transgenic mice. Am J Pathol 156: , 951–964. |
[132] | Traversy MT , Vandal M , Tremblay C , Tournissac M , Giguère-Rancourt A , Bennett AD , Calon F ((2017) ) Altered cerebral insulin response in transgenic mice expressing the epsilon-4 allele of the human apolipoprotein E gene. Psychoneuroendocrinology 77: , 203–210. |
[133] | Graybeal JJ , Bozzelli PL , Graybeal LL , Groeber CM , McKnight PE , Cox DN , Flinn JM ((2015) ) Human ApoEɛ4 alters circadian rhythm activity, IL-1β, and GFAP in CRND8 mice. J Alzheimers Dis 43: , 823–834. |
[134] | Storck SE , Meister S , Nahrath J , Meißner JN , Schubert N , Di Spiezio A , Baches S , Vandenbroucke RE , Bouter Y , Prikulis I , Korth C , Weggen S , Heimann A , Schwaninger M , Bayer TA , Pietrzik CU ((2016) ) Endothelial LRP1 transports amyloid-β(1-42) across the blood-brain barrier. J Clin Invest 126: , 123–136. |
[135] | Storck SE , Kurtyka M , Pietrzik CU ((2021) ) Brain endothelial LRP1 maintains blood-brain barrier integrity. Fluids Barriers CNS 18: , 27. |
[136] | Frautschy SA , Baird A , Coleo GM ((1991) ) Effects of injected Alzheimer beta-amyloid cores in rat brain. Proc Natl Acad Sci U S A 88: , 8362–8366. |
[137] | Jaya Prasanthi RP , Schommer E , Thomasson S , Thompson A , Feist G , Ghribi O ((2008) ) Regulation of beta-amyloid levels in the brain of cholesterol-fed rabbit, a model system for sporadic Alzheimer’s disease. Mech Ageing Dev 129: , 649–655. |
[138] | Martino Adami PV , Galeano P , Wallinger ML , Quijano C , Rabossi A , Pagano ES , Olivar N , Reyes Toso C , Cardinali D , Brusco LI , Do Carmo S , Radi R , Gevorkian G , Castano EM , Cuello AC , Morelli L ((2017) ) Worsening of memory deficit induced by energy-dense diet in a rat model of early-Alzheimer’s disease is associated to neurotoxic Abeta species and independent of neuroinflammation. Biochim Biophys Acta Mol Basis Dis 1863: , 731–743. |
[139] | Denver P , D’Adamo H , Hu S , Zuo X , Zhu C , Okuma C , Kim P , Castro D , Jones MR , Leal C , Mekkittikul M , Ghadishah E , Teter B , Vinters HV , Cole GM , Frautschy SA ((2019) ) A novel model of mixed vascular dementia incorporating hypertension in a rat model of Alzheimer’s disease. Front Physiol 10: , 1269. |
[140] | Virgili J , Lebbadi M , Tremblay C , St-Amour I , Pierrisnard C , Faucher-Genest A , Emond V , Julien C , Calon F ((2018) ) Characterization of a 3xTg-AD mouse model of Alzheimer’s disease with the senescence accelerated mouse prone 8 (SAMP8) background. Synapse 72: doi: 10.1002/syn.22025. |
[141] | Sun M , McDonald SJ , Brady RD , Collins-Praino L , Yamakawa GR , Monif M , O’Brien TJ , Cloud GC , Sobey CG , Mychasiuk R , Loane DJ , Shultz SR ((2020) ) The need to incorporate aged animals into the preclinical modeling of neurological conditions. Neurosci Biobehav Rev 109: , 114–128. |
[142] | Last AR , Wilson SA ((2006) ) Low-carbohydrate diets. Am Fam Physician 73: , 1942–1948. |
[143] | Speakman JR ((2019) ) Use of high-fat diets to study rodent obesity as a model of human obesity. Int J Obes (Lond) 43: , 1491–1492. |
[144] | Lu M , Wan Y , Yang B , Huggins CE , Li D ((2018) ) Effects of low-fat compared with high-fat diet on cardiometabolic indicators in people with overweight and obesity without overt metabolic disturbance: A systematic review and meta-analysis of randomised controlled trials. Br J Nutr 119: , 96–108. |
[145] | Hintze KJ , Benninghoff AD , Cho CE , Ward RE ((2018) ) Modeling the western diet for preclinical investigations. Adv Nutr 9: , 263–271. |
[146] | Chobot A , Górowska-Kowolik K , Sokołowska M , Jarosz-Chobot P ((2018) ) Obesity and diabetes-Not only a simple link between twoepidemics. Diabetes Metab Res Rev 34: , e3042. |
[147] | Whitmer RA , Gustafson DR , Barrett-Connor E , Haan MN , Gunderso EP , Yaffe K ((2008) ) Central obesity and increasedrisk of dementia more than three decades later. Neurology 71: , 1057–1064. |
[148] | Profenno LA , Porsteinsson AP , Faraone SV ((2010) ) Meta-analysis of Alzheimer’s disease risk with obesity, diabetes, and related disorders. Biol Psychiatry 67: , 505–512. |
[149] | Luchsinger JA , Tang MX , Shea S , Mayeux R ((2002) ) Caloric intake and the risk of Alzheimer disease. Arch Neurol 59: , 1258–1263. |
[150] | Prickett C , Brennan L , Stolwyk R ((2015) ) Examining the relationship between obesity and cognitive function: A systematic literature review. Obes Res Clin Pract 9: , 93–113. |
[151] | Edwards Iii GA , Gamez N , Escobedo G Jr. , Calderon O , Moreno-Gonzalez I ((2019) ) Modifiable risk factors for Alzheimer’s disease. Front Aging Neurosci 11: , 146. |
[152] | Rasmussen Eid H , Rosness TA , Bosnes O , Salvesen Ø , Knutli M , Stordal E ((2019) ) Smoking and obesity as risk factors in frontotemporal dementia and Alzheimer’s disease: The HUNT Study. Dement Geriatr Cogn Dis Extra 9: , 1–10. |
[153] | Silva MVF , Loures CMG , Alves LCV , de Souza LC , Borges KBG , Carvalho MDG ((2019) ) Alzheimer’s disease: Risk factors and potentially protective measures. J Biomed Sci 26: , 33. |
[154] | Jagust W , Harvey D , Mungas D , Haan M ((2005) ) Central obesity and the aging brain. Arch Neurol 62: , 1545–1548. |
[155] | De Sousa RAL , Harmer AR , Freitas DA , Mendonca VA , Lacerda ACR , Leite HR ((2020) ) An update on potential links between type 2 diabetes mellitus and Alzheimer’s disease. Mol Biol Rep 47: , 6347–6356. |
[156] | Arvanitakis Z , Wang HY , Capuano AW , Khan A , Taïb B , Anokye-Danso F , Schneider JA , Bennett DA , Ahima RS , Arnold SE ((2020) ) Brain insulin signaling, Alzheimer disease pathology, and cognitivefunction. Ann Neurol 88: , 513–525. |
[157] | Talbot K , Wang HY , Kazi H , Han LY , Bakshi KP , Stucky A , Fuino RL , Kawaguchi KR , Samoyedny AJ , Wilson RS , Arvanitakis Z , Schneider JA , Wolf BA , Bennett DA , Trojanowski JQ , Arnold SE ((2012) ) Demonstrated brain insulin resistance in Alzheimer’s disease patients is associated with IGF-1 resistance, IRS-1 dysregulation, and cognitive decline. J Clin Invest 122: , 1316–1338. |
[158] | Moran C , Beare R , Wang W , Callisaya M , Srikanth V , Alzheimer’s Disease Neuroimaging Initiative ((2019) ) Type 2 diabetes mellitus, brain atrophy, and cognitive decline. Neurology 92: , e823–e830. |
[159] | Arnold SE , Arvanitakis Z , Macauley-Rambach SL , Koenig AM , Wang HY , Ahima RS , Craft S , Gandy S , Buettner C , Stoeckel LE , Holtzman DM , Nathan DM ((2018) ) Brain insulin resistance in type 2 diabetes and Alzheimer disease: Concepts and conundrums. Nat Rev Neurol 14: , 168–181. |
[160] | Kosyreva AM , Sentyabreva AV , Tsvetkov IS , Makarova OV ((2022) ) Alzheimer’s disease and inflammaging. Brain Sci 12: , 1237. |
