Emerging Roles of Meningeal Lymphatic Vessels in Alzheimer’s Disease
Abstract
Meningeal lymphatic vessels (mLVs), the functional lymphatic system present in the meninges, are the key drainage route responsible for the clearance of molecules, immune cells, and cellular debris from the cerebrospinal fluid and interstitial fluid into deep cervical lymph nodes. Aging and ApoE4, the two most important risk factors for Alzheimer’s disease (AD), induce mLV dysfunction, decrease cerebrospinal fluid influx and outflux, and exacerbate amyloid pathology and cognitive dysfunction. Dysfunction of mLVs results in the deposition of metabolic products, accelerates neuroinflammation, and promotes the release of pro-inflammatory cytokines in the brain. Thus, mLVs represent a novel therapeutic target for treating neurodegenerative and neuroinflammatory diseases. This review aims to summarize the structure and function of mLVs and to discuss the potential effect of aging and ApoE4 on mLV dysfunction, as well as their roles in the pathogenesis of AD.
INTRODUCTION
Alzheimer’s disease (AD) is one of the most common neurodegenerative diseases and has been recognized as a global public health threat [1]. The characteristic neuropathology of AD includes extracellular senile plaques formed by amyloid-β (Aβ) and intracellular neurofibrillary tangles formed by hyperphosphorylated tau [2]. Aβ is cleared through several pathways, including hydrolysis by local proteases [3], uptake and degradation by microglia [4], crossing the blood-brain barrier (BBB) [5], and clearance along perivascular spaces surrounding cerebral arteries [4, 6]. Almost half the amount of Aβ in the brain flows into the peripheral system for further clearance [7, 8].
Meningeal lymphatic vessels (mLVs), a pathway that leads cerebrospinal fluid (CSF) and interstitial fluid (ISF) into deep cervical lymph nodes (dCLNs), have recently been shown to be critical for the clearance of Aβ and tau in the brain [9, 10]. In 2015, two teams independently revealed functional lymphatic vessels lining the dural sinuses in rodents and humans [11, 12]. The vessels express the molecular hallmarks of lymphatic endothelial cells, representing a set of functional lymphatic systems in the brain meninges [11, 12]. Another study using the magnetic resonance imaging (MRI) technique confirmed the presence of mLVs in human and non-human primates [13]. Meanwhile, visualization techniques of the human lymphatic system and CSF flow help to monitor CSF clearance and morphological changes of mLVs in aging and neurodegenerative diseases [14, 15], which gives us a much clearer viewpoint of efficient clearance of protein waste products.
mLVs, embedded within the dura and tracking along the superior sagittal sinus (SSS) and transverse sinus (TS) [16, 17], are critical to the absorption of molecules in CSF and ISF [10, 12, 18]. Aβ may be carried to the peripheral circulation along the CSF clearance pathway, crossing the sieve plate and blood-CSF barrier [19, 20], and through mLVs [11, 12, 21]. mLV dysfunction decreases cerebral perfusion, impairs lymphatic drainage, and ultimately exacerbates amyloid pathology, particularly in the meninges [9]. Ablating dural lymphatics with either genetic manipulation or surgery resulted in significantly slower clearance of injected molecules in the deep parenchyma [12, 22]. Both mLV integrity and CSF drainage are impaired during aging [23]. This review aims to explore the structure and function of mLVs and summarizes the potential effect of aging and ApoE4 on mLV dysfunction, which would interfere with inflammation and Aβ and tau clearance in AD.
STRUCTURE AND FUNCTION OF mLVs
Due to the complex anatomy of the skull base, it is difficult to explore mLVs function in the brain. The skull was reported to play a role in CSF outflow and immunosurveillance. The skull base is rich in the lymphatic network, which is sparse in the upper (dorsal) part of the skull [12]. Most studies focus on dorsal mLVs [9–12, 24, 25]. In fact, other parts, such as the lateral or basal mLVs of the dura mater, have remained largely unexplored. This is partially attributed to the extremely complicated skull base in this area that contains enormous bony structures and subarachnoid cisterns, as well as foramina that contain blood vessels and cranial nerves [26]. Dorsal mLVs are located within the dural folds and travel along the SSS and TS (Fig. 1). The lumen is primarily a discontinuous vascular structure with a small diameter. Most mLVs do not form branches but cluster within the dural folds that encase the SSS and TS. There are no valves in the lumen. Lymphatic endothelial cells (LECs) are mostly immature and connect in a continuous and closed zipper-like manner [27, 28].
Fig. 1
The structure and location of dorsal and basal mLVs. Schematic images of dorsal and basal mLVs tracking along the dural sinus with differences in structures, branches, lumen diameter, and lymphatic valves. Dorsal mLVs travel along the superior sagittal sinus (SSS) and transverse sinus (TS). The lumen is primarily a discontinuous vascular structure, with a small diameter and no valves. Basal mLVs are located near the subarachnoid space (SAS) and run along the petrosal sinus (PSS) and sigmoid sinus (SS). The lumen is primarily a continuous vascular structure with a large diameter and lymphatic valves.
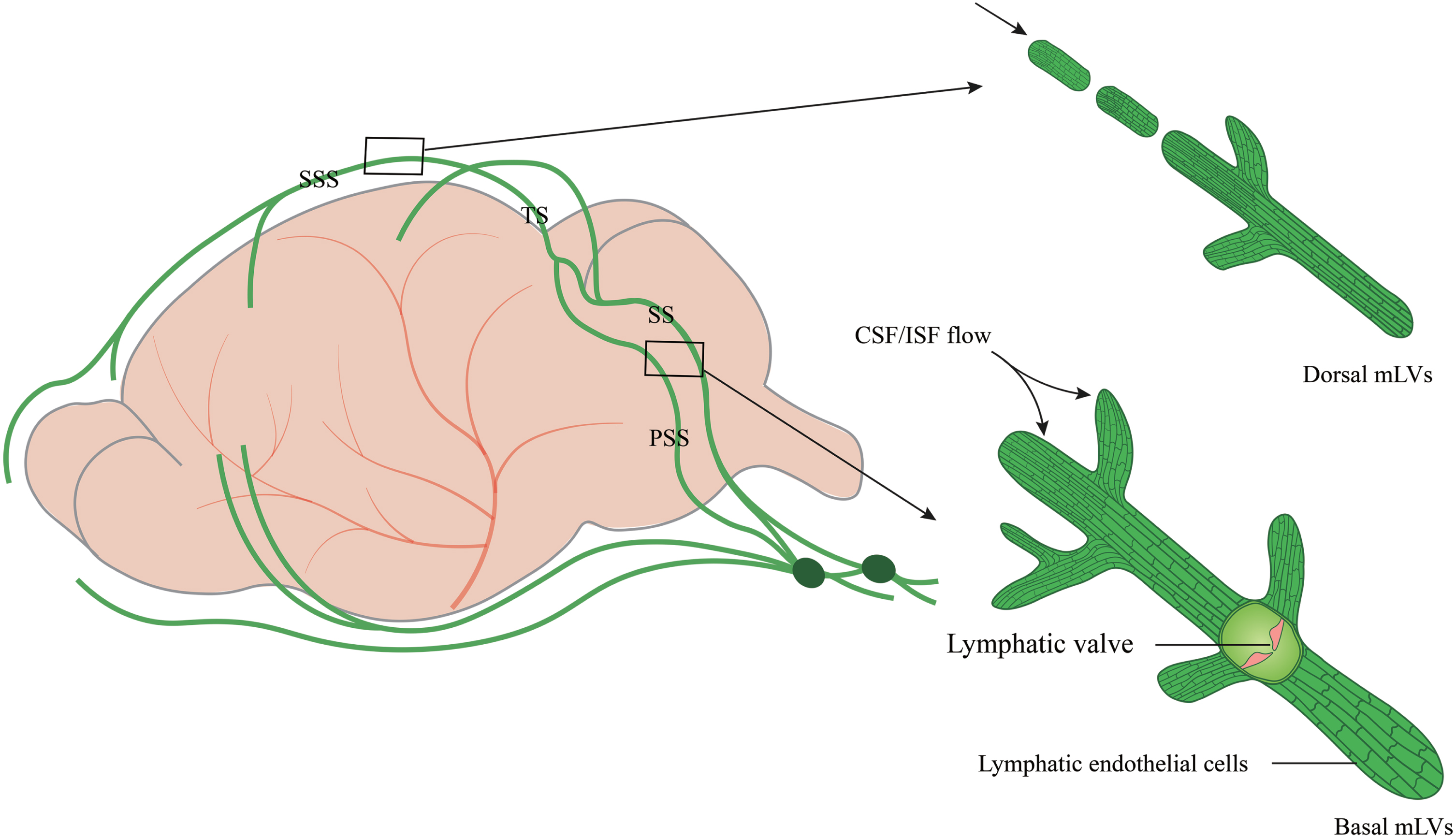
Recent studies have shown that circulating T cells enter the lumen of basal mLVs through the blunt end [27, 28]. Basal mLVs are located near the subarachnoid space (SAS) and run along the petrosal sinus (PSS) and sigmoid sinus (SS) (Fig. 1). They have a large diameter and numerous prominent capillary branches that are rounded and consist of typical oak leaf-like LECs and lymphatic valves. LECs, connected in a button-like manner, have a flap structure. Basal mLVs have lymphatic flaps and capillaries located near the SAS [29]. These structural features and the anatomic location of basal mLVs adjacent to the subarachnoid space make them more likely suitable for fluid uptake and drainage than dorsal mLVs [29]. The basal mLVs absorb and efflux many CSF tracers. Studies in mice also show that basal mLVs are hotspots for the clearance of CSF molecules [29]. The valves of basal mLVs allow the fluid to flow in one direction [30]. Functional assessment of mLVs using CSF contrast-enhanced MRI and fluorescent imaging further revealed that basal mLVs are important routes for macromolecular uptake and CSF drainage [29]. These results indicate a crucial role of basal mLVs in CSF lymphatic drainage. Similarly, dorsal mLVs photoablation significantly decreases drainage of CSF tracers into the dCLNs without structural defects of the basal regions, emphasizing a critical role of initial lymphatics at dorsal mLVs in CSF drainage [31]. Dorsal mLVs are more sensitive to perturbations in VEGFR3 signaling and show earlier regression of lymphatic vessels during aging than basal mLVs [31], while dynamic contrast-enhanced MRI shows that CSF is preferentially discharged through the basal outflow tract [29].
