High Cholesterol Diet Exacerbates Blood-Brain Barrier Disruption in LDLr–/– Mice: Impact on Cognitive Function
Abstract
Background:
Evidence has revealed an association between familial hypercholesterolemia and cognitive impairment. In this regard, a connection between cognitive deficits and hippocampal blood-brain barrier (BBB) breakdown was found in low-density lipoprotein receptor knockout mice (LDLr–/–), a mouse model of familial hypercholesterolemia.
Objective:
Herein we investigated the impact of a hypercholesterolemic diet on cognition and BBB function in C57BL/6 wild-type and LDLr–/–mice.
Methods:
Animals were fed with normal or high cholesterol diets for 30 days. Thus, wild-type and LDLr–/–mice were submitted to memory paradigms. Additionally, BBB integrity was evaluated in the mice’s prefrontal cortices and hippocampi.
Results:
A tenfold elevation in plasma cholesterol levels of LDLr–/–mice was observed after a hypercholesterolemic diet, while in wild-type mice, the hypercholesterolemic diet exposure increased plasma cholesterol levels only moderately and did not induce cognitive impairment. LDLr–/–mice presented memory impairment regardless of the diet. We observed BBB disruption as an increased permeability to sodium fluorescein in the prefrontal cortices and hippocampi and a decrease on hippocampal claudin-5 and occludin mRNA levels in both wild-type and LDLr–/–mice treated with a hypercholesterolemic diet. The LDLr–/–mice fed with a regular diet already presented BBB dysfunction. The BBB-increased leakage in the hippocampi of LDLr–/–mice was related to high microvessel content and intense astrogliosis, which did not occur in the control mice.
Conclusion:
Therefore, LDLr–/–mice seem to be more susceptible to cognitive impairments and BBB damage induced by exposure to a high cholesterol diet. Finally, BBB disruption appears to be a relevant event in hypercholesterolemia-induced brain alterations.
INTRODUCTION
Cholesterol is an important cell membrane component, as well as a precursor of bile acids and steroid hormones, making it essential to all animal life [1]. However, hypercholesterolemia, the term for an increase in blood cholesterol levels, is a well-documented risk factor for cardiovascular diseases of atherosclerotic origin [2]. Familial hypercholesterolemia (FH), a genetic form of hypercholesterolemia, is characterized by severely elevated low-density lipoprotein (LDL) cholesterol levels that lead to premature and progressive atherosclerotic plaque deposition in the coronary arteries and proximal aorta. According to population data, the heterozygous and homozygous forms of FH affect one in 200 and one in 300,000 people, respectively, worldwide. FH is inherited in an autosomal dominant way and is caused mainly by mutations in the LDL receptor (LDLr) gene [3–5].
FH represents a unique opportunity to study the effects of cholesterol metabolism on cognition over time, because affected individuals are exposed to hypercholesterolemia from early in life and carry a dysfunction of LDLr [6, 7]. One clinical study revealed that middle-aged individuals with FH were more likely to exhibit abnormal cognitive functions that meet the criteria for mild cognitive impairment (MCI) [6]. Recent findings have reported neuropsychological deficits, including memory decline, in young FH individuals aged between 18 and 40 years old [7]. We and others corroborated these clinical observations when we showed that LDLr knockout (LDLr–/–) mice, an experimental FH mouse model, displayed cognitive impairment [8, 9]. As early as three months of age, the LDLr–/– mice displayed spatial and working memory impairments in several behavioral tests, e.g., object location, the Morris water maze, and spontaneous alternation tasks [9–12].
Other studies have reported that a high-cholesterol diet induces cognitive impairment in rodent models [13–15]. It is worth noting that the impact of the high cholesterol diet seems to be more intense in LDLr–/– mice. A previous study reported a higher neuroinflammatory response, i.e., glial activation and increased expression of inflammatory cytokines/mediators, in LDLr–/– mice fed with a high cholesterol diet than in wild-type mice fed with the same diet [13]. A recent study suggested that LDLr–/– mice exposed to a Western diet containing a high percentage of cholesterol, fat, and sucrose presented impaired blood-brain barrier (BBB) transport in Gd-DTPA contrast magnetic resonance imaging (MRI) analysis, as well as neuroinflammation [15].
Also, we found that the LDLr–/– mice were more susceptible to amyloid-β peptide (Aβ)-induced neurotoxic effects. The Aβ1–40 (i.c.v)-treated LDLr–/– mice revealed more severe memory dysfunction, disrupted acetylcholinesterase activity, astrogliosis, oxidative imbalance, and cell membrane injury within their hippocampi than identically treated wild-type C57BL/6 mice. Interestingly, we also observed that the LDLr–/– mice per se presented increased BBB permeability in their hippocampi, which was indicated as an increased aquaporin -4 (AQP-4) immunoreactivity within this structure [10]. On the other hand, there were no alterations on Aβ metabolism and content in brains of young and middle-aged LDLr–/– mice, but an exacerbation of neuronal apoptosis [11]. Therefore, BBB dysfunction and further neuroinflammation appear to be the cause of neurodegeneration and consequent cognitive decline in FH.
BBB regulates the transport of molecules into and out of the central nervous system (CNS), which maintains a tight control over the chemical composition of the neuronal milieu that is required for proper neuronal functioning [16]. In this sense, one may suppose that BBB breakdown and dysfunction caused by hypercholesterolemia generates a neurotoxic milieu, which contributes to cognitive impairment.
The purpose of this study was to investigate the impact of exposure to a high cholesterol diet on cognitive function, BBB integrity, and neuroinflammation in LDLr–/– and C57BL/6 wild-type mice. We also intended to find out more about the mechanisms underlying BBB disruption and the implications for cognitive dysfunction in FH. A thirty-day treatment with a high cholesterol diet was used to exacerbate hypercholesterolemia in the experimental animals.
METHODS
Animals
C57BL/6 wild-type and LDLr knockout (LDLr–/–; B6.129S7- Ldlrtm1Her/J) mice founders were purchased from Jackson Laboratory (Bar Harbor, ME) and were bred at Federal University of Santa Catarina (UFSC, Florianópolis, Brazil). Animals were maintained in groups of four to five animals per cage, under controlled temperature (23±1°C) and a 12 h light cycle (lights on 7:00 a.m.), with free access to food and water. In this study, we used male three-month-old wild-type and LDLr–/– mice divided into four experimental groups (approximately 24 per group), totalizing 96 animals. Procedures used in the present study complied with the guidelines on animal care of the local Ethics Committee on the Use of Animals (CEUA/UFSC), which follows the NIH publication “Principles of Laboratory Animal Care”. Process Number of Ethical Committee approval: PP00948.