[161] | Bae HR , Shin SK , Yoo JH , Kim S , Young HA , Kwon EY ((2023) ) Chronic inflammation in high-fat diet-fed mice: Unveiling the early pathogenic connection between liver and adipose tissue. J Autoimmun 139: , 103091. |
[162] | Szarc vel Szic K , Declerck K , Vidakovic M , Vanden Berghe W ((2015) ) From inflammaging to healthy aging by dietary lifestyle choices: Is epigenetics the key to personalized nutrition? Clin Epigenetics 7: , 33. |
[163] | Fulop T , Larbi A , Dupuis G , Le Page A , Frost EH , Cohen AA , Witkowski JM , Franceschi C ((2017) ) Immunosenescence and inflamm-aging as two sides of the same coin:Friends or foes? Front Immunol 8: , 1960. |
[164] | Fulop T , Larbi A , Witkowski JM ((2019) ) Human inflammaging. Gerontology 65: , 495–504. |
[165] | Franceschi C , Bonafe M , Valensin S , Olivieri F , De Luca M , Ottaviani E , De Benedictis G ((2000) ) Inflamm-aging. An evolutionary perspective on immunosenescence. Ann N Y Acad Sci 908: , 244–254. |
[166] | Yanguas-Casas N , Torres C , Crespo-Castrillo A , Diaz-Pacheco S , Healy K , Stanton C , Chowen JA , Garcia-Segura LM , Arevalo MA , Cryan JF , de Ceballos ML ((2021) ) High-fat diet alters stress behavior, inflammatory parameters and gut microbiota in Tg APP mice in a sex-specific manner. Neurobiol Dis 159: , 105495. |
[167] | Abedi A , Foroutan T , Mohaghegh Shalmani L , Dargahi L ((2023) ) Sex-specific effects of high-fat diet on rat brain glucose metabolism and early-onset dementia symptoms. Mech Ageing Dev 211: , 111795. |
[168] | Freire-Regatillo A , Diaz-Pacheco S , Frago LM , Arévalo M , Argente J , Garcia-Segura LM , de Ceballos ML , Chowen JA ((2022) ) Sexdifferences in hypothalamic changes and the metabolic response ofTgAPP mice to a high fat diet. Front Neuroanat 16: , 910477. |
[169] | Mattar JM , Majchrzak M , Iannucci J , Bartman S , Robinson JK , Grammas P ((2022) ) Sex differences in metabolic indices and chronic neuroinflammation in response to prolonged high-fat diet in ApoE4 knock-in mice. Int J Mol Sci 23: , 3921. |
[170] | Landau SM , Harvey D , Madison CM , Koeppe RA , Reiman EM , Foster NL , Weiner MW , Jagust WJ ((2011) ) Associations between cognitive, functional, and FDG-PET measures of decline in AD and MCI. Neurobiol Aging 32: , 1207–1218. |
[171] | Mosconi L , Mistur R , Switalski R , Tsui WH , Glodzik L , Li Y , Pirraglia E , De Santi S , Reisberg B , Wisniewski T , de Leon MJ ((2009) ) FDG-PET changes in brain glucose metabolism from normal cognition to pathologically verified Alzheimer’s disease. Eur J Nucl Med Mol Imaging 36: , 811–822. |
[172] | Edison P , Archer HA , Hinz R , Hammers A , Pavese N , Tai YF , Hotton G , Cutler D , Fox N , Kennedy A , Rossor M , Brooks DJ ((2007) ) Amyloid, hypometabolism, and cognition in Alzheimer disease: An [11C]PIB and [18F]FDG PET study. Neurology 68: , 501–508. |
[173] | Iaccarino L , Sala A , Caminiti SP , Perani D ((2017) ) The emerging role of PET imaging in dementia. F1000Res 6: , 1830. |
[174] | Mosconi L ((2013) ) Glucose metabolism in normal aging and Alzheimer’s disease: Methodological and physiological considerations for PET studies. Clin Transl Imaging 1: , 10.1007/s40336-013-0026-y. |
[175] | Hammond TC , Xing X , Wang C , Ma D , Nho K , Crane PK , Elahi F , Ziegler DA , Liang G , Cheng Q , Yanckello LM , Jacobs N , Lin AL ((2020) ) beta-amyloid and tau drive early Alzheimer’s disease decline while glucose hypometabolism drives late decline. Commun Biol 3: , 352. |
[176] | Butterfield DA , Halliwell B ((2019) ) Oxidative stress, dysfunctional glucose metabolism and Alzheimer disease. Nat Rev Neurosci 20: , 148–160. |
[177] | Wijesekara N , Goncalves RA , De Felice FG , Fraser PE ((2018) ) Impaired peripheral glucose homeostasis and Alzheimer’s disease. Neuropharmacology 136: , 172–181. |
[178] | Vandal M , White PJ , Tremblay C , St-Amour I , Chevrier G , Emond V , Lefrancois D , Virgili J , Planel E , Giguere Y , Marette A , Calon F ((2014) ) Insulin reverses the high-fat diet-induced increase in brain Abeta and improves memory in an animal model of Alzheimer disease. Diabetes 63: , 4291–4301. |
[179] | Crane PK , Walker R , Hubbard RA , Li G , Nathan DM , Zheng H , Haneuse S , Craft S , Montine TJ , Kahn SE , McCormick W , McCurry SM , Bowen JD , Larson EB ((2013) ) Glucose levels and risk of dementia. N Engl J Med 369: , 540–548. |
[180] | Matsuzaki T , Sasaki K , Tanizaki Y , Hata J , Fujimi K , Matsui Y , Sekita A , Suzuki SO , Kanba S , Kiyohara Y , Iwaki T ((2010) ) Insulin resistance is associated with the pathology of Alzheimer disease: The Hisayama study. Neurology 75: , 764–770. |
[181] | Tournissac M , Vu TM , Vrabic N , Hozer C , Tremblay C , Melancon K , Planel E , Pifferi F , Calon F ((2021) ) Repurposing beta-3 adrenergic receptor agonists for Alzheimer’s disease: Beneficial effects in a mouse model. Alzheimers Res Ther 13: , 103. |
[182] | Pedro PF , Tsakmaki A , Bewick GA ((2020) ) The glucose tolerance test in mice. Methods Mol Biol 2128: , 207–216. |
[183] | Durand R , Ouellette A , Houde VP , Guenard F , Varin TV , Marcotte B , Pilon G , Fraboulet E , Vohl MC , Marette A , Bazinet L ((2020) ) Animal and cellular studies demonstrate some of the beneficial impacts of herring milt hydrolysates on obesity-induced glucose intolerance and inflammation. Nutrients 12: , 3235. |
[184] | Valle M , St-Pierre P , Pilon G , Marette A ((2020) ) Differential effects of chronic ingestion of refined sugars versus natural sweeteners on insulin resistance and hepatic steatosis in a rat model of diet-induced obesity. Nutrients 12: , 2292. |
[185] | Huang J , Lai JHC , Han X , Chen Z , Xiao P , Liu Y , Chen L , Xu J , Chan KWY ((2022) ) Sensitivity schemes for dynamic glucose-enhanced magnetic resonance imaging to detect glucose uptake and clearance in mouse brain at 3 T. NMR Biomed 35: , e4640. |
[186] | Tolomeo D , Micotti E , Serra SC , Chappell M , Snellman A , Forloni G ((2018) ) Chemical exchange saturation transfer MRI shows low cerebral 2-deoxy-D-glucose uptake in a model of Alzheimer’s disease. Sci Rep 8: , 9576. |
[187] | Chen L , Wei Z , Chan KW , Li Y , Suchal K , Bi S , Huang J , Xu X , Wong PC , Lu H , van Zijl PC , Li T , Xu J ((2021) ) D-Glucose uptake and clearance in the tauopathy Alzheimer’s disease mouse brain detected by on-resonance variable delay multiple pulse MRI. J Cereb Blood Flow Metab 41: , 1013–1025. |
[188] | Huang J , van Zijl PCM , Han X , Dong CM , Cheng GWY , Tse KH , Knutsson L , Chen L , Lai JHC , Wu EX , Xu J , Chan KWY ((2020) ) Altered d-glucose in brain parenchyma and cerebrospinal fluid of early Alzheimer’s disease detected bydynamic glucose-enhanced MRI. , eaba. Sci Adv 6: , 3884. |