In addition to the drainage of CSF into the dural lymphatic network, a large proportion of CSF drains through the meningeal lymphatic vasculature that runs along the craniofacial and spinal nerves [32–35]. A continuum of metameric spinal lymphatics was described in the vertebral column, both on the dorsal and ventral sides, with lateral exits of the vertebral canal along blood vessels and spinal nerves [25]. In the spinal cord, vertebral lymphatic vessels were found between individual vertebrae where they connect to peripheral sensory and sympathetic ganglia and form metameric vertebral circuits connecting to lymph nodes and the thoracic duct [35]. In addition, CSF also drains through the nasal lymphatic system, in which the septal olfactory nerve lymphatic pathway plays a critical role [36, 37]. There are also clinical data suggesting that children with hydrocephalus develop nasal congestion associated with shunt obstruction [38], suggesting that the drainage of CSF into the lymph nodes is delayed or blocked due to the impairment of the septal nasal lymphatic pathway.
mLVs AND THE GLYMPHATIC SYSTEM
The connection between the mLVs and the glymphatic system was demonstrated by fluorescent tracers and dyes [11]. mLVs absorb fluid from the glymphatic system and then deliver it to extracranial dCLNs [12, 22]. The essence of the glymphatic system is CSF-ISF exchange in the brain parenchyma (Fig. 2). mLVs absorb CSF from the adjacent subarachnoid space and brain ISF through the glymphatic system. CSF in the subarachnoid space flows into deeper brain regions along the arterioles and capillaries and diffuses through the glial limitans into the parenchyma. This is called the periarterial inflow. Efflux of ISF occurs through paravenous spaces back into the subarachnoid CSF. Through glymphatic CSF-ISF exchange in the brain parenchyma, mLVs ultimately transport molecules and waste products into the dCLNs [24]. This system contributes to the clearance of hydrophilic and lipophilic compounds as well as molecules from the brain parenchyma into the CSF and flows with ISF into the perivenous space [39], and then be cleared via drainage through the nasal mucosa lymphatics into cervical lymph nodes [40]. Using a pharmacological method to specifically ablate meningeal (or nasal) lymphatic vessels, the author demonstrates that the nasal route drains directly into the supraclavicular lymph nodes (sCLNs), while the meningeal lymphatic route drains into both the dCLNs and sCLNs [41]. The glymphatic system involves CSF entrance into the periarterial space driven by arteriole pulsatility and CSF pressure and partially through the water channel aquaporin-4 (AQP4) [42, 43]. Intracranial CSF flow is linked to parenchymal ISF circulation [44, 45]. CSF leaves the intracranial circulation by draining into the dural venous sinuses through arachnoid granulations, which contain valves that prevent the backflow of blood or CSF into the CSF compartment. Some authors argue that ISF flows in and out through the periarterial pathway, and there is no evidence of efflux from the parenchyma along with the perivenous pathway [46]. They hypothesized that ISF influx and efflux through the perivascular pathway could go directly to the lymphatics without first mixing with CSF, implying that CSF circulation in the extravascular pathway is not necessary [46]. However, both CSF/ISF and metabolic waste can ultimately enter the dCLNs and the peripheral lymphatic system via mLVs. Impaired glymphatic function leads to the accumulation of pathological products that accelerate the progression of many neurological diseases [47–52].
mLV DYSFUNCTION PROMOTES Aβ ACCUMULATION
Aβ can be cleared from the brain parenchyma through many pathways, such as autophagy and ubiquitination [50]. mLVs are considered an essential mechanism for the clearance of parenchymal Aβ. Contrast medium (a substance used to allow enhanced visualization of tissues) is detected in dCLNs within minutes of CSF delivery, indicating CSF is efficiently drained out of the CNS through the meningeal lymphatics [35, 49, 53]. CSF in the subarachnoid space keeps the CNS active and serves as a fluid source for lymphatic influx [52]. MRI imaging of intrathecally injected contrast in the human brain shows that CSF flows along a pathway similar to the glymphatic system in rodents [54]. PET studies show that reduced CSF clearance in AD patients is associated with increased Aβ deposition [51]. Repetitive transcranial magnetic stimulation has been shown to increase the drainage efficiency of mLVs and improve cognitive memory in 5×FAD mice [55]. In addition, a novel method using the noninvasive music-induced opening of the BBB stimulates lymphatic Aβ clearance [56]. Another study indicated that sound/music facilitates the opening of the BBB, accelerates waste clearance from mLVs, and subsequently improves cognitive function [57]. When the BBB opens with high permeability, the meningeal vascular diameter increases, triggering solute flow from mLVs to the lymph nodes. Thus, the BBB and mLVs cooperate with each other to facilitate the removal of metabolic waste. However, the underlying mechanisms bridging the BBB and meningeal lymphatic drainage remain to be elucidated [57].
Fig. 2
Overview of the transport of CSF and ISF through meningeal lymphatic vessels. Cerebrospinal fluid (CSF) is transported within a specialized perivascular network that helps to remove metabolic products from the central nervous system to the peripheral circulation system. A) CSF transport in the glymphatic pathway through periarterial inflow and perivenous outflow. CSF in the subarachnoid space flows into the brain through the perivascular space of the perforating arteries, which is called the periarterial inflow. Through glymphatic CSF/interstitial fluid (ISF) exchange in the brain parenchyma, metabolic wastes such as Aβ and cytokines are excreted toward the perivenous space. These products ultimately reach deep cervical lymph nodes (dCLNs) and peripheral circulation through meningeal lymphatic vessels. B) Magnified schematic illustration of CSF inflow and ISF outflow to the subarachnoid space along the periarterial and perivenous space.