Experimental protocol
In order to investigate the impact of hypercholesterolemia on BBB integrity and neuroinflammation and its relationship with the cognitive performance, three-month-old male C57BL/6 wild-type and LDLr–/– mice were fed for thirty days with a standard (Nuvilab CR1, Nuvital; Quimtia Nutrientes SA, Colombo, PR, Brazil) or with a hypercholesterolemic diet (20% fat and 1.25% cholesterol; produced at the State University of Campinas, Campinas, SP, Brazil) [9]. Afterwards, the mice were submitted to several behavioral tests, including object location, objection recognition, and spontaneous alternation (independent groups of animals). One-day after the object recognition test, mice were food-deprived overnight, anesthetized, and the blood was collected from the heart to determine plasma cholesterol levels. Mice’s prefrontal cortex and hippocampus were dissected to determine mRNA levels of tight junction proteins and pro-inflammatory molecules. In the sequence of object location test, four or five animals in each group were perfused transcardially, and an immunofluorescence staining was performed in order to analyze the microvessels using tomato lectin, as well as glial fibrillary acidic protein (GFAP) and AQP-4 immunoreactivity. Finally, in another experimental set, immediately after the spontaneous alternation test, the mice were submitted to sodium fluorescein assay to evaluate the BBB permeability. It is worth mentioning that the animals continued to be fed with a high cholesterol diet or regular diet until euthanasia. Figure 1A presents a scheme of the study design and the experimental cohorts’ organization.
Fig. 1
Experimental protocol, physiological parameters, and cognitive function of C57BL/6 wild-type and LDLr–/– mice after normal or high cholesterol diets. A) Experimental design: The C57BL/6 and LDLr–/– mice were exposed for thirty days to normal or high cholesterol diet (1.25% of cholesterol). In the end, the animals were submitted to behavioral tasks and sodium fluorescein assay. The sodium fluorescein assay was used to analyze the blood-brain barrier permeability. Afterwards, the mice’s brains were prepared for gene expression and immunofluorescence analysis. Also, plasma total cholesterol levels and body weight were evaluated. B) Cholesterol levels. C) Body mass. D) Locomotor activity (open field). E) A significant correlation between cholesterol levels and the total number of crossings in the open field test. F) Recognition memory (object recognition test). G) A significant correlation between cholesterol levels and discriminatory score (Pearson’s correlations). H) Spatial memory (object location test). I) Working memory (spontaneous alternation test). Data are expressed as the mean±SEM (n = 4–5 for cholesterol analysis and n = 8 for weight gain and behavioral analysis). *p < 0.05, **p < 0.01 and ***p < 0.001 compared with C57BL/6 mice fed with a normal diet, ##p < 0.01 and ###p < 0.001 compared with C57BL/6 mice fed with a high cholesterol diet, and &p < 0.05 and &&&p < 0.001 compared with LDLr–/– mice fed with a normal diet (Two-way ANOVA followed by Duncan post-hoc test). +p < 0.05 versus chance levels (50% of a new object or displaced object investigation in test trial and spontaneous alternations, respectively; one-sample t-tests).
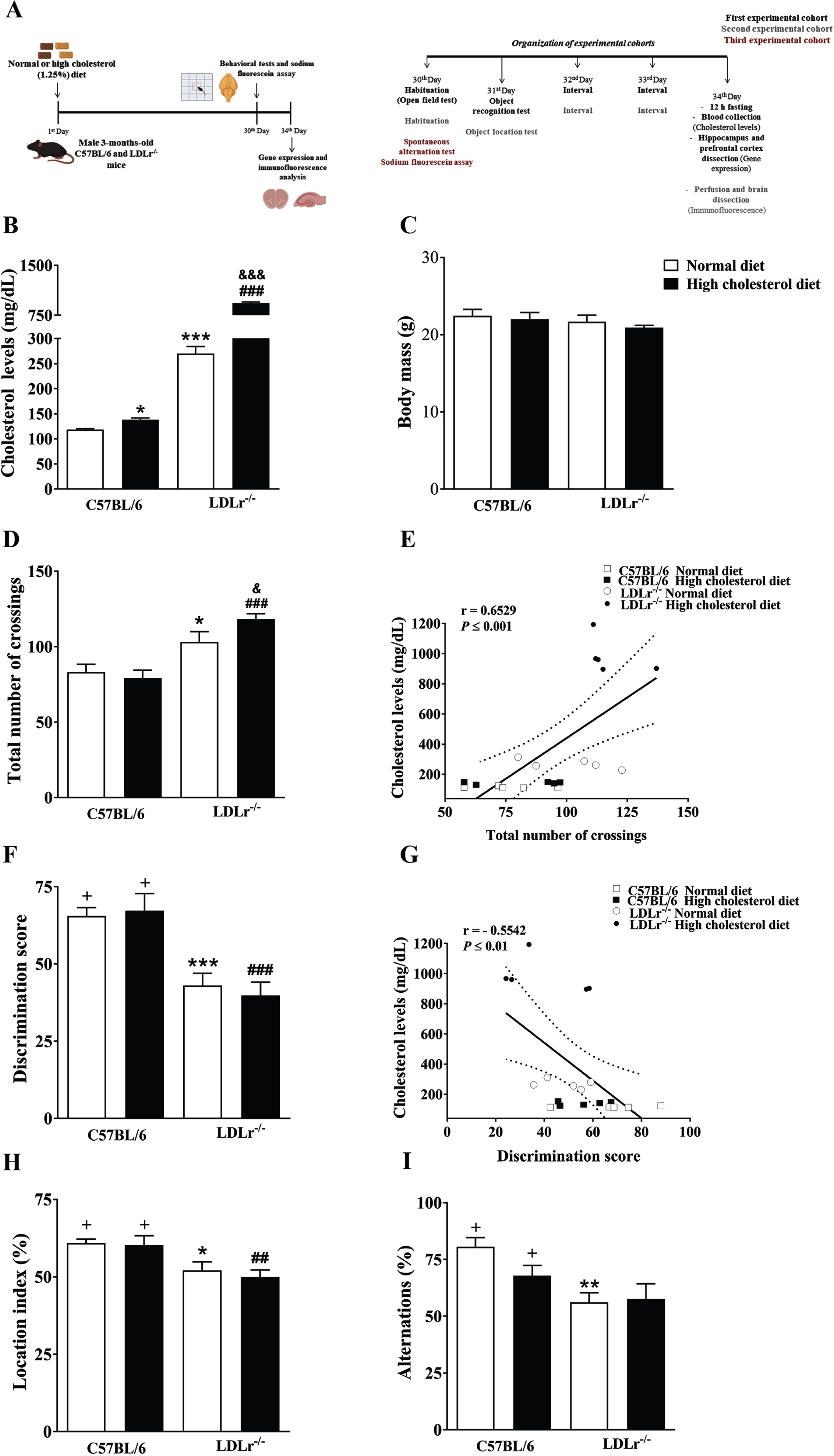
Plasmatic total cholesterol levels and body mass
Total cholesterol levels were measured in the plasma using the commercially available enzymatic kit (Gold Analisa Diagnóstica Ltda, Minas Gerais, Brazil) and following the manufacturer’s instructions. The results were expressed in mg/dL. The plasma cholesterol was analyzed in 20 animals (n = 4–5). At the end of the feeding protocol, the body weight of the animals was measured.
Behavioral tests
Open field
The spontaneous locomotor activity of mice was evaluated in the open-field arena. The apparatus, made of transparent PVC, had a white floor of 50 cm×50 cm and transparent walls (divided by black lines into squares of 10 cm×10 cm), 40 cm high. The experiments were conducted in a dimly lit (7 lx) and sound-isolated room. Each mouse was placed in the center of the open field, and the number of squares crossed was registered for 5 min.