[189] | Maesako M , Uemura K , Kubota M , Kuzuya A , Sasaki K , Asada M , Watanabe K , Hayashida N , Ihara M , Ito H , Shimohama S , Kihara T , Kinoshita A ((2012) ) Environmental enrichment ameliorated high-fat diet-induced Abeta deposition and memory deficit in APP transgenic mice. Neurobiol Aging 33: , 1011.e11–23. |
[190] | Maesako M , Uemura K , Kubota M , Kuzuya A , Sasaki K , Hayashida N , Asada-Utsugi M , Watanabe K , Uemura M , Kihara T , Takahashi R , Shimohama S , Kinoshita A ((2012) ) Exercise is more effective than diet control in preventing high fat diet-induced beta-amyloid deposition and memory deficit in amyloid precursor protein transgenic mice. J Biol Chem 287: , 23024–23033. |
[191] | Herculano B , Tamura M , Ohba A , Shimatani M , Kutsuna N , Hisatsune T ((2013) ) beta-alanyl-L-histidine rescues cognitive deficits caused by feeding a high fat diet in a transgenic mouse model of Alzheimer’s disease. J Alzheimers Dis 33: , 983–997. |
[192] | Walker JM , Dixit S , Saulsberry AC , May JM , Harrison FE ((2017) ) Reversal of high fat diet-induced obesity improves glucose tolerance, inflammatory response, beta-amyloid accumulation and cognitive decline in the APP/PSEN1 mouse model of Alzheimer’s disease. Neurobiol Dis 100: , 87–98. |
[193] | Ho L , Qin W , Pompl PN , Xiang Z , Wang J , Zhao Z , Peng Y , Cambareri G , Rocher A , Mobbs CV , Hof PR , Pasinetti GM ((2004) ) Diet-induced insulin resistance promotes amyloidosis in a transgenic mouse model of Alzheimer’s disease. FASEB J 18: , 902–904. |
[194] | de Bem AF , Krolow R , Farias HR , de Rezende VL , Gelain DP , Moreira JCF , Duarte J , de Oliveira J ((2020) ) Animal models of metabolic disorders in the study of neurodegenerative diseases: An overview. Front Neurosci 14: , 604150. |
[195] | Knight EM , Martins IV , Gumusgoz S , Allan SM , Lawrence CB ((2014) ) High-fat diet-induced memory impairment in triple-transgenic Alzheimer’s disease (3xTgAD) mice is independent of changes in amyloid and tau pathology. Neurobiol Aging 35: , 1821–1832. |
[196] | Spinelli M , Fusco S , Grassi C ((2019) ) Brain insulin resistance and hippocampal plasticity: Mechanisms and biomarkers of cognitive decline. Front Neurosci 13: , 788. |
[197] | Vandal M , Bourassa P , Calon F ((2015) ) Can insulin signaling pathways be targeted to transport Abeta out of the brain? Front Aging Neurosci 7: , 114. |
[198] | Shieh JC , Huang PT , Lin YF ((2020) ) Alzheimer’s disease and diabetes: Insulin signaling as the bridge linking two pathologies. Mol Neurobiol 57: , 1966–1977. |
[199] | Berlanga-Acosta J , Guillén-Nieto G , Rodríguez-Rodríguez N , Bringas-Vega ML , García-Del-Barco-Herrera D , Berlanga-Saez JO , García-Ojalvo A , Valdés-Sosa MJ , Valdés-Sosa PA ((2020) ) Insulin resistance at the crossroad of Alzheimer disease pathology:A review. Front Endocrinol (Lausanne) 11: , 560375. |
[200] | Kellar D , Craft S ((2020) ) Brain insulin resistance in Alzheimer’s disease and related disorders: Mechanisms and therapeutic approaches. Lancet Neurol 19: , 758–766. |
[201] | Lee JH , Jahrling JB , Denner L , Dineley KT ((2018) ) Targeting insulin for Alzheimer’s disease: Mechanisms, status and potential directions. J Alzheimers Dis 64: , S427–s453. |
[202] | Leclerc M , Bourassa P , Tremblay C , Caron V , Sugere C , Emond V , Bennett DA , Calon F ((2023) ) Cerebrovascular insulin receptors are defective in Alzheimer’s disease. Brain 146: , 75–90. |
[203] | Kellar D , Register T , Lockhart SN , Aisen P , Raman R , Rissman RA , Brewer J , Craft S ((2022) ) Intranasal insulin modulates cerebrospinal fluid markers of neuroinflammation in mild cognitive impairment and Alzheimer’s disease: A randomized trial. Sci Rep 12: , 1346. |
[204] | Craft S , Claxton A , Baker LD , Hanson AJ , Cholerton B , Trittschuh EH , Dahl D , Caulder E , Neth B , Montine TJ , Jung Y , Maldjian J , Whitlow C , Friedman S ((2017) ) Effects of regular and long-acting insulin on cognition and Alzheimer’s disease biomarkers: A pilot clinical trial. J Alzheimers Dis 57: , 1325–1334. |
[205] | Brunner YF , Kofoet A , Benedict C , Freiherr J ((2015) ) Central insulin administration improves odor-cued reactivation of spatial memory in young men. J Clin Endocrinol Metab 100: , 212–219. |
[206] | Claxton A , Baker LD , Hanson A , Trittschuh EH , Cholerton B , Morgan A , Callaghan M , Arbuckle M , Behl C , Craft S ((2015) ) Long-acting intranasal insulin detemir improves cognition for adults with mild cognitive impairment or early-stage Alzheimer’s disease dementia. J Alzheimers Dis 44: , 897–906. |
[207] | Mather KJ , Tjaden AH , Hoehn A , Nadeau KJ , Buchanan TA , Kahn SE , Arslanian SA , Caprio S , Atkinson KM , Cree-Green M , Utzschneider KM , Edelstein SL ((2021) ) Precision and accuracy of hyperglycemic clamps in a multicenter study. , . Am J Physiol Endocrinol Metab 320: , E797–e807. |
[208] | Charbonneau A , Marette A ((2010) ) Inducible nitric oxide synthase induction underlies lipid-induced hepatic insulin resistance in mice: Potential role of tyrosine nitration of insulin signaling proteins. Diabetes 59: , 861–871. |
[209] | Kim JK ((2009) ) Hyperinsulinemic-euglycemic clamp to assess insulin sensitivity. Methods Mol Biol 560: , 221–238. |
[210] | Cózar-Castellano I , Perdomo G ((2020) ) Assessment of insulin tolerance in mice. Methods Mol Biol 2128: , 217–224. |
[211] | Muniyappa R , Lee S , Chen H , Quon MJ ((2008) ) Current approaches for assessing insulin sensitivity and resistance: Advantages, limitations, and appropriate usage. Am J Physiol Endocrinol Metab 294: , E15–26. |
[212] | Wallace TM , Levy JC , Matthews DR ((2004) ) Use and abuse of HOMA modeling. Diabetes Care 27: , 1487–1495. |
[213] | Leighton E , Sainsbury CA , Jones GC ((2017) ) A practical review of C-peptide testing in diabetes. Diabetes Ther 8: , 475–487. |
[214] | McGuinness OP , Ayala JE , Laughlin MR , Wasserman DH ((2009) ) NIH experiment in centralized mouse phenotyping: The Vanderbilt experience and recommendations for evaluating glucose homeostasis in the mouse. , E. Am J Physiol Endocrinol Metab 297: , 849–855. |
[215] | Chen Y , Feng R , Wang H , Wei R , Yang J , Wang L , Wang H , Zhang L , Hong TP , Wen J ((2014) ) High-fat diet induces early-onset diabetes in heterozygous Pax6 mutant mice. Diabetes Metab Res Rev 30: , 467–475. |
[216] | Kiliaan AJ , Arnoldussen IAC , Gustafson DR ((2014) ) Adipokines: A link between obesity and dementia? . Lancet Neurol 13: , 913–923. |