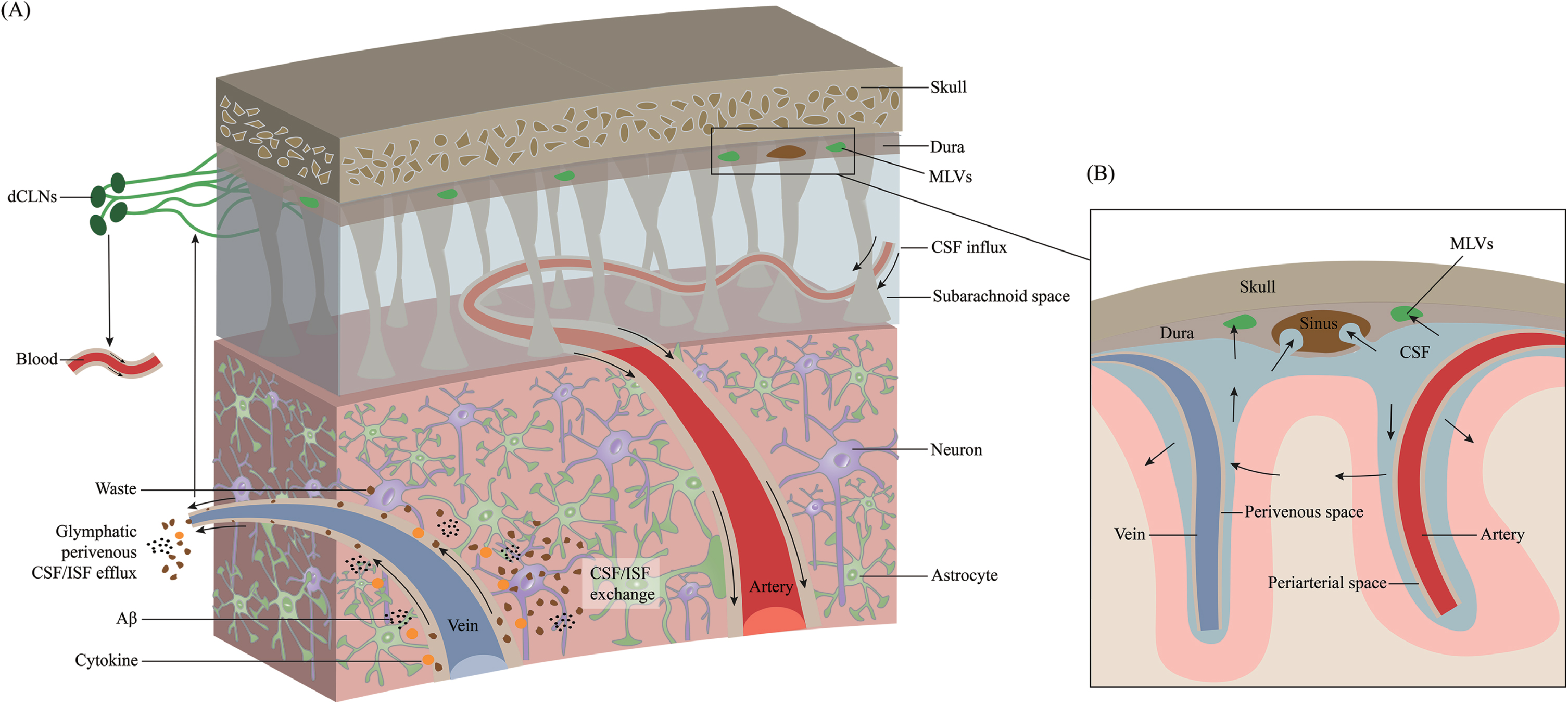
A key driver of ISF/CSF exchange in the mouse glymphatic pathway is arterial pulsation. Ligation of the unilateral internal carotid artery decreases cerebral arterial pulsation and reduces ISF/CSF transport [43]. Blocking the drainage of mLVs exacerbates AD-related pathology in APP/PS1 mice, including Aβ accumulation, AQP4 polarization, and cognitive deficits [58]. Some environmentally modifiable AD risk factors also influence AD pathology by affecting meningeal lymphatics [47, 59, 60]. In a mouse model of traumatic brain injury (TBI), brain trauma causes severe deficits in meningeal lymphatic drainage, which leads to increased neuroinflammation and negative cognitive outcomes [47]. Drainage of CSF from the cisterna magna to the lymph nodes exhibits daily variation. Loss of AQP4 eliminates the day-night difference in the drainage of CSF to the lymph nodes [59]. Furthermore, sleep deprivation impairs glymphatic function, leading to increased Aβ levels in CSF [60, 61]. Instead, the efficiency of Aβ clearance through the glymphatic pathway increases during sleep [15, 62]. Moreover, “Sleep-wakefulness” disorders are associated with increased oxidative stress in the brain and impaired BBB functioning, which presumably enhances Aβ deposition [63]. Meningeal lymphatic function and CSF transport become significantly less efficient in aged mice and in the elderly [64, 65]. Augmentation of meningeal lymphatic drainage in aged mice can ultimately facilitate the clearance of CSF and ISF molecules from the brain, resulting in improved cognitive function. In AD patients, impairment of the lymphatic system is partly due to dysregulation of AQP4 [66, 67]. AQP4 is indispensable for the lymphatic system and is mainly localized at perivascular astrocyte terminals and astrocyte membranes facing the pia mater, maintaining water homeostasis in the CNS [68]. AQP4 acts as an indispensable component of the glymphatic system, facilitates paravascular CSF-ISF exchange, and drives the clearance of substances from the brain parenchyma [44]. AQP4 facilitates CSF inflow into the neuropil. Both CSF influx and ISF efflux are reduced in mice lacking glial AQP4 compared with wild-type littermates [44]. AQP4 expression is upregulated surrounding Aβ plaques [69]. Animal experiments have identified that deletion and loss of AQP4 localization decrease Aβ clearance in APP/PS1 mice, leading to Aβ accumulation in the brain [51, 70, 71]. Furthermore, Aβ moves quickly along the vasculature toward capillaries and large draining veins, and 125I-Aβ clearance is reduced by approximately 55% in AQP4-null mice [44]. In addition, AQP4-deficient APP/PS1 transgenic mice tend to alleviate neuroinflammation but aggravate Aβ accumulation in the brain [72]. However, some authors question the importance of AQP4 in glymphatic transport by showing that AQP4 deletion does not affect CSF tracer distribution, nor does it affect the dispersion of tracers injected into the striatum [73].
AGING AND mLV DYSFUNCTION
Aging is an essential factor affecting the brain’s lymphatic drainage system. Water and metabolites are eliminated rapidly and efficiently by multiple lymphatic drainage pathways, the ability of which declines with increasing age [74, 75]. With aging, the number of lymphatic flaps is reduced, resulting in delayed CSF clearance [30]. Moreover, CSF-ISF exchange is reduced during aging [64]. This is consistent with the observation that the polarization of AQP4 in the perivascular spaces is impaired in old animals [64], leading to failure of waste clearance and accumulation of Aβ in the brain. During aging, the cerebral arteries become stiffer, and the amplitude of pulsations decreases, leading to the retention of fluid in the dilated perivascular space [64]. Compared with their young counterparts, aged mice are less efficient in the perivascular drainage of solutes [76]. Moreover, the thickness of the capillary basement membrane increases with aging [77]. This further affects the transportation of soluble molecules along the cerebrovascular basement membrane, leading to Aβ accumulation in the basement membrane of the cerebrovasculature [78]. Thus, improving the function of the lymphatic drainage system should be beneficial for AD treatment [79].
POTENTIAL EFFECTS OF APOE4 ON mLV DYSFUNCTION
Apolipoprotein E (ApoE) is the most abundant apolipoprotein in the brain and is responsible for lipid transport [80, 81], especially for the redistribution of cholesterol from glial cells to neurons [82]. The ApoE family has been shown to participate in transvascular Aβ clearance through a low-density receptor-related protein-1 (LRP1) transporter. In contrast to ApoE2 and ApoE3, ApoE4 competitively inhibits the binding of Aβ to LRP1 [83]. The ApoE-Aβ interaction regulates Aβ aggregation and clearance in the brain [84]. ApoE4 also reduces the efficiency of Aβ efflux across the BBB, resulting in poor Aβ clearance from the CNS [85]. Class B scavenger receptor type 1 (SRB1), which is expressed in lymphatic endothelial cells and binds to fibrillar Aβ [86, 87], may play a part in mediating Aβ clearance.
mLVs are less complex than peripheral lymphatic vessels, with smaller lymphatic branches and fewer valves to prevent lymphatic reflux [12]. The function of ApoE4 in peripheral lymphatic vessels has been established [88, 89]. Interestingly, ApoE4 may also influence mLVs in the CNS. Analysis of the cell type-specific RNA-Seq dataset indicates that the expression of RNA transcripts corresponding to lymphatic or lymphatic-related markers is dramatically increased in induced pluripotent stem cells carrying the ApoE3 versus ApoE4 alleles [30]. ApoE4 induces premature contraction of mLVs, meningeal lymphosclerosis, and lymphedema, leading to reduced CSF drainage, accumulation of Aβ and other macromolecules and inflammatory mediators as well as immune cells in the brain, and promotes AD progression [30]. mLV ablation in 5×FAD mice leads to meningeal lymphatic dysfunction, which in turn affects CSF macromolecular influx and ISF efflux and its drainage to dCLNs [9]. Although no study has been conducted to assess the potential role of ApoE4 in the regulation of mLV function, the connection between ApoE4 and mLV function is interesting and needs further exploration.
mLV DYSFUNCTION DRIVES NEUROIMMUNE DYSFUNCTION
In addition to entering the nasal mucosa through a sieve plate and some other pathways, the dCLN pathway through mLVs is the predominant way for trafficking immune cells, cytokines, and CNS-derived antigens [90–93]. Therefore, mLV impairment accelerates the neuroinflammatory response because of the entrance of metabolic products, inflammatory cells, and cytokines. It remains unknown whether modulation of meningeal lymphatic function can influence the outcome of immunotherapy in AD. A recent study indicated that ablation of dorsal mLVs in 5xFAD mice worsens the outcome of mice treated with anti-Aβ antibodies by exacerbating Aβ deposition, microglial proliferation, neurovascular dysfunction, and behavioral deficits [31]. Microglia shift from a steady state toward a more inflammatory phenotype, leading to increased cytokine release and leukocyte migration [31]. Genomic analysis revealed a close relationship between injury of meningeal LECs and microglial activation in an AD mouse model [31]. LECs cooperate with microglia to remove waste from the brain [94]. In contrast, therapeutic delivery of VEGF-C improves Aβ clearance by monoclonal antibodies [31]. However, it was shown in experimental autoimmune encephalomyelitis mice that meningeal lymphatics govern inflammatory processes and immune surveillance of the CNS via brain-reactive T cells [41]. Attenuation was obtained after surgical and pharmacological blockade of lymphatic function, suggesting that drainage contributes to the activation of encephalitogenic T cells in the lymph nodes [41].