Object location test
To assess the spatial reference memory of mice, we used the object location task, which is based on the spontaneous tendency of rodents, previously exposed to two identical objects, to later explore one of the objects (replaced in a new location) for a longer time than they explore the nondisplaced object, providing a qualitative index of learning [17]. The n per group in this test was eight. The experimental apparatus used was an open-field box (50 cm wide×50 cm deep×40 cm high) made of transparent polyvinyl chloride (PVC), placed in a dimly lit (7 lx) and sound-isolated room. Identical plastic rectangles (4 cm high×4.5 cm wide) were used as objects. The protocol was based on that previously described [18]. The mice were placed in the center of the apparatus with two identical objects for 5 min. The objects were placed 7 cm away from the walls of the open field. Exploration of the objects was timed by a stopwatch when mice sniffed, whisked, or looked at the objects from no more than 1 cm away. After the training phase, the mice were removed from the apparatus for 180 min. After the inter-trial interval, one object was moved to a new location. The time spent exploring the objects in new (novel) and old (familiar) locations was recorded for 5 min. All locations for the objects were counterbalanced among the groups. After each trial, the experimental apparatus was cleaned only with dry paper, thus ensuring that it was saturated with the animals’ smell. In order to analyze the cognitive performance, a location index was calculated as previously described by Murai et al. [17]: (Tnovel × 100)/(Tnovel + Tfamiliar) , where Tnovel is the time spent exploring the displaced object and Tfamiliar is the time spent exploring the nondisplaced object [17].
Object recognition test
The object recognition task was also used to assess hippocampal-dependent memory [19]. During the training session, the animal was exposed to two identical objects for 5 min. The animals were returned to their home cages immediately after training. One hour after the training, the animals were re-introduced into the open field that contained one novel object and one previously explored object. The objects were of similar exploratory level/physical complexity and similar size. A discriminatory index (total time spent with the new object/total time of objects exploration) was used to measure recognition memory [20]. The n per group in this test was eight.
Spontaneous alternation test
The delayed spontaneous alternation test in the T maze was used as a qualitative index of working memory in mice [10]. The experimental apparatus was made of transparent black Plexiglass with a central arm and two lateral arms positioned at a 90° angle to the central arm. The central arm was separated from the other two by a sliding door. Sliding doors were placed at the entrance of each lateral arm. In the first trial, the ‘forced-choice trial,’ either the left or right goal arm, is blocked by lowering the guillotine door. After the mouse has been released from the start arm, it will negotiate the maze, eventually enter the open goal arm, and return to the start position. The animal will be confined for 5 s by lowering the guillotine door of the start arm. After that, during 14 ‘free-choice’ trials, the mouse can choose freely between the left and right goal arm. After opening the guillotine door of the start arm, the animal is free to choose between both goal arms (all guillotine doors open). When the mouse has entered one goal arm, the other goal arm is closed. The mouse eventually returns to the start arm, and the next free-choice trial starts after 5 s confinement in the start arm. A session is terminated, and the animal is removed from the maze immediately after the 14 free-choice trials have been performed, or 30 min have elapsed, whatever event occurs first. During the session, the animals are never handled by the experimenter. In order to isolate the cognitive component, care was taken to diminish the odor cues present by cleaning the maze with a damp sponge before each new animal started its session, as these cues can influence the alternation rate [10, 21]. In this behavioral test, we used a total of 32 mice (n = 8).
Sodium fluorescein assay
The BBB permeability to low molecular weight dye, sodium fluorescein, was evaluated in the prefrontal cortex and hippocampus of mice. Each mouse was anesthetized with isoflurane and injected via penile vein by sodium fluorescein (4%, 4 mL/kg). After 30 min of the administration, animals were anesthetized under ketamine and xylazine mixture (75 and 10 mg/kg, respectively, i.p.) and perfused through the left cardiac ventricle with 0.9% saline solution. Followed the perfusion, brain structures were dissected and processed for the sodium fluorescein concentration analyses [22, 23]. Briefly, the prefrontal cortex and hippocampus were diluted in trichloroacetic acid (TCA) 7.5% (1:7 and 1:6, respectively), homogenized and centrifuged at 10,000×g for 10 min. Supernatants were diluted with 1:2.5 volume of 1 M phosphate buffer (TFK; pH 7.0) before spectrophotometric determination of sodium fluorescein (485 nm excitation/538 nm emission) fluorescence. Data are calculated using a calibration curve of sodium fluorescein and reported as ng/mg wet weight. We performed the sodium fluorescein assay in 28 mice (n = 6–7).
Immunofluorescence
Approximately twenty mice (n = 4–5) were deeply anesthetized under ketamine and xylazine mixture (75 and 10 mg/kg, respectively, i.p.) and were perfused through the left cardiac ventricle with 0.9% saline solution, followed by 4% paraformaldehyde in 0.1 M phosphate-buffered saline (PBS), pH 7.4. After perfusion, the brains were removed, post-fixed in the same fixative solution for 24 h at room temperature, and cryoprotected by immersion in a 30% sucrose solution in PBS at 4°C. The brains were then frozen by immersion in cooled isopentane and stored in a freezer at –80°C for later analyses. Serial coronal sections (40μm) of the prefrontal cortex and hippocampi were obtained with a cryostat (Leica) at –20°C. The free-floating sections were first blocked using 5% horse serum (HS) diluted in PBS containing 2% Triton X-100 (PBS-Tx) for 2 h at room temperature and incubated overnight at 4°C with the following primary antibodies: mouse anti-GFAP (Sigma, 1:400) and anti-AQP-4 (Santa Cruz Biotechnology, AQP4, 1:100) from goat in 1% HS diluted in 0.5% PBS-Tx. After three washes in PBS, tissue sections were incubated with anti-mouse Alexa 488 (Invitrogen, 1:400) and anti-goat Alexa 568 (Invitrogen, 1:400) in 1% HS diluted in 0.5% PBS-Tx for 2 h at room temperature. Hereafter, the sections were washed three times in PBS and mounted on slides with CC/Mount (Sigma), and covered with coverslips. For microvessels staining, the free-floating sections were first incubated with 0.1 M tris-buffered saline (TBS) containing 1% Triton X-100 for 20 min at room temperature. Next, the sections were incubated overnight at 4°C with lectin from Lycopersicon esculentum (tomato lectin; Sigma), 5μg/mL. After three washes in TBS, tissue sections were incubated with Streptavidin Alexa 568 conjugate (Invitrogen, 1:500) in TBS for 1.5 h at room temperature. Hereafter, the sections were washed three times in TBS and mounted on slides with CC/Mount (Sigma) and covered with coverslips. Finally, images from 8 serial sections of each mouse prefrontal cortex (at the level of Bregma 3.14 to 1.98 mm) and hippocampi (at the level of Bregma –1.28 to –2.75 mm) were obtained with a laser-scanning confocal Leica DMI6000 B Microscope. Accurately, approximately 40–50 confocal sections of 0.8μm thickness were taken parallel to the coverslip (xy sections). All lighting conditions and magnifications were kept constant with an ×10 objective. After that, we performed a Z-stack reconstruction. Image reconstruction and fluorescence quantification of the total prefrontal cortex and of the sum of the hippocampi CA1, CA3, and dentate gyrus regions were performed using ImageJ software, mostly as described [10, 24].