[217] | Leboucher A , Laurent C , Fernandez-Gomez FJ , Burnouf S , Troquier L , Eddarkaoui S , Demeyer D , Caillierez R , Zommer N , Vallez E , Bantubungi K , Breton C , Pigny P , Buee-Scherrer V , Staels B , Hamdane M , Tailleux A , Buee L , Blum D ((2013) ) Detrimental effects of diet-induced obesity on tau pathology are independent of insulin resistance in tau transgenic mice. Diabetes 62: , 1681–1688. |
[218] | Gratuze M , Julien J , Morin F , Calon F , Hebert SS , Marette A , Planel E ((2016) ) High-fat, high-sugar, and high-cholesterol consumption does not impact tau pathogenesis in a mouse model of Alzheimer’s disease-like tau pathology. Neurobiol Aging 47: , 71–73. |
[219] | Hull C , Dekeryte R , Koss DJ , Crouch B , Buchanan H , Delibegovic M , Platt B ((2020) ) Knock-in of mutated hTAU causes insulin resistance, inflammation and proteostasis disturbance in a mouse model of frontotemporal dementia. Mol Neurobiol 57: , 539–550. |
[220] | Marciniak E , Leboucher A , Caron E , Ahmed T , Tailleux A , Dumont J , Issad T , Gerhardt E , Pagesy P , Vileno M , Bournonville C , Hamdane M , Bantubungi K , Lancel S , Demeyer D , Eddarkaoui S , Vallez E , Vieau D , Humez S , Faivre E , Grenier-Boley B , Outeiro TF , Staels B , Amouyel P , Balschun D , Buee L , Blum D ((2017) ) Tau deletion promotes brain insulin resistance. J Exp Med 214: , 2257–2269. |
[221] | Allweyer M , Emde M , Bähr I , Spielmann J , Bieramperl P , Naujoks W , Kielstein H ((2022) ) Investigation of behavior and plasma levels of corticosterone in restrictive- and ad libitum-fed diet-induced obese mice. Nutrients 14: , 1746. |
[222] | Bracko O , Vinarcsik LK , Cruz Hernandez JC , Ruiz-Uribe NE , Haft-Javaherian M , Falkenhain K , Ramanauskaite EM , Ali M , Mohapatra A , Swallow MA , Njiru BN , Muse V , Michelucci PE , Nishimura N , Schaffer CB ((2020) ) High fat diet worsens Alzheimer’s disease-related behavioral abnormalities and neuropathology in APP/PS1 mice, but not by synergistically decreasing cerebral blood flow. Sci Rep 10: , 9884. |
[223] | Sah SK , Lee C , Jang JH , Park GH ((2017) ) Effect of high-fat diet on cognitive impairment in triple-transgenic mice model of Alzheimer’s disease. Biochem Biophys Res Commun 493: , 731–736. |
[224] | Sanguinetti E , Guzzardi MA , Panetta D , Tripodi M , De Sena V , Quaglierini M , Burchielli S , Salvadori PA , Iozzo P ((2019) ) Combined effect of fatty diet and cognitive decline on brain metabolism, food intake, body weight, and counteraction by intranasal insulin therapy in 3xTg mice. Front Cell Neurosci 13: , 188. |
[225] | Mancini G , Dias C , Lourenco CF , Laranjinha J , de Bem A , Ledo A ((2021) ) A high fat/cholesterol diet recapitulates some Alzheimer’s disease-like features in mice: Focus on hippocampal mitochondrial dysfunction. J Alzheimers Dis 82: , 1619–1633. |
[226] | Ma X , Guo Y , Xu J , Wang X , Dong S , Gao Y , Van Halm-Lutterodt N , Yuan L ((2022) ) Effects of distinct n-6 to n-3 polyunsaturated fatty acid ratios on insulin resistant and AD-like phenotypes in high-fat diets-fed APP/PS1 mice. Food Res Int 162: , 112207. |
[227] | Busciglio J , Pelsman A , Wong C , Pigino G , Yuan M , Mori H , Yankner BA ((2002) ) Altered metabolism of the amyloid beta precursor protein is associated with mitochondrial dysfunction in Down’s syndrome. Neuron 33: , 677–688. |
[228] | Okazawa H ((2021) ) Intracellular amyloid hypothesis for ultra-early phase pathology of Alzheimer’s disease. Neuropathology 41: , 93–98. |
[229] | Ricciarelli R , Fedele E ((2017) ) The amyloid cascade hypothesis in Alzheimer’s disease: It’s time to change our mind. Curr Neuropharmacol 15: , 926–935. |
[230] | Selkoe DJ ((2001) ) Alzheimer’s disease: Genes, proteins, and therapy. Physiol Rev 81: , 741–766. |
[231] | Zaretsky DV , Zaretskaia MV ((2021) ) Mini-review: Amyloid degradation toxicity hypothesis of Alzheimer’s disease. Neurosci Lett 756: , 135959. |
[232] | Jellinger KA ((2020) ) Neuropathological assessment of the Alzheimer spectrum. J Neural Transm (Vienna) 127: , 1229–1256. |
[233] | Bourassa P , Tremblay C , Schneider JA , Bennett DA , Calon F ((2019) ) Beta-amyloid pathology in human brain microvessel extracts from the parietal cortex: Relation with cerebral amyloid angiopathy and Alzheimer’s disease. Acta Neuropathol 137: , 801–823. |
[234] | Lucey BP , Mawuenyega KG , Patterson BW , Elbert DL , Ovod V , Kasten T , Morris JC , Bateman RJ ((2017) ) Associations between β-amyloid kinetics and the β-amyloid diurnal pattern in the central nervous system. JAMA Neurol 74: , 207–215. |
[235] | Gupta A , Iadecola C ((2015) ) Impaired Aβ clearance: A potential link between atherosclerosis and Alzheimer’s disease. Front Aging Neurosci 7: , 115. |
[236] | Tarasoff-Conway JM , Carare RO , Osorio RS , Glodzik L , Butler T , Fieremans E , Axel L , Rusinek H , Nicholson C , Zlokovic BV , Frangione B , Blennow K , Ménard J , Zetterberg H , Wisniewski T , de Leon MJ ((2015) ) Clearance systems in the brain-implications for Alzheimerdisease. Nat Rev Neurol 11: , 457–470. |
[237] | De Strooper B , Karran E ((2016) ) The cellular phase of Alzheimer’s disease. Cell 164: , 603–615. |
[238] | Montine TJ , Phelps CH , Beach TG , Bigio EH , Cairns NJ , Dickson DW , Duyckaerts C , Frosch MP , Masliah E , Mirra SS , Nelson PT , Schneider JA , Thal DR , Trojanowski JQ , Vinters HV , Hyman BT ((2012) ) National Institute on Aging-Alzheimer’s Association guidelines for the neuropathologic assessment of Alzheimer’s disease: A practical approach. Acta Neuropathol 123: , 1–11. |
[239] | Pedrini S , Thomas C , Brautigam H , Schmeidler J , Ho L , Fraser P , Westaway D , Hyslop PS , Martins RN , Buxbaum JD , Pasinetti GM , Dickstein DL , Hof PR , Ehrlich ME , Gandy S ((2009) ) Dietary composition modulates brain mass and solubilizable Abeta levels in a mouse model of aggressive Alzheimer’s amyloid pathology. Mol Neurodegener 4: , 40. |
[240] | Li L , Cao D , Garber DW , Kim H , Fukuchi K-i ((2003) ) Association of aortic atherosclerosis with cerebral β-amyloidosis and learning deficits in a mouse model of Alzheimer’s disease. Am J Pathol 163: , 2155–2164. |
[241] | Mazzei G , Ikegami R , Abolhassani N , Haruyama N , Sakumi K , Saito T , Saido TC , Nakabeppu Y ((2021) ) A high-fat diet exacerbates the Alzheimer’s disease pathology in the hippocampus of the App(NL-F/NL-F) knock-in mouse model. Aging Cell 20: , e13429. |
[242] | Ramos-Rodriguez JJ , Ortiz-Barajas O , Gamero-Carrasco C , de la Rosa PR , Infante-Garcia C , Zopeque-Garcia N , Lechuga-Sancho AM , Garcia-Alloza M ((2014) ) Prediabetes-induced vascular alterations exacerbate central pathology in APPswe/PS1dE9 mice. Psychoneuroendocrinology 48: , 123–135. |