mLVs play a pivotal role in transporting immune cells [29]. A large number of T cells, such as CD11c+ cells and B220+ cells, are present in the meninges of naive mice [11, 95]. T cells enter the lymphatic vessels under physiological conditions and are transported to dCLNs through mLVs. It is important to identify and characterize the routes responsible for the drainage of CNS-derived antigens because modulation of these antigens may offer a valuable approach to the treatment of neuroinflammation. The entire trafficking process of immune cells via the mLVs to the draining lymph nodes primarily relies on the C-C motif chemokine receptor type 7- C-C motif chemokine ligand type 21 (CCR7-CCL21) pathway [41]. RNA-seq of meningeal LECs of the brain and spinal cord demonstrates the uniqueness of mLVs, which do not undergo morphological changes during inflammation and are notably different from the inflammatory environment that causes endothelial proliferation and swelling [41]. Reduced antigen efflux alleviates the immune response and tissue swelling. In addition to T cells, other immune cells, such as dendritic cells, B cells, and red blood cells, can also be drained through the dorsal and basal meningeal lymphatic endothelial cells [90–93]. Antigen presentation by lymphatic endothelial cells requires cooperation with dendritic cells that migrate in an amoeboid-like manner [90]. Indeed, inflammatory responses mainly occur in the early stages of neurodegenerative diseases. The level of AQP4 increases in the early stages of inflammation or injury, but this does not support lymphatic transport [64, 92]. The subsequent loss of AQP4 polarization is associated with reduced lymphatic flow [64]. CSF efflux is blocked when infiltrating immune cells accumulate in the perivascular space. Blockage of the flow of the lymphatic system and the subsequent accumulation of cytokines or other inflammatory mediators will create a vicious circle that keeps inflammation into a chronic, long-lasting state [96–98]. When the disease progresses to the later stage and is accompanied by continuous interruption of meningeal lymphatic drainage, the accumulation of inflammatory factors will accelerate the progression of AD [61, 92, 99]. Overall, mLV disorder disrupts the brain’s drainage system, which accelerates neuroinflammatory responses, possibly due to the accumulation of waste products or the retention of cytokines in the brain. When waste in the CNS accumulates to a certain level, it will in turn accelerate the destruction of the lymphatic system, thereby forming a vicious cycle.
MENINGEAL IMMUNOLOGY AND Aβ CLEARANCE
Recent works have shown that Aβ in the interstitial space and CSF follows a diurnal pattern: with the highest concentration in wakefulness and with the lowest concentration during sleep in both compartments [100, 101]. This regulation of protein concentrations may reflect a “turn-on” and “turn-off” pattern of glymphatic clearance during sleep and wakefulness [102]. Recent studies in humans and transgenic animal models have shown that the glymphatic system is highly activated during sleep and silent during wakefulness [103]. During wakefulness, the levels of extracellular metabolites including Aβ and tau increase. However, these aggregates are removed from the extracellular space by the convective flow of interstitial and para-arterial fluid in the paravenous space during sleep [103]. Meningeal ablation impairs mLVs, causes activation of myeloid cells, and increases Aβ levels in 5×FAD mice [31]. Aβ accumulation is increased after treatment with mAb158 (an Aβ selective monoclonal antibody) in lymphatic dysfunction, suggesting that Aβ immunotherapy alone cannot reverse the reduced Aβ clearance during lymphatic dysfunction [31, 104]. Reduced meningeal lymphatic drainage does not affect the expression of genes involved in Aβ transport in cerebral endothelial cells [31]. Enhancing meningeal lymphatic drainage of CSF macromolecules in aged mice by local injection of recombinant VEGF-C reduces Aβ deposition and improves cognitive function [105]. In 5×FAD mice, age-related deficiency of CCR7-dependent immune cells reduces lymphatic influx and increases Aβ accumulation and microglial hyperactivation [106]. Treatment of aged mice with anti-CD25 antibodies alleviates meningeal regulatory T-cell responses and improves cognitive function [106]. Intracerebroventricular delivery of AAV1-DSCR1 to 5×FAD mice facilitates the growth of dorsal meningeal lymphatics to improve drainage efficiency and protects against AD pathologies [107].
Cribriform plate LECs (cpLECs) exhibit a distinct phenotype and increased binding to leukocytes and upregulation of PD-L1 through IFN-γ [108]. The cross-talk between DCs, cpLECs, and CD4+ T cells and the regulation of molecules such as MHC II and PD-L1 identify cpLECs as an immunoregulatory niche [41, 108]. Hu et al. found that intracranial tumors induce extensive remodeling of dorsal mLVs [109]. Specific pharmacochemical ablation of dorsal mLVs significantly blocks the transport of dendritic cells from brain tumors to dCLNs and reduces the efficacy of anti-PD-1/CTLA-4 combination therapy [109]. Similarly, meningeal immunology may have great therapeutic potential in AD treatment [110]. A recent study reported the important role of the dural sinus as an immune surveillance center for soluble CNS-derived antigens in the CSF, allowing antigen-presenting cells and T cells to interact [111]. Under physical conditions, CSF antigens flow around the dura and are captured. This process is disrupted in the elderly [111]. These findings further deepen the understanding of CNS immunity in meningeal function. A recent study by Da Mesquita et al. reported a much more improved efficiency of a combination of meningeal lymphatic drainage and anti-Aβ monoclonal antibodies in clearing Aβ in 5×FAD mice [31]. However, side effects should also be considered, and vasogenic edema may occur after Aβ immunotherapy (e.g., aducanumab) [109, 112]. Significantly, recent literature reports that the dura appears to be less involved than expected in terms of autoimmune inflammation, as a lack of CNS autoantigens reaching the dura and a lack of pathogenic T-cell reactivation in the dura leads to a reduced release of proinflammatory factors [113]. Another reason is the limited functional connectivity between the parenchyma/leptomeninges and the dura [113]. However, since autoimmune inflammation targets neural tissue, the findings do not exclude a role for the dura in other immune-inflammatory pathological processes.
mLVs AND TAU PATHOLOGY
In addition to Aβ pathology, lymphatic drainage dysfunction has also been reported to play a role in tau deposition [10, 114, 115], especially when tau endocytosis fails. To study the clearance of extracellular tau from the brain, monomeric human tau conjugated with the near-infrared dye cypate was injected into the parenchyma of both wild-type and K14-VEGFR3-Ig transgenic mice, which lack a functional CNS lymphatic system [10]. The results show that a significantly higher amount of tau is retained in the brains of K14-VEGFR3-Ig, and its subsequent clearance to the periphery is delayed [10]. Studies in rTg4510 mice have revealed that tau proteins in the extracellular space are substrates for clearance via mLVs [115]. AQP4 plays a central role in tau clearance through the lymphatic system [115]. Impaired CSF/ISF exchange and AQP4 polarization exacerbate the aggregation of tau [10]. TGN-020 (an AQP4 inhibitor) dramatically impairs glymphatic CSF-ISF exchange and tau protein clearance [115]. AQP4 deficiency exacerbates glymphatic pathway dysfunction, not only increasing tau deposition in CSF but also promoting the development of neurofibrillary pathology and neurodegeneration [101].
CONCLUSION AND FUTURE PERSPECTIVES
The clearance of aggregated proteins in AD includes various pathways, including proteolysis and autophagy. Moreover, mLVs have recently been demonstrated to be an important waste clearance pathway in the brain and are involved in the pathological process of AD. Moreover, basal mLVs near the subarachnoid space are more likely to absorb CSF and play a more important role in Aβ clearance. The edema and sclerosis of mLVs associated with ApoE4 may exacerbate AD progression, but the exact mechanism remains to be elucidated. mLV dysfunction results in Aβ and tau accumulation and inflammatory reactions and increases microglial cytotoxicity, which in turn impairs glymphatic CSF/ISF exchange in the brain parenchyma and influences CSF/ISF transport to the peripheral circulation. Meanwhile, AQP4 is the driving force for the transportation of the glymphatic system and is indispensable for the clearance of pathological proteins. Focusing on the waste removal system, mLVs are an attractive alternative method for Aβ and tau clearance in AD. Given the function of mLVs in paravascular influx/efflux of CSF/ISF and in neuromodulatory effects of immune molecules, the activity of mLVs could alter the accessibility of CSF-borne immune neuromodulators to the brain parenchyma, thereby altering their effects on the brain [24]. Accordingly, mLVs can be viewed as a novel player in neurophysiology.