Isolation of RNA from brain tissues and cDNA synthesis for real-time quantitative PCR
Animals (n = 5–7 per group, a total of 28 animals) were anesthetized with the preparation of xylazine 10 mg/kg and ketamine 75 mg/kg, i.p., euthanized, and the prefrontal cortex and hippocampus were dissected. Brain regions were flash-frozen in liquid nitrogen and kept at –80°C until used. Total RNA was extracted using SV Total RNA Isolation Kit (Promega, USA), and 0.4μg of each sample was reverse transcribed to cDNA using TaqMan RT reagents. The reaction mixture was incubated 25°C for 10 min, 48°C for 1 h, and 95°C for 5 min. Quantitative real-time PCR, using the ABI 7900HT cycler and SYBR Green Master Mix reagent, was performed according to the manufacturer’s protocol (Applied Biosystems). 10–100 ng of cDNA were amplified in a total volume of 10μL. PCR reactions were conducted using primer designed with the Primer Express version 3.0 software (Applied Biosystems, USA). The primers were as follows: for claudin-5 forward 5′- GCTCAGAACAGACTACAGGCACTTT -3′ and reverse 5′-GTGGCCAACTGCGCATAGA -3′; occludin forward 5′-AGCCTCGGTACAGCAGCAAT -3′ and reverse 5′- CCTTCGTGGGAGCCCTTT -3′; interleukin (IL) IL-1β forward 5′- GATGATAACCTGCTGGTGTGTGA -3′ and reverse 5′- GTTGTTCATCTCGGAGCCTGTAG -3′; IL-6 forward 5′- CGCTATGAAGTTCCTCTCTGCAA -3′ and reverse 5′- CACCAGCATCAGTCCCAAGA -3′; NOS-2 forward 5′- GGCAGCCTGTGAGACCTTTG -3′ and reverse 5′- CATTGGAAGTGAAGCGTTTCG -3′ and for ß-actin forward 5′- AAATCGTGCGTGACATCAAAGA -3′ and reverse 5′- GCCATCTCCTGCTCGAAGTC -3′. Primers were used in a final concentration of 0.5μM. The reaction conditions were 50°C for 2 min, then 95°C for 10 min, followed by 40 cycles of 95°C for 10 s and 60°C for 1 min. The data were analyzed using Sequence Detection Systems (SDS) version 2.4 software (Applied Biosystems). A dissociation step was added for SYBR Green runs. For each sample, gene expression was quantified using a standard curve and normalized against the expression of the ß-actin gene [11].
Statistical analyses
All data were expressed as the mean±SEM. Statistical evaluation was carried out using the two-way analysis of variance (ANOVA) with genotype and diet as independent variables. Following significant ANOVAs, multiple comparisons were performed using Duncan’s post hoc test, without multiple comparisons adjustments. The Shapiro-Wilk normality test was used, and data were normalized using square root when they did not follow a normal distribution. The object recognition, object location, and spontaneous alternation tasks were also analyzed by one-sample t-tests to determine whether the discrimination score, location index, and percentage of alternations were different from minus 50% (i.e., chance level). Pearson’s correlations (r, P) were calculated for associations between plasma cholesterol total content and the total number of crossings or discrimination score, as well as between cholesterol levels and sodium fluorescein permeability in the hippocampus, and content of sodium fluorescein in the hippocampus and discrimination score (the plot includes values from all the animals’ groups). Outliers were defined, and then, excluded after analysis. The accepted level of significance for the tests was p < 0.05. All tests were performed using the Statistic software package (Stat-Soft Inc., Tulsa, OK, USA).
RESULTS
Plasma cholesterol levels and body weight in LDLr–/– and wild-type mice after high cholesterol diet
Like other research groups and we demonstrated previously, LDLr–/– mice present a two to three-fold increase in plasma cholesterol when maintained on a normal diet (standard rodent diet) [9, 13, 25]. When submitted a high cholesterol diet for 30 days, LDLr–/– mice presented with an increase around ten-fold in their total cholesterol levels compared with wild-type C57BL/6 mice. It is worth mentioning that the exposure to high cholesterol diet in wild-type mice caused a 16% increase in the plasma cholesterol levels (moderate hypercholesterolemia) when compared with wild-type mice fed with a normal diet. The two-way ANOVA indicated significant effect for genotype by diet interaction on total cholesterol levels [F(1,15) = 582.30, p < 0.001] (Fig. 1B). About body weight, the two-way ANOVA analyses revealed no significant effect for genotype or diet (Fig. 1C). Therefore, at the end of treatments, the weight did not distinguish between the experimental groups.
Memory loss and increased locomotor activity in hypercholesterolemic mice
Previous work from our laboratory and others showed that LDLr–/– mice present higher locomotor activity than normal matched-mice [12, 26]. Here, we investigated the effect of high cholesterol diet consumption on the locomotor activity of wild-type and LDLr–/– mice, using the open-field arena. Two-way ANOVA indicated a significant effect for genotype on locomotor activity [F(1, 28) = 30.25, p < 0.001]. Subsequent post-hoc comparisons revealed that LDLr–/– mice performed a higher number of crossings in the arena than control mice. When these hypercholesterolemic mice were fed with a high cholesterol diet, the number of total crossing was even higher (Fig. 1D). Also, we observed that the levels of plasma cholesterol were positively correlated with the total number of crossings [R = 0.6129, p < 0.01] (Fig. 1E).
Next, we evaluated a hippocampal-dependent memory (recognition memory) through the object recognition test. Two-way ANOVA revealed a significant effect for genotype on recognition memory [F(1, 28) = 34.90, p < 0.001]. Subsequent post-hoc comparisons demonstrated that LDLr–/– mice, regardless of the diet, presented with memory impairment. Unlike the wild-type mice, LDLr–/– mice did not show any preference toward the novel object, presenting a discrimination score similar to the random chance (Fig. 1F). Furthermore, we found that levels of blood total cholesterol were negatively correlated with the discrimination score [R = –0.5542, p < 0.01] (Fig. 1G).
We also assessed the spatial memory of mice using object location task. Two-way ANOVA pointed a significant effect for genotype on spatial memory [F(1, 28) = 15.44, p < 0.001]. Following Duncan’s test analysis showed that LDLr–/– mice exhibited spatial memory deficits, independent of the diet. Once again, our data showed that the LDLr–/– mice presented with impaired memory regardless of the diet, while C57BL/6 wild-type did not display cognitive deficits in the object location task after the exposure to high cholesterol diet (Fig. 1H).
Moreover, the T-maze was used to assess spontaneous alternation and working memory. Two-way ANOVA indicated a significant effect for genotype on working memory [F(1, 28) = 12.03, p < 0.01]. Subsequent Duncan’s post-hoc test pointed out that LDLr–/– mice fed with a normal or a high cholesterol diet presented with working memory decline. Only wild-type mice, regardless of the diet, presented with a significantly higher percentage of alternations than chance performance. In contrast, both LDLr–/– mice groups fed with standard diet or hypercholesterolemic diet displayed a spontaneous alternation index similar to the chance level (Fig. 1I).