[243] | Tremblay C , Francois A , Delay C , Freland L , Vandal M , Bennett DA , Calon F ((2017) ) Association of neuropathological markers in the parietal cortex with antemortem cognitive function in persons with mild cognitive impairment and Alzheimer disease. J Neuropathol Exp Neurol 76: , 70–88. |
[244] | Wesseling H , Mair W , Kumar M , Schlaffner CN , Tang S , Beerepoot P , Fatou B , Guise AJ , Cheng L , Takeda S , Muntel J , Rotunno MS , Dujardin S , Davies P , Kosik KS , Miller BL , Berretta S , Hedreen JC , Grinberg LT , Seeley WW , Hyman BT , Steen H , Steen JA ((2020) ) Tau PTM profiles identify patient heterogeneity and stages of Alzheimer’s disease. Cell 183: , 1699–1713.e13. |
[245] | Gratuze M , Joly-Amado A , Buee L , Vieau D , Blum D ((2019) ) Tau, diabetes and insulin. Adv Exp Med Biol 1184: , 259–287. |
[246] | Tremblay C , Pilote M , Phivilay A , Emond V , Bennett DA , Calon F ((2007) ) Biochemical characterization of Aβ and tau pathologies in mild cognitive impairment and Alzheimer’s disease. J Alzheimers Dis 12: , 377–390. |
[247] | Gao Y , Tan L , Yu JT , Tan L ((2018) ) Tau in Alzheimer’s disease: Mechanisms and therapeutic strategies. Curr Alzheimer Res 15: , 283–300. |
[248] | Petrov D , Pedros I , Artiach G , Sureda FX , Barroso E , Pallas M , Casadesus G , Beas-Zarate C , Carro E , Ferrer I , Vazquez-Carrera M , Folch J , Camins A ((2015) ) High-fat diet-induced deregulation of hippocampal insulin signaling and mitochondrial homeostasis deficiences contribute to Alzheimer disease pathology in rodents. Biochim Biophys Acta 1852: , 1687–1699. |
[249] | Koga S , Kojima A , Ishikawa C , Kuwabara S , Arai K , Yoshiyama Y ((2014) ) Effects of diet-induced obesity and voluntary exercise in a tauopathy mouse model: Implications of persistent hyperleptinemia and enhanced astrocytic leptin receptor expression. Neurobiol Dis 71: , 180–192. |
[250] | Julien C , Tremblay C , Phivilay A , Berthiaume L , Emond V , Julien P , Calon F ((2010) ) High-fat diet aggravates amyloid-beta and tau pathologies in the 3xTg-AD mouse model. Neurobiol Aging 31: , 1516–1531. |
[251] | Elahi M , Motoi Y , Shimonaka S , Ishida Y , Hioki H , Takanashi M , Ishiguro K , Imai Y , Hattori N ((2021) ) High-fat diet-induced activation of SGK1 promotes Alzheimer’s disease-associated tau pathology. Hum Mol Genet 30: , 1693–1710. |
[252] | Gannon OJ , Robison LS , Salinero AE , Abi-Ghanem C , Mansour FM , Kelly RD , Tyagi A , Brawley RR , Ogg JD , Zuloaga KL ((2022) ) High-fat diet exacerbates cognitive decline in mouse models of Alzheimer’s disease and mixed dementia in a sex-dependent manner. J Neuroinflammation 19: , 110. |
[253] | King MR , Anderson NJ , Deciu M , Guernsey LS , Cundiff M , Hajizadeh S , Jolivalt CG ((2020) ) Insulin deficiency, but not resistance, exaggerates cognitive deficits in transgenic mice expressing human amyloid and tau proteins. Reversal by Exendin-4 treatment. J Neurosci Res 98: , 2357–2369. |
[254] | To AW , Ribe EM , Chuang TT , Schroeder JE , Lovestone S ((2011) ) The epsilon3 and epsilon4 alleles of human APOE differentially affect tau phosphorylation in hyperinsulinemic and pioglitazone treated mice. PLoS One 6: , e16991. |
[255] | Colom-Cadena M , Spires-Jones T , Zetterberg H , Blennow K , Caggiano A , DeKosky ST , Fillit H , Harrison JE , Schneider LS , Scheltens P , de Haan W , Grundman M , van Dyck CH , Izzo NJ , Catalano SM ((2020) ) The clinical promise of biomarkers of synapse damage or loss in Alzheimer’s disease. Alzheimers Res Ther 12: , 21. |
[256] | Selkoe DJ ((2002) ) Alzheimer’s disease is a synaptic failure. Science 298: , 789–791. |
[257] | Scheff SW , Neltner JH , Nelson PT ((2014) ) Is synaptic loss a unique hallmark of Alzheimer’s disease? Biochem Pharmacol 88: , 517–528. |
[258] | Calon F , Lim GP , Yang F , Morihara T , Teter B , Ubeda O , Rostaing P , Triller A , Salem N Jr , Ashe KH , Frautschy SA , Cole GM ((2004) ) Docosahexaenoic acid protects from dendritic pathology in an Alzheimer’s disease mouse model. Neuron 43: , 633–645. |
[259] | Terry RD , Masliah E , Salmon DP , Butters N , DeTeresa R , Hill R , Hansen LA , Katzman R ((1991) ) Physical basis of cognitive alterations in Alzheimer’s disease: Synapse loss is the major correlate of cognitive impairment. Ann Neurol 30: , 572–580. |
[260] | Julien C , Tremblay C , Bendjelloul F , Phivilay A , Coulombe MA , Emond V , Calon F ((2008) ) Decreased drebrin mRNA expression in Alzheimer disease: Correlation with tau pathology. J Neurosci Res 86: , 2292–2302. |
[261] | Jia L , Zhu M , Kong C , Pang Y , Zhang H , Qiu Q , Wei C , Tang Y , Wang Q , Li Y , Li T , Li F , Wang Q , Li Y , Wei Y , Jia J ((2021) ) Blood neuro-exosomal synaptic proteins predict Alzheimer’s disease at the asymptomatic stage. Alzheimers Dement 17: , 49–60. |
[262] | Sandelius A , Portelius E , Kallen A , Zetterberg H , Rot U , Olsson B , Toledo JB , Shaw LM , Lee VMY , Irwin DJ , Grossman M , Weintraub D , Chen-Plotkin A , Wolk DA , McCluskey L , Elman L , Kostanjevecki V , Vandijck M , McBride J , Trojanowski JQ , Blennow K ((2019) ) Elevated CSF GAP-43 is Alzheimer’s disease specific and associated with tau and amyloid pathology. Alzheimers Dement 15: , 55–64. |
[263] | Brinkmalm A , Brinkmalm G , Honer WG , Frölich L , Hausner L , Minthon L , Hansson O , Wallin A , Zetterberg H , Blennow K , AnnikaÖhrfelt A ((2014) ) SNAP-25 is a promising novel cerebrospinalfluid biomarker for synapse degeneration in Alzheimer’s disease. Mol Neurodegener 9: , 2–13. |
[264] | Spires-Jones TL , Hyman BT ((2014) ) The intersection of amyloid beta and tau at synapses in Alzheimer’s disease. Neuron 82: , 756–771. |
[265] | Pickett EK , Rose J , McCrory C , McKenzie CA , King D , Smith C , Gillingwater TH , Henstridge CM , Spires-Jones TL ((2018) ) Region-specific depletion of synaptic mitochondria in the brains of patients with Alzheimer’s disease. Acta Neuropathol 136: , 747–757. |
[266] | Koffie RM , Meyer-Luehmann M , Hashimoto T , Adams KW , Mielke ML , Garcia-Alloza M , Micheva KD , Smith SJ , Kim ML , Lee VM , Hyman BT , Spires-Jones TL ((2009) ) Oligomeric amyloid beta associates with postsynaptic densities and correlates with excitatory synapse loss near senile plaques. Proc Natl Acad Sci U S A 106: , 4012–4017. |
[267] | Bhat NR , Thirumangalakudi L ((2013) ) Increased tau phosphorylation and impaired brain insulin/IGF signaling in mice fed a high fat/high cholesterol diet. J Alzheimers Dis 36: , 781–789. |