Visualization techniques such as MRI/PET/SPECT help to dynamically monitor CSF clearance and morphological changes in mLVs in aging and neurodegenerative diseases [14, 15, 116], which gives us a clearer view of the efficiency of macromolecules and waste clearance. Given that the mLVs are embedded within the dura and the arachnoid mater is supposedly impermeable to CSF, how can macromolecules and immune cells drain from the CSF into mLVs? Further experiments are necessary to answer this question. AQP4 and sleep are required for glymphatic clearance of waste products that build up in the awake. Conversely, lack of sleep and AQP4 deficiency are linked to reduced glymphatic clearance. However, it is still not understood why and how the absence of AQP4 reduces rates of solute elimination from the parenchyma. As such, the mechanisms by which sleep and AQP4 are linked to glymphatic clearance should be elucidated. Additionally, are there additional astrocytic channels, apart from AQP4, that facilitate CSF/ISF exchange during sleep? While dural lymphatic vessels may be important for the clearance of macromolecules from the brain, no change in intracranial pressure or brain water content was observed in mice lacking them [12]. Furthermore, the exact route taken by macromolecules and immune cells to exit the CNS, as well as the contribution of each route to the CNS-associated initiation of immune responses, are still a matter of debate.
Both mLV integrity and paravascular CSF influx/ISF efflux of solutes are impaired with aging. This could lead to a vicious cycle in which the clearance of macromolecules from the CSF is delayed [9, 29]. However, it remains unknown how mLVs contribute to the neuropathophysiological processes associated with aging. Taking into account the roles of the brain vascular endothelium and other components of the BBB, such as pericytes, in the excretion of Aβ from the brain, it would be interesting to determine whether dysfunction of the age-associated BBB and brain neurovascular unit affects meningeal lymphatic drainage. A similar situation should be considered to explore a possible connection between aging-related changes in meningeal lymphatic dysfunction and in the inflammatory processes and immune surveillance in AD. Additionally, whether mLVs affect the efficacy of current therapeutic strategies for AD, such as antibody-based treatments, needs to be further explored.
ACKNOWLEDGMENTS
The authors have no acknowledgments to report.
FUNDING
This work was supported by grants from the National Key Research and Development Program of China (2019YFE0115900), the National Natural Science Foundation of China (No. 81822016 and 81771382), and the Medical Science Advancement Program of Wuhan University (No. TFLC2018001).
CONFLICT OF INTEREST
The authors have no conflict of interest to report.
REFERENCES
[1] | Alzheimer’s Association ((2016) ) 2016 Alzheimer’s disease facts and figures. Alzheimers Dement 12: , 459–509. |
[2] | Knopman DS , Amieva H , Petersen RC , Chetelat G , Holtzman DM , Hyman BT , Nixon RA , Jones DT ((2021) ) Alzheimer disease. Nat Rev Dis Primers 7: , 33. |
[3] | Saido T , Leissring MA ((2012) ) Proteolytic degradation of amyloid β-protein. Cold Spring Harb Perspect Med 2: , a006379. |
[4] | Preston SD , Steart PV , Wilkinson A , Nicoll JA , Weller RO ((2003) ) Capillary and arterial cerebral amyloid angiopathy in Alzheimer’s disease: Defining the perivascular route for the elimination of amyloid beta from the human brain. Neuropathol Appl Neurobiol 29: , 106–117. |
[5] | Deane R , Du Yan S , Submamaryan RK , LaRue B , Jovanovic S , Hogg E , Welch D , Manness L , Lin C , Yu J , Zhu H , Ghiso J , Frangione B , Stern A , Schmidt AM , Armstrong DL , Arnold B , Liliensiek B , Nawroth P , Hofman F , Kindy M , Stern D , Zlokovic B ((2003) ) RAGE mediates amyloid-beta peptide transport across the blood-brain barrier and accumulation in brain. Nat Med 9: , 907–913. |
[6] | Weller RO , Subash M , Preston SD , Mazanti I , Carare RO ((2008) ) Perivascular drainage of amyloid-beta peptides from the brain and its failure in cerebral amyloid angiopathy and Alzheimer’s disease. Brain Pathol 18: , 253–266. |
[7] | Cheng Y , Wang YJ ((2020) ) Meningeal lymphatic vessels: A drain of the brain involved in neurodegeneration? Neurosci Bull 36: , 557–560. |
[8] | Wang J , Gu BJ , Masters CL , Wang YJ ((2017) ) A systemic view of Alzheimer disease - insights from amyloid-β metabolism beyond the brain. Nat Rev Neurol 13: , 703. |
[9] | Da Mesquita S , Louveau A , Vaccari A , Smirnov I , Cornelison RC , Kingsmore KM , Contarino C , Onengut-Gumuscu S , Farber E , Raper D , Viar KE , Powell RD , Baker W , Dabhi N , Bai R , Cao R , Hu S , Rich SS , Munson JM , Lopes MB , Overall CC , Acton ST , Kipnis J ((2018) ) Functional aspects of meningeal lymphatics in ageing and Alzheimer’s disease. Nature 560: , 185–191. |
[10] | Patel TK , Habimana-Griffin L , Gao X , Xu B , Achilefu S , Alitalo K , McKee CA , Sheehan PW , Musiek ES , Xiong C , Coble D , Holtzman DM ((2019) ) Dural lymphatics regulate clearance of extracellular tau from the CNS. Mol Neurodegener 14: , 11. |
[11] | Louveau A , Smirnov I , Keyes TJ , Eccles JD , Rouhani SJ , Peske JD , Derecki NC , Castle D , Mandell JW , Lee KS , Harris TH , Kipnis J ((2015) ) Structural and functional features of central nervous system lymphatic vessels. Nature 523: , 337–341. |
[12] | Aspelund A , Antila S , Proulx ST , Karlsen TV , Karaman S , Detmar M , Wiig H , Alitalo K ((2015) ) A dural lymphatic vascular system that drains brain interstitial fluid and macromolecules. J Exp Med 212: , 991–999. |
[13] | Absinta M , Ha SK , Nair G , Sati P , Luciano NJ , Palisoc M , Louveau A , Zaghloul KA , Pittaluga S , Kipnis J , Reich DS ((2017) ) Human and nonhuman primate meninges harbor lymphatic vessels that can be visualized noninvasively by MRI. Elife 6: , e29738. |
[14] | Verma A , Hesterman JY , Chazen JL , Holt R , Connolly P , Horky L , Vallabhajosula S , Mozley PD ((2020) ) Intrathecal (99m)Tc-DTPA imaging of molecular passage from lumbar cerebrospinal fluid to brain and periphery in humans. Alzheimers Dement (Amst) 12: , e12030. |
[15] | Shokri-Kojori E , Wang GJ , Wiers CE , Demiral SB , Guo M , Kim SW , Lindgren E , Ramirez V , Zehra A , Freeman C , Miller G , Manza P , Srivastava T , De Santi S , Tomasi D , Benveniste H , Volkow ND ((2018) ) β-amyloid accumulation in the human brain after one night of sleep deprivation. Proc Natl Acad Sci U S A 115: , 4483–4488. |
[16] | Visanji NP , Lang AE , Munoz DG ((2018) ) Lymphatic vasculature in human dural superior sagittal sinus: Implications for neurodegenerative proteinopathies. Neurosci Lett 665: , 18–21. |
[17] | Buckley MW , McGavern DB ((2022) ) Immune dynamics in the CNS and its barriers during homeostasis and disease. Immunol Rev 306: , 58–75. |
[18] | Tamura R , Yoshida K , Toda M ((2020) ) Current understanding of lymphatic vessels in the central nervous system. Neurosurg Rev 43: , 1055–1064. |
[19] | Tarasoff-Conway JM , Carare RO , Osorio RS , Glodzik L , Butler T , Fieremans E , Axel L , Rusinek H , Nicholson C , Zlokovic BV , Frangione B , Blennow K , Ménard J , Zetterberg H , Wisniewski T , de Leon MJ ((2015) ) Clearance systems in the brain-implications for Alzheimerdisease. Nat Rev Neurol 11: , 457–470. |