High cholesterol diet exposure caused the loss of BBB integrity in mice
The two-way ANOVA indicated a significant effect for genotype [F(1,23) = 10.96, p < 0.01] and for diet [F(1,23) = 8.18, p < 0.01] on sodium fluorescein permeability in the hippocampus of mice. The subsequent post-hoc comparison revealed that the moderate hypercholesterolemia, in the control mice, was already associated with high permeability to sodium fluorescein in the hippocampus. Furthermore, the LDLr–/– mice per se presented with a significant increase in BBB permeability. When fed with a high cholesterol diet, LDLr–/– mice had their BBB permeability to sodium fluorescein further increased within the hippocampus (Fig. 2A).
With regard to prefrontal cortex, the two-way ANOVA revealed a significant effect for genotype [F(1,24) = 7.99, p < 0.01] and for diet [F(1, 24) = 11.08, p < 0.01] on fluorescein permeability. Subsequent post-hoc comparisons revealed increased sodium fluorescein permeability in the prefrontal cortex of wild-type mice exposed to a high cholesterol diet. The LDLr–/– mice fed with a standard diet presented with a slight increase (p = 0.09) in sodium fluorescein permeability in this brain structure. Importantly, when exposed to a high cholesterol diet, the BBB permeability of LDLr–/– mice was higher than those fed with a normal diet, or even than wild-type mice exposed also to a high cholesterol diet (Fig. 2B).
Fig. 2
Effects of high cholesterol diet on blood-brain barrier (BBB) integrity of C57BL/6 wild-type and LDLr–/– mice. BBB permeability to sodium fluorescein in the (A) hippocampus and (B) prefrontal cortex of wild-type and LDLr–/– mice fed with normal or high cholesterol diet (n = 6–7). C) A significant correlation between cholesterol levels and permeability to sodium fluorescein in the hippocampus. D) A Significant correlation between BBB permeability to sodium fluorescein in the hippocampus and discriminatory score (Pearson’s correlations). Representative images of aquaporin-4 (AQP-4) content immunofluorescence analysis in the (E) hippocampus and (F) prefrontal cortex of the animals. The serial stack of images of AQP-4 (red) immunofluorescence staining were obtained with a Leica DMI6000 B confocal microscope. Scale bars, 150μm. Quantitation of AQP-4 immunoreactivity in the (G) hippocampus and (H) prefrontal cortex (n = 4–5). Gene expression of tight junction’s protein, claudin-5 and occludin, in mice’s (I and K, respectively) hippocampus and (J and L, respectively) prefrontal cortex (n = 5). Data are expressed as the mean±SEM. *p < 0.05, **p < 0.01, and ***p < 0.001 compared with C57BL/6 mice fed with a normal diet, #p < 0.05 and ##p < 0.01 compared with C57BL/6 mice fed with a high cholesterol diet, and &p < 0.05 and &&p < 0.01 compared with LDLr–/– mice fed with a normal diet (Two-way ANOVA followed by Duncan post-hoc test).
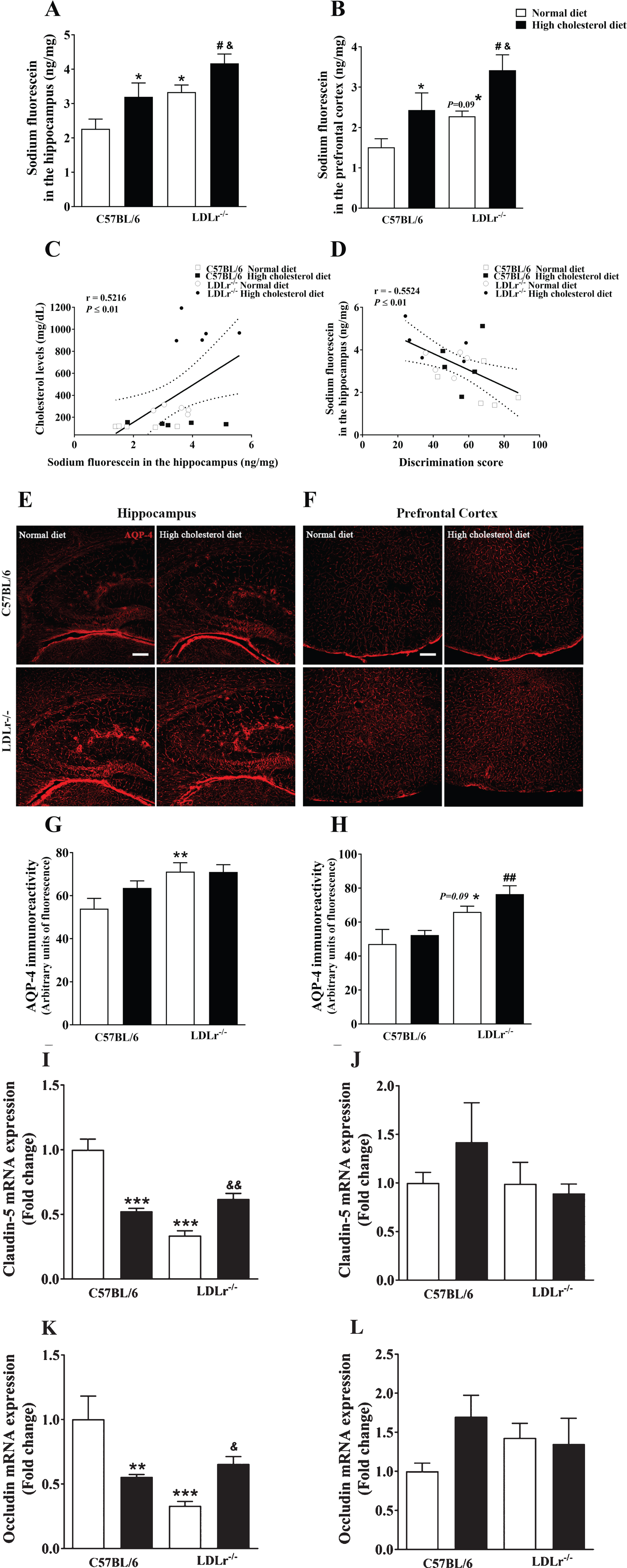
Importantly, we observed a positive correlation between plasma cholesterol levels and BBB permeability to sodium fluorescein in the hippocampus of animals [R = 0.5216, p < 0.01] (Fig. 2C). Also, the BBB permeability in the hippocampus of mice was negatively correlated with the discrimination score, i.e., memory performance, of the mice [R = –0.5524, p < 0.01] (Fig. 2D).