[268] | Perez SE , Berg BM , Moore KA , He B , Counts SE , Fritz JJ , Hu YS , Lazarov O , Lah JJ , Mufson EJ ((2010) ) DHA diet reduces AD pathology in young APPswe/PS1 Delta E9 transgenic mice: Possible gender effects. J Neurosci Res 88: , 1026–1040. |
[269] | Tönnies E , Trushina E ((2017) ) Oxidative stress, synaptic dysfunction, and Alzheimer’s disease. J Alzheimers Dis 57: , 1105–1121. |
[270] | Swerdlow RH ((2018) ) Mitochondria and mitochondrial cascades in Alzheimer’s disease. J Alzheimers Dis 62: , 1403–1416. |
[271] | Flannery PJ , Trushina E ((2019) ) Mitochondrial dysfunction in Alzheimer’s disease and progress in mitochondria-targeted therapeutics. Curr Behav Neurosci Rep 6: , 88–102. |
[272] | Heneka MT , Carson MJ , Khoury JE , Landreth GE , Brosseron F , Feinstein DL , Jacobs AH , Wyss-Coray T , Vitorica J , Ransohoff RM , Herrup K , Frautschy SA , Finsen B , Brown GC , Verkhratsky A , Yamanaka K , Koistinaho J , Latz E , Halle A , Petzold GC , Town T , Morgan D , Shinohara ML , Perry VH , Holmes C , Bazan NG , Brooks DJ , Hunot S , Joseph B , Deigendesch N , Garaschuk O , Boddeke E , Dinarello CA , Breitner JC , Cole GM , Golenbock DT , Kummer MP ((2015) ) Neuroinflammation in Alzheimer’s disease. Lancet Neurol 14: , 388–405. |
[273] | Bradley-Whitman MA , Lovell MA ((2015) ) Biomarkers of lipid peroxidation in Alzheimer disease (AD): An update. Arch Toxicol 89: , 1035–1044. |
[274] | Chiesa ST , Charakida M , Georgiopoulos G , Roberts JD , Stafford SJ , Park C , Mykkanen J , Kahonen M , Lehtimaki T , Ala-Korpela M , Raitakari O , Pietiainen M , Pussinen P , Muthurangu V , Hughes AD , Sattar N , Timpson NJ , Deanfield JE ((2022) ) Glycoprotein acetyls: A novel inflammatory biomarker of early cardiovascular risk in the young. J Am Heart Assoc 11: , e024380. |
[275] | Myette-Cote E , Durrer C , Neudorf H , Bammert TD , Botezelli JD , Johnson JD , DeSouza CA , Little JP ((2018) ) The effectof a short-term low-carbohydrate, high-fat diet with or without postmeal walks on glycemic control andinflammation in type 2 diabetes: A randomized trial. Am J Physiol Regul Integr Comp Physiol 315: , R1210–R1219. |
[276] | Hu T , Yuan X , Wei G , Luo H , Lee HJ , Jin W ((2018) ) Myricetin-induced brown adipose tissue activation prevents obesity and insulin resistance in db/db mice. Eur J Nutr 57: , 391–403. |
[277] | Shie FS , Jin LW , Cook DG , Leverenz JB , LeBoeuf R ((2002) ) Diet-induced hypercholesterolemia enhances brain Ab accumulation in transgenic mice. Neuroreport 13: , 455–459. |
[278] | Thériault P , ElAli A , Rivest S ((2016) ) High fat diet exacerbatesAlzheimer’s disease-related pathology in APPswe/PS1 mice. Oncotarget 7: , 67809–67825. |
[279] | Ettcheto M , Petrov D , Pedros I , Alva N , Carbonell T , Beas-Zarate C , Pallas M , Auladell C , Folch J , Camins A ((2016) ) Evaluation of neuropathological effects of a high-fat diet in a presymptomatic Alzheimer’s disease stage in APP/PS1 mice. J Alzheimers Dis 54: , 233–251. |
[280] | Statovci D , Aguilera M , MacSharry J , Melgar S ((2017) ) The impact of western diet and nutrients on the microbiota and immune response at mucosal interfaces. Front Immunol 8: , 838. |
[281] | Moreno-Fernández S , Garcés-Rimón M , Vera G , Astier J , Landrier JF , Miguel M ((2018) ) High fat/high glucose diet inducesmetabolic syndrome in an experimental rat model. Nutrients 10: , 1502. |
[282] | Desmoulins L , Chrétien C , Paccoud R , Collins S , Cruciani-Guglielmacci C , Galinier A , Liénard F , Quinault A , Grall S , Allard C , Fenech C , Carneiro L , Mouillot T , Fournel A , Knauf C , Magnan C , Fioramonti X , Pénicaud L , Leloup C ((2019) ) Mitochondrial Dynamin-Related Protein 1 (DRP1) translocation inresponse to cerebral glucose is impaired in a rat model of earlyalteration in hypothalamic glucose sensing. Mol Metab 20: , 166–177. |
[283] | Lin B , Hasegawa Y , Takane K , Koibuchi N , Cao C , Kim-Mitsuyama S ((2016) ) High-fat-diet intake enhances cerebral amyloid angiopathy and cognitive impairment in a mouse model of Alzheimer’s disease, independently of metabolic disorders. J Am Heart Assoc 5: , e003154. |
[284] | Graham LC , Harder JM , Soto I , de Vries WN , John SW , Howell GR ((2016) ) Chronic consumption of a western diet induces robust glial activation in aging mice and in a mouse model of Alzheimer’s disease. Sci Rep 6: , 21568. |
[285] | Christensen A , Pike CJ ((2019) ) APOE genotype affects metabolic and Alzheimer-related outcomes induced by Western diet in female EFAD mice. FASEB J 33: , 4054–4066. |
[286] | Moser VA , Pike CJ ((2017) ) Obesity accelerates Alzheimer-related pathology in APOE4 but not APOE3 Mice. eNeuro 4: , ENEURO.0077-17.2017. |
[287] | Crawford MA , Casperd NM , Sinclair AJ ((1976) ) The long chain metabolites of linoleic avid linolenic acids in liver and brain in herbivores and carnivores. Comp Biochem Physiol B 54: , 395–401. |
[288] | Diau GY , Hsieh AT , Sarkadi-Nagy EA , Wijendran V , Nathanielsz PW , Brenna JT ((2005) ) The influence of long chain polyunsaturate supplementation on docosahexaenoic acid and arachidonic acid in baboon neonate central nervous system. BMC Med 3: , 11. |
[289] | Julien C , Berthiaume L , Hadj-Tahar A , Rajput AH , Bedard PJ , Di Paolo T , Julien P , Calon F ((2006) ) Postmortem brain fatty acid profile of levodopa-treated Parkinson disease patients and parkinsonian monkeys. Neurochem Int 48: , 404–414. |
[290] | Moriguchi T , Loewke J , Garrison M , Catalan JN , Salem N ((2001) ) Reversal of docosahexaenoic acid deficiency in the rat brain, retina, liver, and serum. J Lipid Res 42: , 419–427. |
[291] | Sastry PS ((1985) ) Lipids of nervous tissue: Composition and metabolism. Prog Lipid Res 24: , 69–176. |
[292] | Bourre JM , Pascal G , Durand G , Masson M , Dumont O , Piciotti M ((1984) ) Alterations in the fatty acid composition of rat brain cells (neurons, astrocytes, and oligodendrocytes) and of subcellular fractions (myelin and synaptosomes) induced by a diet devoid of n-3 fatty acids. J Neurochem 43: , 342–348. |
[293] | Greenwood CE , Winocur G ((1996) ) Cognitive impairment in rats fed high-fat diets: A specific effect of saturated fatty-acid intake. Behav Neurosci 110: , 451–459. |
[294] | Bousquet M , St-Amour I , Vandal M , Julien P , Cicchetti F , Calon F ((2012) ) High-fat diet exacerbates MPTP-induced dopaminergic degeneration in mice. Neurobiol Dis 45: , 529–538. |
[295] | Sighinolfi G , Clark S , Blanc L , Cota D , Rhourri-Frih B ((2021) ) Mass spectrometry imaging of mice brain lipid profile changes over time under high fat diet. Sci Rep 11: , 19664. |