[20] | Pascale CL , Miller MC , Chiu C , Boylan M , Caralopoulos IN , Gonzalez L , Johanson CE , Silverberg GD ((2011) ) Amyloid-beta transporter expression at the blood-CSF barrier is age-dependent. Fluids Barriers CNS 8: , 21. |
[21] | Iliff JJ , Goldman SA , Nedergaard M ((2015) ) Implications of the discovery of brain lymphatic pathways. Lancet Neurol 14: , 977–979. |
[22] | Louveau A , Plog BA , Antila S , Alitalo K , Nedergaard M , Kipnis J ((2017) ) Understanding the functions and relationships of the glymphatic system and meningeal lymphatics. J Clin Invest 127: , 3210–3219. |
[23] | Jaffe RJ , Dave RS , Byrareddy SN ((2019) ) Meningeal lymphatics in aging and Alzheimer’s disease. Ann Transl Med 7: , S2. |
[24] | Da Mesquita S , Fu Z , Kipnis J ((2018) ) The meningeal lymphatic system: A new player in neurophysiology. Neuron 100: , 375–388. |
[25] | Antila S , Karaman S , Nurmi H , Airavaara M , Voutilainen MH , Mathivet T , Chilov D , Li Z , Koppinen T , Park JH , Fang S , Aspelund A , Saarma M , Eichmann A , Thomas JL , Alitalo K ((2017) ) Development and plasticity of meningeal lymphatic vessels. J Exp Med 214: , 3645–3667. |
[26] | Forstmann BU , de Hollander G , van Maanen L , Alkemade A , Keuken MC ((2016) ) Towards a mechanistic understanding of the human subcortex. Nat Rev Neurosci 18: , 57–65. |
[27] | Petrova TV , Koh GY ((2018) ) Organ-specific lymphatic vasculature: From development to pathophysiology. J Exp Med 215: , 35–49. |
[28] | Margaris KN , Black RA ((2012) ) Modelling the lymphatic system: Challenges and opportunities. J R Soc Interface 9: , 601–612. |
[29] | Ahn JH , Cho H , Kim JH , Kim SH , Ham JS , Park I , Suh SH , Hong SP , Song JH , Hong YK , Jeong Y , Park SH , Koh GY ((2019) ) Meningeal lymphatic vessels at the skull base drain cerebrospinal fluid. Nature 572: , 62–66. |
[30] | Mentis A-FA , Dardiotis E , Chrousos GP ((2021) ) Apolipoprotein E4 and meningeal lymphatics in Alzheimer disease: A conceptual framework. Mol Psychiatry 26: , 1075–1097. |
[31] | Da Mesquita S , Papadopoulos Z , Dykstra T , Brase L , Farias FG , Wall M , Jiang H , Kodira CD , de Lima KA , Herz J , Louveau A , Goldman DH , Salvador AF , Onengut-Gumuscu S , Farber E , Dabhi N , Kennedy T , Milam MG , Baker W , Smirnov I , Rich SS , Benitez BA , Karch CM , Perrin RJ , Farlow M , Chhatwal JP , Holtzman DM , Cruchaga C , Harari O , Kipnis J ((2021) ) Meningeal lymphatics affect microglia responses and anti-Aβ immunotherapy. Nature 593: , 255–260. |
[32] | Knopf PM , Cserr HF , Nolan SC , Wu TY , Harling-Berg CJ ((1995) ) Physiology and immunology of lymphatic drainage of interstitial and cerebrospinal fluid from the brain. Neuropathol Appl Neurobiol 21: , 175–180. |
[33] | Silver I , Kim C , Mollanji R , Johnston M ((2002) ) Cerebrospinal fluid outflow resistance in sheep: Impact of blocking cerebrospinal fluid transport through the cribriform plate. Neuropathol Appl Neurobiol 28: , 67–74. |
[34] | Johnston M , Zakharov A , Papaiconomou C , Salmasi G , Armstrong D ((2004) ) Evidence of connections between cerebrospinal fluid and nasal lymphatic vessels in humans, non-human primates and other mammalian species. Cerebrospinal Fluid Res 1: , 2. |
[35] | Jacob L , Boisserand LSB , Geraldo LHM , de Brito Neto J , Mathivet T , Antila S , Barka B , Xu Y , Thomas JM , Pestel J , Aigrot MS , Song E , Nurmi H , Lee S , Alitalo K , Renier N , Eichmann A , Thomas JL ((2019) ) Anatomy and function of the vertebral column lymphatic network in mice. Nat Commun 10: , 4594. |
[36] | Koh L , Zakharov A , Johnston M ((2005) ) Integration of the subarachnoid space and lymphatics: Is it time to embrace a new concept of cerebrospinal fluid absorption? Cerebrospinal Fluid Res 2: , 6. |
[37] | Koh L , Zakharov A , Nagra G , Armstrong D , Friendship R , Johnston M ((2006) ) Development of cerebrospinal fluid absorption sites in the pig and rat: Connections between the subarachnoid space and lymphatic vessels in the olfactory turbinates. Anat Embryol (Berl) 211: , 335–344. |
[38] | McComb JG ((1983) ) Recent research into the nature of cerebrospinal fluid formation and absorption. J Neurosurg 59: , 369–383. |
[39] | Plog BA , Nedergaard M ((2018) ) The glymphatic system in central nervous system health and disease: Past, present, and future. Annu Rev Pathol 13: , 379–394. |
[40] | Louveau A , Harris TH , Kipnis J ((2015) ) Revisiting the mechanisms of CNS immune privilege. Trends Immunol 36: , 569–577. |
[41] | Louveau A , Herz J , Alme MN , Salvador AF , Dong MQ , Viar KE , Herod SG , Knopp J , Setliff JC , Lupi AL , Da Mesquita S , Frost EL , Gaultier A , Harris TH , Cao R , Hu S , Lukens JR , Smirnov I , Overall CC , Oliver G , Kipnis J ((2018) ) CNS lymphatic drainage and neuroinflammation are regulated by meningeal lymphatic vasculature. Nat Neurosci 21: , 1380–1391. |
[42] | Rangroo Thrane V , Thrane AS , Plog BA , Thiyagarajan M , Iliff JJ , Deane R , Nagelhus EA , Nedergaard M ((2013) ) Paravascularmicrocirculation facilitates rapid lipid transport and astrocytesignaling in the brain. Sci Rep 3: , 2582. |
[43] | Iliff JJ , Wang M , Zeppenfeld DM , Venkataraman A , Plog BA , Liao Y , Deane R , Nedergaard M ((2013) ) Cerebral arterial pulsation drives paravascular CSF-interstitial fluid exchange in the murine brain. J Neurosci 33: , 18190–18199. |
[44] | Iliff JJ , Wang M , Liao Y , Plogg BA , Peng W , Gundersen GA , Benveniste H , Vates GE , Deane R , Goldman SA , Nagelhus EA , Nedergaard M ((2012) ) A paravascular pathway facilitates CSF flow through the brain parenchyma and the clearance of interstitial solutes, including amyloid β. Sci Transl Med 4: , 147ra111. |
[45] | Yang L , Kress BT , Weber HJ , Thiyagarajan M , Wang B , Deane R , Benveniste H , Iliff JJ , Nedergaard M ((2013) ) Evaluating glymphatic pathway function utilizing clinically relevant intrathecal infusion of CSF tracer. J Transl Med 11: , 107. |
[46] | Hladky SB , Barrand MA ((2022) ) The glymphatic hypothesis: The theory and the evidence. Fluids Barriers CNS 19: , 9. |
[47] | Bolte AC , Dutta AB , Hurt ME , Smirnov I , Kovacs MA , McKee CA , Ennerfelt HE , Shapiro D , Nguyen BH , Frost EL , Lammert CR , Kipnis J , Lukens JR ((2020) ) Meningeal lymphatic dysfunction exacerbates traumatic brain injury pathogenesis. Nat Commun 11: , 4524. |
[48] | Martinez-Valbuena I , Valenti-Azcarate R , Amat-Villegas I , Riverol M , Marcilla I , de Andrea CE , Sanchez-Arias JA , Del Mar Carmona-Abellan M , Marti G , Erro ME , Martinez-Vila E , Tunon MT , Luquin MR ((2019) ) Amylin as a potential link between type 2 diabetes and alzheimer disease. Ann Neurol 86: , 539–551. |
[49] | Ma Q , Decker Y , Müller A , Ineichen BV , Proulx ST ((2019) ) Clearance of cerebrospinal fluid from the sacral spine through lymphatic vessels. J Exp Med 216: , 2492–2502. |
[50] | Thibaudeau TA , Anderson RT , Smith DM ((2018) ) A common mechanism of proteasome impairment by neurodegenerative disease-associated oligomers. Nat Commun 9: , 1097. |
[51] | Rasmussen MK , Mestre H , Nedergaard M ((2018) ) The glymphatic pathway in neurological disorders. Lancet Neurol 17: , 1016–1024. |
[52] | Sakka L , Coll G , Chazal J ((2011) ) Anatomy and physiology of cerebrospinal fluid. Eur Ann Otorhinolaryngol Head Neck Dis 128: , 309–316. |
[53] | Ma Q , Ries M , Decker Y , Müller A , Riner C , Bücker A , Fassbender K , Detmar M , Proulx ST ((2019) ) Rapid lymphatic efflux limits cerebrospinal fluid flow to the brain. Acta Neuropathol 137: , 151–165. |