Besides, AQP-4, a bidirectional water channel primarily expressed in perivascular astrocytic foot processes, was also used as a putative biomarker of BBB permeability [10] (Fig. 2E, F). The two-way ANOVA indicated a significant effect for genotype on AQP-4 immunoreactivity in the hippocampus [F(1, 16) = 9.74, p < 0.01] and prefrontal cortex [F(1,15) = 19.95, p < 0.01]. Duncan’s post-hoc test indicated that AQP-4 content in the hippocampus of LDLr–/–mice increased independently of the diet (Fig. 2G). Moreover, subsequent post-hoc comparisons revealed a lightly increase (p = 0.09) in the immunoreactivity of AQP-4 in the prefrontal cortex of LDLr–/– mice. The LDLr–/– mice exposed to hypercholesterolemic diet displayed enhanced immunocontent of AQP-4 in the prefrontal cortex when compared with control mice also exposed to this diet (Fig. 2H).
The BBB proper composes of endothelial cells of the cerebral microvasculature, which are interconnected by tight junctions that, in turn, form a physical barrier restricting paracellular flux [27]. Thus, we evaluated the gene expression of claudin-5 and occludin, which form the paracellular tight junctions. The two-way ANOVA indicated a significant effect for genotype and diet interaction on claudin-5 [F(1, 16) = 54.69, p < 0.001] and occludin [F(1, 16) = 16.15, p < 0.001] mRNA levels in the hippocampus. The exposition to the high cholesterol diet caused a decrease in the levels of RNAm of tight junction’s proteins (claudin-5 and occludin) in the hippocampus of C57BL/6 wild-type mice. Additionally, the LDLr–/– mice presented with decreased gene expression of claudin-5 and occludin in the hippocampus. Notably, when LDLr–/– mice were fed with a high cholesterol diet, tight junction’s proteins RNAm levels were higher than LDLr–/– mice feeding normal diet, but these levels remained different from those of control animals exposed to a normal diet (Fig. 2I and K, respectively). By contrast, in the prefrontal cortex, the two-way ANOVA analyses indicated no significant effect for genotype or diet in claudin-5 and occludin gene expression (Fig. 2J and L, respectively).
High cholesterol diet modifies the brain microvessels content in LDLr–/– mice
The microvessels endothelial cells were labeled using tomato lectin from Lycopersicon esculentum (Fig. 3A, B). The two-way ANOVA indicated a significant effect for genotype [F(1, 15) = 17.06, p < 0.001] on lectin-positive cells in the hippocampus of mice. Subsequent post-hoc analysis revealed that LDLr–/– mice, regardless of the diet, presented with increased labeling of tomato lectin-positive areas compared with wild-type mice fed with a regular diet. However, we did not observe differences in the hippocampal content of lectin-positive cells between LDLr–/– feeding high cholesterol diet and wild-type fed with a high cholesterol diet (Fig. 3A, C). On the other hand, in the prefrontal cortex, the two-way ANOVA analyses indicated no significant effect for genotype or diet in lectin-positive cells (Fig. 3B, D).
Fig. 3
Brain microvessels content in C57BL/6 wild-type and LDLr–/– mice after a high cholesterol diet. The series of images labeled by tomato lectin (red) were obtained with a Leica DMI6000 B confocal microscope. Tomato lectin staining representative images of (A) hippocampi (zoom of CA3 subfield) and (B) prefrontal cortex of mice. Scale bars = 150μm and 40μm (zoom). Quantitation of tomato lectin-positive staining in the (C) hippocampus and (D) prefrontal cortex (n = 4–5). Data are expressed as the mean±SEM. **p < 0.01 compared with C57BL/6 mice fed with a normal diet (Two-way ANOVA followed by Duncan post-hoc test).
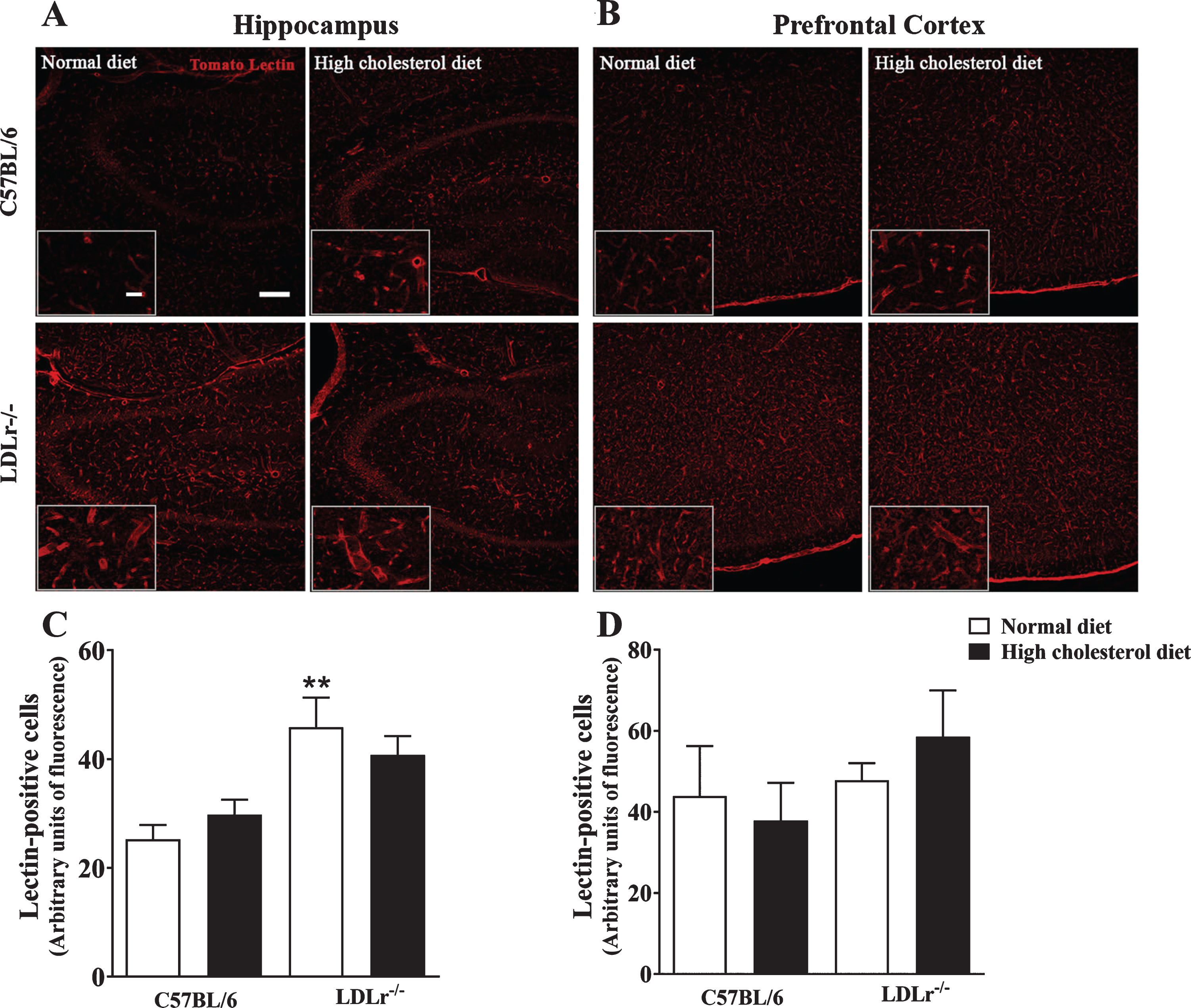
High cholesterol diet stimulated neuroinflammation in mice’s brain
Increased BBB permeability leads to the migration of monocytes across the barrier, as well as the infusion of other pro-inflammatory cytokines resulting in neuroinflammation [28]. Here, we assessed the immune content of GFAP, used as a marker of astrogliosis (Fig. 4A–D). The two-way ANOVA analyses indicated a significant effect for genotype on GFAP immunoreactivity [F(1, 16) = 12.44, p < 0.01] in the hippocampus of mice. Wild-type mice fed with a high cholesterol diet presented with increased GFAP immunoreactivity in the hippocampus (p = 0.055). In agreement with our previous work [10], LDLr–/– mice, regardless of the diet, had high GFAP immune content (Fig. 4A, B, D). As shown in Fig. 4B, LDLr–/– mice hippocampus displayed pronounced astrogliosis, an overlap of astrocyte processes, and disrupted individual astrocyte domains. Finally, we did not find any difference in GFAP content in the mice’s prefrontal cortex (Fig. 4C). It is worth highlight that as we found few astrocytes in the prefrontal cortex, quantification was not performed.