[296] | Butler MJ , Cole RM , Deems NP , Belury MA , Barrientos RM ((2020) ) Fatty food, fatty acids, and microglial priming in the adult and aged hippocampus and amygdala. Brain Behav Immun 89: , 145–158. |
[297] | Demers G , Roy J , Machuca-Parra AI , Dashtehei Pour Z , Bairamian D , Daneault C , Rosiers CD , Ferreira G , Alquier T , Fulton S , Representative of c ((2020) ) Fish oil supplementation alleviates metabolic and anxiodepressive effects of diet-induced obesity and associated changes in brain lipid composition in mice. Int J Obes (Lond) 44: , 1936–1945. |
[298] | Bazan NG , Musto AE , Knott EJ ((2011) ) Endogenous signaling by omega-3 docosahexaenoic acid-derived mediators sustains homeostatic synaptic and circuitry integrity. Mol Neurobiol 44: , 216–222. |
[299] | Vouimba RM , Bakoyiannis I , Ducourneau EG , Maroun M , Ferreira G ((2021) ) Bidirectional modulation of hippocampal and amygdala synaptic plasticity by post-weaning obesogenic diet intake in male rats: Influence of the duration of diet exposure. Hippocampus 31: , 117–121. |
[300] | Arsenault D , Julien C , Chen CT , Bazinet RP , Calon F ((2012) ) Dietary intake of unsaturated fatty acids modulates physiological properties of entorhinal cortex neurons in mice. J Neurochem 122: , 427–443. |
[301] | Arsenault D , Julien C , Tremblay C , Calon F ((2011) ) DHA improves cognition and prevents dysfunction of entorhinal cortex neurons in 3xTg-AD mice. PLoS One 6: , e17397. |
[302] | Crawford MA , Schmidt WF , Broadhurst CL , Wang Y ((2021) ) Lipids in the origin of intracellular detail and speciationin the Cambrian epoch and the significance of the last double bond of docosahexaenoic acid in cell signaling. Prostaglandins Leukot Essent Fatty Acids 166: , 102230. |
[303] | Bruno MJ , Koeppe RE , 2nd, Andersen OS ((2007) ) Docosahexaenoic acid alters bilayer elastic properties. Proc Natl Acad Sci U S A 104: , 9638–9643. |
[304] | Jais A , Solas M , Backes H , Chaurasia B , Kleinridders A , Theurich S , Mauer J , Steculorum SM , Hampel B , Goldau J , Alber J , Forster CY , Eming SA , Schwaninger M , Ferrara N , Karsenty G , Bruning JC ((2016) ) Myeloid-cell-derived VEGF maintains brain glucose uptake and limits cognitive impairment in obesity. Cell 165: , 882–895. |
[305] | Schuler R , Seebeck N , Osterhoff MA , Witte V , Floel A , Busjahn A , Jais A , Bruning JC , Frahnow T , Kabisch S , Pivovarova O , Hornemann S , Kruse M , Pfeiffer AFH ((2018) ) VEGF and GLUT1 are highly heritable, inversely correlated and affected by dietary fat intake: Consequences for cognitive function in humans. Mol Metab 11: , 129–136. |
[306] | Kothari V , Luo Y , Tornabene T , O’Neill AM , Greene MW , Geetha T , Babu JR ((2017) ) High fat diet induces brain insulin resistance and cognitive impairment in mice. Biochim Biophys Acta Mol Basis Dis 1863: , 499–508. |
[307] | Li W , Prakash R , Chawla D , Du W , Didion SP , Filosa JA , Zhang Q , Brann DW , Lima VV , Tostes RC , Ergul A ((2013) ) Early effects of high-fat diet on neurovascular function and focal ischemic brain injury. Am J Physiol Regul Integr Comp Physiol 304: , R1001–1008. |
[308] | Amine H , Benomar Y , Taouis M ((2021) ) Palmitic acid promotes resistin-induced insulin resistance and inflammation in SH-SY5Y human neuroblastoma. Sci Rep 11: , 5427. |
[309] | Fatima S , Hu X , Gong RH , Huang C , Chen M , Wong HLX , Bian Z , Kwan HY ((2019) ) Palmitic acid is an intracellular signaling molecule involved in disease development. Cell Mol Life Sci 76: , 2547–2557. |
[310] | Cole GM , Frautschy SA ((2006) ) Docosahexaenoic acid protects from amyloid and dendritic pathology in an Alzheimer’s disease mouse model. Nutr Health 2018: , 249–259. |
[311] | Tournissac M , Vandal M , Tremblay C , Bourassa P , Vancassel S , Emond V , Gangloff A , Calon F ((2018) ) Dietary intakeof branched-chain amino acids in a mouse model of Alzheimer’s disease: Effects on survival, behavior, andneuropathology. Alzheimers Dement (N Y) 4: , 677–687. |
[312] | Vinuesa A , Bentivegna M , Calfa G , Filipello F , Pomilio C , Bonaventura MM , Lux-Lantos V , Matzkin ME , Gregosa A , Presa J , Matteoli M , Beauquis J , Saravia F ((2019) ) Early exposure to a high-fat diet impacts on hippocampal plasticity: Implication of microglia-derived exosome-like extracellular vesicles. Mol Neurobiol 56: , 5075–5094. |
[313] | Crescenzo R , Spagnuolo MS , Cancelliere R , Iannotta L , Mazzoli A , Gatto C , Iossa S , Cigliano L ((2019) ) Effect of initial aging and high-fat/high-fructose diet on mitochondrial bioenergetics and oxidative status in rat brain. Mol Neurobiol 56: , 7651–7663. |
[314] | Chen K , Yuan R , Geng S , Zhang Y , Ran T , Kowalski E , Liu J , Li L ((2017) ) Toll-interacting protein deficiency promotes neurodegeneration via impeding autophagy completion in high-fat diet-fed ApoE(-/-) mouse model. Brain Behav Immun 59: , 200–210. |
[315] | Pantiya P , Thonusin C , Chunchai T , Ongnok B , Nawara W , Arunsak B , Chattipakorn N , Chattipakorn SC ((2023) ) Higher untrained fitness exerts a neuroprotection in Independence to caloric restriction or exercise in high-fat diet-induced obesity. Exp Neurol 365: , 114416. |
[316] | Gali CC , Fanaee-Danesh E , Zandl-Lang M , Albrecher NM , Tam-Amersdorfer C , Stracke A , Sachdev V , Reichmann F , Sun Y , Avdili A , Reiter M , Kratky D , Holzer P , Lass A , Kandimalla KK , Panzenboeck U ((2019) ) Amyloid-beta impairs insulin signaling by accelerating autophagy-lysosomal degradation of LRP-1 and IR-β in blood-brain barrier endothelial cells and in 3XTg-AD mice. Mol Cell Neurosci 99: , 103390. |
[317] | Davis JF , Tracy AL , Schurdak JD , Tschop MH , Lipton JW , Clegg DJ , Benoit SC ((2008) ) Exposure to elevated levels of dietary fat attenuates psychostimulant reward and mesolimbic dopamine turnover in the rat. Behav Neurosci 122: , 1257–1263. |
[318] | Martin H , Bullich S , Martinat M , Chataigner M , Di Miceli M , Simon V , Clark S , Butler J , Schell M , Chopra S , Chaouloff F , Kleinridders A , Cota D , De Deurwaerdere P , Penicaud L , Laye S , Guiard BP , Fioramonti X (2022) Insulin modulates emotional behavior through a serotonin-dependent mechanism. Mol Psychiatry, doi: 10.1038/s41380-022-01812-3. |
[319] | Fulton S , Decarie-Spain L , Fioramonti X , Guiard B , Nakajima S ((2022) ) The menace of obesity to depression and anxiety prevalence. Trends Endocrinol Metab 33: , 18–35. |
[320] | Gao Y , Bielohuby M , Fleming T , Grabner GF , Foppen E , Bernhard W , Guzman-Ruiz M , Layritz C , Legutko B , Zinser E , Garcia-Caceres C , Buijs RM , Woods SC , Kalsbeek A , Seeley RJ , Nawroth PP , Bidlingmaier M , Tschop MH , Yi CX ((2017) ) Dietary sugars, not lipids, drive hypothalamic inflammation. Mol Metab 6: , 897–908. |