[54] | Ringstad G , Vatnehol SAS , Eide PK ((2017) ) Glymphatic MRI in idiopathic normal pressure hydrocephalus. Brain 140: , 2691–2705. |
[55] | Lin Y , Jin J , Lv R , Luo Y , Dai W , Li W , Tang Y , Wang Y , Ye X , Lin WJ ((2021) ) Repetitive transcranial magnetic stimulation increases the brain’s drainage efficiency in a mouse model of Alzheimer’s disease. Acta Neuropathol Commun 9: , 102. |
[56] | Semyachkina-Glushkovskaya O , Khorovodov A , Fedosov I , Pavlov A , Shirokov A , Sharif AE , Dubrovsky A , Blokhina I , Terskov A , Navolokin N , Evsukova A , Karandin G , Elovenko D , Tzoy M , Ageev V , Agranovich I , Telnova V , Tsven A , Saranceva E , Iskra T , Kurths J ((2021) ) A novel method to stimulate lymphatic clearance of beta-amyloid from mouse brain using noninvasive music-induced opening of the blood–brain barrier with EEG markers. Applied Sciences 11: , 10287. |
[57] | Sachdeva S , Persaud S , Patel M , Popard P , Colverson A , Doré S ((2022) ) Effects of sound interventions on the permeability of theblood-brain barrier and meningeal lymphatic clearance. Brain Sci 12: , 742. |
[58] | Wang L , Zhang Y , Zhao Y , Marshall C , Wu T , Xiao M ((2019) ) Deep cervical lymph node ligation aggravates AD-like pathology of APP/PS1 mice. Brain Pathol 29: , 176–192. |
[59] | Hablitz LM , Plá V , Giannetto M , Vinitsky HS , Stæger FF , Metcalfe T , Nguyen R , Benrais A , Nedergaard M ((2020) ) Circadian control of brain glymphatic and lymphatic fluid flow. Nat Commun 11: , 4411. |
[60] | Xie L , Kang H , Xu Q , Chen MJ , Liao Y , Thiyagarajan M , O’Donnell J , Christensen DJ , Nicholson C , Iliff JJ , Takano T , Deane R , Nedergaard M ((2013) ) Sleep drives metabolite clearance from the adult brain. Science 342: , 373–377. |
[61] | Nedergaard M , Goldman SA ((2020) ) Glymphatic failure as a final common pathway to dementia. Science 370: , 50–56. |
[62] | Bishir M , Bhat A , Essa MM , Ekpo O , Ihunwo AO , Veeraraghavan VP , Mohan SK , Mahalakshmi AM , Ray B , Tuladhar S , Chang S , Chidambaram SB , Sakharkar MK , Guillemin GJ , Qoronfleh MW , Ojcius DM ((2020) ) Sleep deprivation and neurological disorders. Biomed Res Int 2020: , 5764017. |
[63] | Louveau A , Da Mesquita S , Kipnis J ((2016) ) Lymphatics in neurological disorders: A neuro-lympho-vascular component of multiple sclerosis and Alzheimer’s disease? Neuron 91: , 957–973. |
[64] | Kress BT , Iliff JJ , Xia M , Wang M , Wei HS , Zeppenfeld D , Xie L , Kang H , Xu Q , Liew JA , Plog BA , Ding F , Deane R , Nedergaard M ((2014) ) Impairment of paravascular clearance pathways in the aging brain. Ann Neurol 76: , 845–861. |
[65] | Ma Q , Ineichen BV , Detmar M , Proulx ST ((2017) ) Outflow of cerebrospinal fluid is predominantly through lymphatic vessels and is reduced in aged mice. Nat Commun 8: , 1434. |
[66] | Silva I , Silva J , Ferreira R , Trigo D ((2021) ) Glymphatic system, AQP4, and their implications in Alzheimer’s disease. Neurol Res Pract 3: , 5. |
[67] | Mestre H , Hablitz LM , Xavier AL , Feng W , Zou W , Pu T , Monai H , Murlidharan G , Castellanos Rivera RM , Simon MJ , Pike MM , Plá V , Du T , Kress BT , Wang X , Plog BA , Thrane AS , Lundgaard I , Abe Y , Yasui M , Thomas JH , Xiao M , Hirase H , Asokan A , Iliff JJ , Nedergaard M ((2018) ) Aquaporin-4-dependent glymphatic solute transport in therodent brain. Elife 7: , e40070. |
[68] | Papadopoulos MC , Verkman AS ((2013) ) Aquaporin water channels in the nervous system. Nat Rev Neurosci 14: , 265–277. |
[69] | Yang J , Zhang R , Shi C , Mao C , Yang Z , Suo Z , Torp R , Xu Y ((2017) ) AQP4 association with amyloid deposition and astrocyte pathology in the Tg-ArcSwe mouse model of Alzheimer’s disease. J Alzheimers Dis 57: , 157–169. |
[70] | Cao X , Xu H , Feng W , Su D , Xiao M ((2018) ) Deletion of aquaporin-4 aggravates brain pathology after blocking of the meningeal lymphatic drainage. Brain Res Bull 143: , 83–96. |
[71] | Zeppenfeld DM , Simon M , Haswell JD , D’Abreo D , Murchison C , Quinn JF , Grafe MR , Woltjer RL , Kaye J , Iliff JJ ((2017) ) Association of perivascular localization of aquaporin-4 with cognition and Alzheimer disease in aging brains. JAMA Neurol 74: , 91–99. |
[72] | Xu Z , Xiao N , Chen Y , Huang H , Marshall C , Gao J , Cai Z , Wu T , Hu G , Xiao M ((2015) ) Deletion of aquaporin-4 in APP/PS1 mice exacerbates brain Aβ accumulation and memory deficits. Mol Neurodegener 10: , 58. |
[73] | Smith AJ , Yao X , Dix JA , Jin BJ , Verkman AS ((2017) ) Test of the ‘glymphatic’ hypothesis demonstrates diffusive and aquaporin-4-independent solute transport in rodent brain parenchyma. Elife 6: , e27679. |
[74] | Love S , Miners JS ((2016) ) Cerebrovascular disease in ageing and Alzheimer’s disease. Acta Neuropathol 131: , 645–658. |
[75] | Knopman DS , Jack CR Jr, Wiste HJ , Weigand SD , Vemuri P , Lowe VJ , Kantarci K , Gunter JL , Senjem ML , Mielke MM , Machulda MM , Roberts RO , Boeve BF , Jones DT , Petersen RC ((2016) ) Age and neurodegeneration imaging biomarkers in persons with Alzheimer disease dementia. Neurology 87: , 691–698. |
[76] | Hawkes CA , Hartig W , Kacza J , Schliebs R , Weller RO , Nicoll JA , Carare RO ((2011) ) Perivascular drainage of solutes is impaired in the ageing mouse brain and in the presence of cerebral amyloid angiopathy. Acta Neuropathol 121: , 431–443. |
[77] | Hawkes CA , Gatherer M , Sharp MM , Dorr A , Yuen HM , Kalaria R , Weller RO , Carare RO ((2013) ) Regional differences in the morphological and functional effects of aging on cerebral basement membranes and perivascular drainage of amyloid-beta from the mouse brain. Aging Cell 12: , 224–236. |
[78] | Lardenoije R , Iatrou A , Kenis G , Kompotis K , Steinbusch HW , Mastroeni D , Coleman P , Lemere CA , Hof PR , van den Hove DL , Rutten BP ((2015) ) The epigenetics of aging and neurodegeneration. Prog Neurobiol 131: , 21–64. |
[79] | Smith DK , He M , Zhang CL , Zheng JC ((2017) ) The therapeutic potential of cell identity reprogramming for the treatment of aging-related neurodegenerative disorders. Prog Neurobiol 157: , 212–229. |
[80] | Lanfranco MF , Ng CA , Rebeck GW ((2020) ) ApoE lipidation as a therapeutic target in Alzheimer’s disease. Int J Mol Sci 21: , 6336. |
[81] | Pitas RE , Boyles JK , Lee SH , Hui D , Weisgraber KH ((1987) ) Lipoproteins and their receptors in the central nervous system. Characterization of the lipoproteins in cerebrospinal fluid and identification of apolipoprotein B,E(LDL) receptors in the brain. J Biol Chem 262: , 14352–14360. |
[82] | Rebeck GW , Alonzo NC , Berezovska O , Harr SD , Knowles RB , Growdon JH , Hyman BT , Mendez AJ ((1998) ) Structure and functions of human cerebrospinal fluid lipoproteins from individuals of different APOE genotypes. Exp Neurol 149: , 175–182. |
[83] | Verghese PB , Castellano JM , Garai K , Wang Y , Jiang H , Shah A , Bu G , Frieden C , Holtzman DM ((2013) ) ApoE influences amyloid-β (Aβ) clearance despite minimal apoE/Aβ association in physiological conditions. Proc Natl Acad Sci U S A 110: , E1807–1816. |
[84] | Wisniewski T , Drummond E ((2020) ) APOE-amyloid interaction:Therapeutic targets. Neurobiol Dis 138: , 104784. |
[85] | Deane R , Sagare A , Hamm K , Parisi M , Lane S , Finn MB , Holtzman DM , Zlokovic BV ((2008) ) apoE isoform-specific disruption of amyloid beta peptide clearance from mouse brain. J Clin Invest 118: , 4002–4013. |