Fig. 4
Neuroinflammation in C57BL/6 wild-type and LDLr–/– mice after a high cholesterol diet. The series of images of glial fibrillary acidic protein (GFAP) (green) immunofluorescence staining were obtained with a Leica DMI6000 B confocal microscope. Representative images of GFAP immunoreactivity of mice’s (A and B) hippocampus and (C) prefrontal cortex. Scale bars = (E and G) 150μm, (F) 50μm and, (B) 20μm (zoom of CA3 subfield). Quantitation of GFAP-positive staining in the hippocampus is presented in panel D (n = 5). Gene expression of proteins related to the brain inflammatory process, interleukin (IL) IL-1β, IL-6, and NOS-2 in the (E, F, and G, respectively) hippocampus and (H, I, and J, respectively) prefrontal cortex (n = 6) of the animals. Data are expressed as the mean±SEM. *p < 0.05 and **p < 0.01 compared with C57BL/6 mice fed with a normal diet, #p < 0.05 and ###p < 0.001 compared with C57BL/6 mice fed with a high cholesterol diet, and &p < 0.05 compared with LDLr–/– mice fed with a normal diet (Two-way ANOVA followed by Duncan post-hoc test).
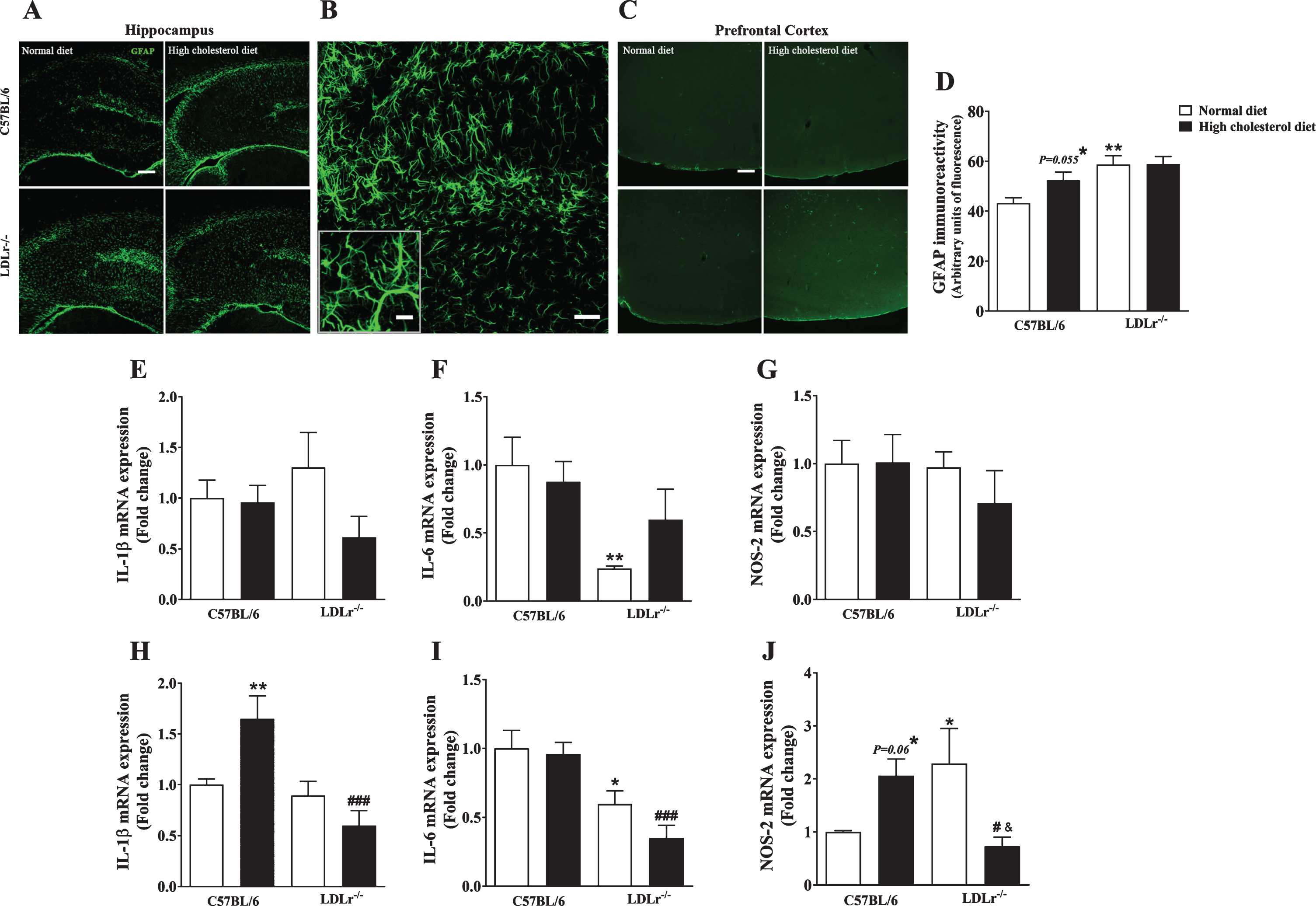
Taking into account that astrogliosis is associated with the production of pro-inflammatory cytokines (such as IL-1β and IL-6) and reactive species (e.g., nitric oxide), we investigated the gene expression of proteins related to inflammation [29]. The two-way ANOVA revealed a significant effect for genotype and diet interaction on IL-1β gene expression in the prefrontal cortex of mice [F(1, 20) = 9.35, p < 0.01]. Duncan’s test pointed out that a high cholesterol diet caused an increase in the levels of IL-1β mRNA in the prefrontal cortex of wild-type mice, which not occurred in LDLr–/– mice (Fig. 4H). Concerning the hippocampus, no differences were observed between experimental groups (Fig. 4E).
The two-way ANOVA analysis also indicated a significant effect for genotype on IL-6 mRNA levels in the hippocampus [F(1, 20) = 9.44, p < 0.01] and prefrontal cortex [F(1, 20) = 24.36, p < 0.001]. Subsequent post-hoc evaluation demonstrated that LDLr–/– per se presented with lower levels of IL-6 mRNA levels in the hippocampus (Fig. 4F) and prefrontal cortex (Fig. 4I).