[321] | Milanski M , Degasperi G , Coope A , Morari J , Denis R , Cintra DE , Tsukumo DM , Anhe G , Amaral ME , Takahashi HK , Curi R , Oliveira HC , Carvalheira JB , Bordin S , Saad MJ , Velloso LA ((2009) ) Saturated fatty acids produce an inflammatory response predominantly through the activation of TLR4 signaling in hypothalamus: Implications for the pathogenesis of obesity. J Neurosci 29: , 359–370. |
[322] | Maric T , Woodside B , Luheshi GN ((2014) ) The effects of dietary saturated fat on basal hypothalamic neuroinflammation in rats. Brain Behav Immun 36: , 35–45. |
[323] | Sobesky JL , Barrientos RM , De May HS , Thompson BM , Weber MD , Watkins LR , Maier SF ((2014) ) High-fat diet consumption disrupts memory and primes elevations in hippocampal IL-1beta, an effect that can be prevented with dietary reversal or IL-1 receptor antagonism. Brain Behav Immun 42: , 22–32. |
[324] | Spencer SJ , Korosi A , Laye S , Shukitt-Hale B , Barrientos RM ((2017) ) Food for thought: How nutrition impacts cognition and emotion. NPJ Sci Food 1: , 7. |
[325] | McGuire MJ , Ishii M ((2016) ) Leptin dysfunction and Alzheimer’s disease: Evidence from cellular, animal, and human studies. Cell Mol Neurobiol 36: , 203–217. |
[326] | McGregor G , Harvey J ((2018) ) Regulation of hippocampal synaptic function by the metabolic hormone, leptin: Implications for health and neurodegenerative disease. Front Cell Neurosci 12: , 340. |
[327] | Samant NP , Gupta GL ((2021) ) Adiponectin: A potential target for obesity-associated Alzheimer’s disease. Metab Brain Dis 36: , 1565–1572. |
[328] | Forsythe P , Bienenstock J , Kunze WA ((2014) ) Vagal pathways for microbiome-brain-gut axis communication. Adv Exp Med Biol 817: , 115–133. |
[329] | Dinan TG , Cryan JF ((2017) ) Gut instincts: Microbiota as a key regulator of brain development, ageing and neurodegeneration. J Physiol 595: , 489–503. |
[330] | Kim JS , de La Serre CB ((2018) ) Diet, gut microbiota composition and feeding behavior. Physiol Behav 192: , 177–181. |
[331] | Cawthon CR , Kirkland RA , Pandya S , Brinson NA , de La Serre CB ((2020) ) Non-neuronal crosstalk promotes an inflammatory response in nodose ganglia cultures after exposure to byproducts from gram positive, high-fat-diet-associated gut bacteria. Physiol Behav 226: , 113124. |
[332] | Kim JS , Kirkland RA , Lee SH , Cawthon CR , Rzepka KW , Minaya DM , de Lartigue G , Czaja K , de La Serre CB ((2020) ) Gut microbiota composition modulates inflammation and structure of the vagal afferent pathway. Physiol Behav 225: , 113082. |
[333] | Cawthon CR , de La Serre CB ((2021) ) The critical role of CCK in the regulation of food intake and diet-induced obesity. Peptides 138: , 170492. |
[334] | Cryan JF , O’Riordan KJ , Cowan CSM , Sandhu KV , Bastiaanssen TFS , Boehme M , Codagnone MG , Cussotto S , Fulling C , Golubeva AV , Guzzetta KE , Jaggar M , Long-Smith CM , Lyte JM , Martin JA , Molinero-Perez A , Moloney G , Morelli E , Morillas E , O’Connor R , Cruz-Pereira JS , Peterson VL , Rea K , Ritz NL , Sherwin E , Spichak S , Teichman EM , van de Wouw M , Ventura-Silva AP , Wallace-Fitzsimons SE , Hyland N , Clarke G , Dinan TG ((2019) ) The microbiota-gut-brain axis. Physiol Rev 99: , 1877–2013. |
[335] | Liu T , Guo Z , Song X , Liu L , Dong W , Wang S , Xu M , Yang C , Wang B , Cao H ((2020) ) High-fat diet-induced dysbiosis mediates MCP-1/CCR2 axis-dependent M2 macrophage polarization and promotes intestinal adenoma-adenocarcinoma sequence. J Cell Mol Med 24: , 2648–2662. |
[336] | Wu GD , Chen J , Hoffmann C , Bittinger K , Chen YY , Keilbaugh SA , Bewtra M , Knights D , Walters WA , Knight R , Sinha R , Gilroy E , Gupta K , Baldassano R , Nessel L , Li H , Bushman FD , Lewis JD ((2011) ) Linking long-term dietary patterns with gut microbial enterotypes. Science 334: , 105–108. |
[337] | Brandscheid C , Schuck F , Reinhardt S , Schafer KH , Pietrzik CU , Grimm M , Hartmann T , Schwiertz A , Endres K ((2017) ) Altered gut microbiome composition and tryptic activity of the 5xFAD Alzheimer’s mouse model. J Alzheimers Dis 56: , 775–788. |
[338] | Harach T , Marungruang N , Duthilleul N , Cheatham V , Mc Coy KD , Frisoni G , Neher JJ , Fak F , Jucker M , Lasser T , Bolmont T ((2017) ) Reduction of Abeta amyloid pathology in APPPS1 transgenic mice in the absence of gut microbiota. Sci Rep 7: , 41802. |
[339] | Bauerl C , Collado MC , Diaz Cuevas A , Vina J , Perez Martinez G ((2018) ) Shifts in gut microbiota composition in anAPP/PSS1 transgenic mouse model of Alzheimer’s disease during lifespan. Lett Appl Microbiol 66: , 464–471. |
[340] | Shen L , Liu L , Ji HF ((2017) ) Alzheimer’s disease histological and behavioral manifestations in transgenic mice correlate with specific gut microbiome state. J Alzheimers Dis 56: , 385–390. |
[341] | Wu SC , Cao ZS , Chang KM , Juang JL ((2017) ) Intestinal microbial dysbiosis aggravates the progression of Alzheimer’s disease in Drosophila. Nat Commun 8: , 24. |
[342] | Kong C , Gao R , Yan X , Huang L , Qin H ((2019) ) Probiotics improve gut microbiota dysbiosis in obese mice fed a high-fat or high-sucrose diet. Nutrition 60: , 175–184. |
[343] | Craft S , Raman R , Chow TW , Rafii MS , Sun CK , Rissman RA , Donohue MC , Brewer JB , Jenkins C , Harless K , Gessert D , Aisen PS ((2020) ) Safety, efficacy, and feasibility of intranasal insulin for the treatment of mild cognitiveimpairment and Alzheimer disease dementia: A randomized clinical trial. JAMA Neurol 77: , 1099–1109. |
[344] | Kellar D , Lockhart SN , Aisen P , Raman R , Rissman RA , Brewer J , Craft S ((2021) ) Intranasal insulin reduces white matter hyperintensity progression in association with improvements in cognition and CSF biomarker profiles in mild cognitive impairment and Alzheimer’s disease. J Prev Alzheimers Dis 8: , 240–248. |
[345] | Broom GM , Shaw IC , Rucklidge JJ ((2019) ) The ketogenic diet as a potential treatment and prevention strategy for Alzheimer’s disease. Nutrition 60: , 118–121. |
[346] | Sartorius T , Ketterer C , Kullmann S , Balzer M , Rotermund C , Binder S , Hallschmid M , Machann J , Schick F , Somoza V , Preissl H , Fritsche A , Haring HU , Hennige AM ((2012) ) Monounsaturated fatty acids prevent the aversive effects of obesityon locomotion, brain activity, and sleep behavior. Diabetes 61: , 1669–1679. |
[347] | Li JW , Zong Y , Cao XP , Tan L , Tan L ((2018) ) Microglial priming inAlzheimer’s disease. Ann Transl Med 6: , 176. |