[86] | Lim HY , Thiam CH , Yeo KP , Bisoendial R , Hii CS , McGrath KC , Tan KW , Heather A , Alexander JS , Angeli V ((2013) ) Lymphatic vessels are essential for the removal of cholesterol from peripheral tissues by SR-BI-mediated transport of HDL. Cell Metab 17: , 671–684. |
[87] | Tammela T , Alitalo K ((2010) ) Lymphangiogenesis: Molecular mechanisms and future promise. Cell 140: , 460–476. |
[88] | Baloyannis SJ , Baloyannis IS ((2012) ) The vascular factor in Alzheimer’s disease: A study in Golgi technique and electron microscopy. J Neurol Sci 322: , 117–121. |
[89] | Lim HY , Rutkowski JM , Helft J , Reddy ST , Swartz MA , Randolph GJ , Angeli V ((2009) ) Hypercholesterolemic mice exhibit lymphatic vessel dysfunction and degeneration. Am J Pathol 175: , 1328–1337. |
[90] | Randolph GJ , Ivanov S , Zinselmeyer BH , Scallan JP ((2017) ) The lymphatic system: Integral roles in immunity. Annu Rev Immunol 35: , 31–52. |
[91] | van Lessen M , Shibata-Germanos S , van Impel A , Hawkins TA , Rihel J , Schulte-Merker S ((2017) ) Intracellular uptake of macromolecules by brain lymphatic endothelial cells during zebrafish embryonic development. Elife 6: , e25932. |
[92] | Mogensen FL , Delle C , Nedergaard M ((2021) ) The glymphatic system (en)during inflammation. Int J Mol Sci 22: , 7491. |
[93] | Suárez I , Schulte-Merker S ((2021) ) Cells with many talents:Lymphatic endothelial cells in the brain meninges. Cells 10: , 799. |
[94] | Huisman Y , Uphoff K , Berger M , Dobrindt U , Schelhaas M , Zobel T , Bussmann J , van Impel A , Schulte-Merker S ((2022) ) Meningeal lymphatic endothelial cells fulfill scavenger endothelial cell function and cooperate with microglia in waste removal from the brain. Glia 70: , 35–49. |
[95] | Ribeiro M , Brigas HC , Temido-Ferreira M , Pousinha PA , Regen T , Santa C , Coelho JE , Marques-Morgado I , Valente CA , Omenetti S , Stockinger B , Waisman A , Manadas B , Lopes LV , Silva-Santos B , Ribot JC ((2019) ) Meningeal γδ T cell-derived IL-17 controls synaptic plasticity and short-term memory. Sci Immunol 4: , eaay5199. |
[96] | Jha MK , Kim JH , Song GJ , Lee WH , Lee IK , Lee HW , An SSA , Kim S , Suk K ((2018) ) Functional dissection of astrocyte-secreted proteins: Implications in brain health and diseases. Prog Neurobiol 162: , 37–69. |
[97] | Liddelow SA , Guttenplan KA , Clarke LE , Bennett FC , Bohlen CJ , Schirmer L , Bennett ML , Münch AE , Chung WS , Peterson TC , Wilton DK , Frouin A , Napier BA , Panicker N , Kumar M , Buckwalter MS , Rowitch DH , Dawson VL , Dawson TM , Stevens B , Barres BA ((2017) ) Neurotoxic reactive astrocytes are induced by activated microglia. Nature 541: , 481–487. |
[98] | Hinkerohe D , Smikalla D , Schoebel A , Haghikia A , Zoidl G , Haase CG , Schlegel U , Faustmann PM ((2010) ) Dexamethasone prevents LPS-induced microglial activation and astroglial impairment in an experimental bacterial meningitis co-culture model. Brain Res 1329: , 45–54. |
[99] | Molteni M , Rossetti C ((2017) ) Neurodegenerative diseases: The immunological perspective. J Neuroimmunol 313: , 109–115. |
[100] | Kang JE , Lim MM , Bateman RJ , Lee JJ , Smyth LP , Cirrito JR , Fujiki N , Nishino S , Holtzman DM ((2009) ) Amyloid-beta dynamics are regulated by orexin and the sleep-wake cycle. Science 326: , 1005–1007. |
[101] | Holth JK , Fritschi SK , Wang C , Pedersen NP , Cirrito JR , Mahan TE , Finn MB , Manis M , Geerling JC , Fuller PM , Lucey BP , Holtzman DM ((2019) ) The sleep-wake cycle regulates brain interstitial fluid tau in mice and CSF tau in humans. Science 363: , 880–884. |
[102] | Hauglund NL , Kusk P , Kornum BR , Nedergaard M ((2020) ) Meningeal lymphangiogenesis and enhanced glymphatic activity in mice with chronically implanted EEG electrodes. J Neurosci 40: , 2371–2380. |
[103] | Elvsashagen T , Mutsaerts HJ , Zak N , Norbom LB , Quraishi SH , Pedersen PO , Malt UF , Westlye LT , van Someren EJ , Bjornerud A , Groote IR ((2019) ) Cerebral blood flow changes after a day of wake, sleep, and sleep deprivation. Neuroimage 186: , 497–509. |
[104] | Laaker C , Fabry Z ((2021) ) The meningeal lymphatics: Regulators of Aβ immunotherapy? Trends Immunol 42: , 940–942. |
[105] | Wen YR , Yang JH , Wang X , Yao ZB ((2018) ) Induced dural lymphangiogenesis facilities soluble amyloid-beta clearance from brain in a transgenic mouse model of Alzheimer’s disease. Neural Regen Res 13: , 709–716. |
[106] | Da Mesquita S , Herz J , Wall M , Dykstra T , de Lima KA , Norris GT , Dabhi N , Kennedy T , Baker W , Kipnis J ((2021) ) Aging-associated deficit in CCR7 is linked to worsened glymphatic function, cognition, neuroinflammation, and β-amyloid pathology. Sci Adv 7: , eabe4601. |
[107] | Choi C , Park J , Kim H , Chang KT , Park J , Min KT ((2021) ) DSCR1 upregulation enhances dural meningeal lymphatic drainage to attenuate amyloid pathology of Alzheimer’s disease. J Pathol 255: , 296–310. |
[108] | Hsu M , Laaker C , Madrid A , Herbath M , Choi YH , Sandor M , Fabry Z ((2022) ) Neuroinflammation creates an immune regulatory niche at the meningeal lymphatic vasculature near the cribriform plate. Nat Immunol 23: , 581–593. |
[109] | Hu X , Deng Q , Ma L , Li Q , Chen Y , Liao Y , Zhou F , Zhang C , Shao L , Feng J , He T , Ning W , Kong Y , Huo Y , He A , Liu B , Zhang J , Adams R , He Y , Tang F , Bian X , Luo J ((2020) ) Meningeal lymphatic vessels regulate brain tumor drainage and immunity. Cell Res 30: , 229–243. |
[110] | Ma T , Wang F , Xu S , Huang JH ((2021) ) Meningeal immunity: Structure, function and a potential therapeutic target of neurodegenerative diseases. Brain Behav Immun 93: , 264–276. |
[111] | Rustenhoven J , Drieu A , Mamuladze T , de Lima KA , Dykstra T , Wall M , Papadopoulos Z , Kanamori M , Salvador AF , Baker W , Lemieux M , Da Mesquita S , Cugurra A , Fitzpatrick J , Sviben S , Kossina R , Bayguinov P , Townsend RR , Zhang Q , Erdmann-Gilmore P , Smirnov I , Lopes MB , Herz J , Kipnis J ((2021) ) Functional characterization of the dural sinuses as a neuroimmune interface. Cell 184: , 1000–1016.e1027. |
[112] | Tolar M , Abushakra S , Hey JA , Porsteinsson A , Sabbagh M ((2020) ) Aducanumab, gantenerumab, BAN2401, and ALZ-801-the first wave of amyloid-targeting drugs for Alzheimer’s disease with potential for near term approval. Alzheimers Res Ther 12: , 95. |
[113] | Merlini A , Haberl M , Strauß J , Hildebrand L , Genc N , Franz J , Chilov D , Alitalo K , Flügel-Koch C , Stadelmann C , Flügel A , Odoardi F ((2022) ) Distinct roles of the meningeal layers in CNS autoimmunity. Nat Neurosci 25: , 887–899. |
[114] | Iliff JJ , Chen MJ , Plog BA , Zeppenfeld DM , Soltero M , Yang L , Singh I , Deane R , Nedergaard M ((2014) ) Impairment of glymphatic pathway function promotes tau pathology after traumatic brain injury. J Neurosci 34: , 16180–16193. |
[115] | Harrison IF , Ismail O , Machhada A , Colgan N , Ohene Y , Nahavandi P , Ahmed Z , Fisher A , Meftah S , Murray TK , Ottersen OP , Nagelhus EA , O’Neill MJ , Wells JA , Lythgoe MF ((2020) ) Impaired glymphatic function and clearance of tau in an Alzheimer’s disease model. Brain 143: , 2576–2593. |
[116] | Li G , Cao Y , Tang X , Huang J , Cai L , Zhou L ((2022) ) The meningeal lymphatic vessels and the glymphatic system: Potential therapeutic targets in neurological disorders. J Cereb Blood Flow Metab 42: , 1364–1382. |