Additionally, we measured the gene expression of NOS-2, an inducible form of nitric oxide synthase. Importantly, the regulation of NOS-2 is implicated in neuroinflammation mediated by glial cells, mainly astrocytes. In the brain, NOS-2 and cytokines (such as IL-1β) are activated by the NF-κB pathway [30]. The two-way ANOVA indicated a significant effect for genotype and diet interaction on NO-2 gene expression in the mice’s prefrontal cortex [F(1, 20) = 12.08, p < 0.01]. Subsequent post-hoc evaluation (Duncan’s test) showed that wild-type exposed to a high cholesterol diet displayed a slight increase in the levels of NOS-2 mRNA in the prefrontal cortex (p = 0.06) (Fig. 4J). The LDLr–/– mice fed with a standard diet presented with increased gene expression of NOS-2 in the prefrontal cortex. Notably, when LDLr–/– were exposed to a hypercholesterolemic diet, the levels of NOS-2 mRNA decreased (Fig. 4J). No significant differences were found regarding the NOS-2 mRNA levels in the hippocampus of mice (Fig. 4G).
DISCUSSION
The natural history of FH patients involves the premature development of cardiovascular diseases associated with atherosclerosis [31]. In the last decade, it has been discovered that FH is also related to the emergence of cognitive impairment in adulthood [6, 7]. In this context, we previously reported that three-month-old LDLr–/– mice already displayed learning and memory deficits that seemed to be triggered by BBB dysfunction and neuroinflammation within the hippocampus [9, 10]. In this study, we aimed to investigate the impact of exposure to a high cholesterol diet for thirty days on cognitive function and BBB integrity in wild-type C57BL/6 and LDLr–/– mice. We also searched for the mechanisms underpinning cognitive deficits and BBB disruption in FH.
As expected [32], LDLr–/– mice fed with a regular diet presented with a two- to three-fold increase in total cholesterol levels in the plasma. It is worth mentioning that a high cholesterol diet induced an almost tenfold elevation in the plasma cholesterol levels of LDLr–/– mice. In contrast, wild-type mice manifested only a moderate increase in the blood cholesterol levels when fed with a hypercholesterolemic diet. Another critical point is that a high cholesterol diet did not cause any changes in the body weight of both mouse strains; although the diet used herein contained 20% of fat, it was not obesogenic. This diet is much more related to changing cholesterol levels due to the high concentration of cholesterol when compared with other experimental diets. Experimental studies in which C57BL/6 wild-type and LDLr–/– mice were exposed to Western and high-fat feeds for more extended periods observed changes in body weight [15, 33, 34]. Western diets contain a higher concentration of fat and a lower concentration of cholesterol than the diet used in our study, while the majority of high-fat diets contain more than 40% of fat without the addition of cholesterol.
In this study, the moderate hypercholesterolemia in wild-type mice was associated with BBB leakage of sodium fluorescein into the hippocampus and prefrontal cortex. Our data accords with a study by Ullrich et al. [35]. They observed an enhanced immunocontent of IgG in the cortex of rats fed with a high cholesterol diet. Furthermore, C57BL/6 wild-type mice exposed to a Western diet also presented with an increased BBB transport observed in MRI analysis [15].
Our data showed that BBB dysfunction was also observed in the brain structures of LDLr–/– mice fed with a normal diet. In contrast, Rutkowsky and collaborators [15] observed increased BBB transport in LDLr–/– mice only when they were exposed to a Western diet. Sodium fluorescein is a small molecule that can be detected in brain areas at extremely low concentrations. The spectrophotometric measurement of sodium fluorescein injected via peripheral infusion is an overly sensitive method of evaluating BBB permeability. It has been suggested that an increased transport of sodium fluorescein across the BBB appears to be the most sensitive indicator of the increased permeability of the BBB [36]. In fact, the peripheral injection of dyes and analysis of the leakage in brain tissue has been used extensively to evaluate BBB function [36, 37]. Notably, when exposed to a high cholesterol diet, the BBB of LDLr–/– mice leaked sodium fluorescein more severely. Additionally, the increased susceptibility of LDLr–/– mice to manifest BBB disruption was herein demonstrated by increased staining of AQP-4 in the animals’ hippocampi. AQP-4, which is expressed in the feet of astrocytes, is the main water channel in the brain. Increased AQP-4 expression is related to brain edema and consequently, neurological disorders pathogenesis [38, 39]. However, the contribution of AQP-4 depends on the disease stage. Besides water balance regulation, AQP-4 has a range of functions in the CNS, including a role in brain inflammation [39]. It is worth noting that BBB disruption and increased permeability in the hippocampi and prefrontal cortices of LDLr–/– mice occurred as early as three months of age, and it seemed to be more intense than the BBB disturbance visualized in the brains of wild-type mice fed with a high cholesterol diet. We observed a positive correlation between plasma cholesterol levels and BBB permeability to sodium fluorescein in the mice’s hippocampi.
The BBB is extraordinarily complex and dynamic barrier. It is composed of a network of brain endothelial cells from capillaries, which present protective features, and perivascular cells (including astrocytes) that maintain those features. For example, brain vascular endothelium presents tight junctions between endothelial cells. The components of these tight junctions, e.g., the membrane proteins claudin-5 and occludin, are fundamental to BBB integrity and function [40–42]. Indeed, claudin-5 and occludin are the dominant tight junctions proteins in brain areas such as the hippocampus [43, 44], and a decreased gene expression of these proteins is closely related to BBB disruption [45, 46]. We observed that leakage in the BBB of LDLr–/– mice and wild-type mice fed with a high cholesterol diet was accompanied by a decreased hippocampal gene expression of claudin-5 and occludin. Notably, high-cholesterol-fed LDLr–/– mice presented higher mRNA levels of tight junction proteins than those observed in LDLr–/– mice fed with a normal diet. One might suppose that such upregulation in the mRNA levels of tight-junctional proteins is a compensatory mechanism for the greater disruption to the integrity of the endothelial barrier observed in high-cholesterol-fed LDLr–/– mice, in order to repair the compromised BBB. Moreover, we also found an increased microvasculature density, indicated by a larger number of lectin-positive cells within the hippocampus of LDLr–/– mice, regardless of diet. According to this, Franciosi and colleagues [47] previously showed pathological changes in the brain vessels of six-month-old LDLr–/– mice. Pathological angiogenesis correlated with BBB breakdown was also observed in the hippocampi of rats fed with a high-fat diet for 12 weeks [48]. Nonetheless, as we observed in the findings from the sodium fluorescein assay (a functional test to analyze BBB integrity), the increase in the tight junctions’ mRNA levels was not enough to prevent BBB leakage in the hippocampus. Our results suggest that BBB plasticity is triggered during the evolution of FH, although it was not enough to control leakage or prevent further brain damage.
BBB integrity is critical to neuronal homeostasis and function. The BBB separates the brain from the periphery both physically and metabolically. It makes the brain an “immune privileged” place [49, 50]. Significantly, BBB dysfunction was detected in patients with MCI and dementia [16, 51]. Herein we showed that the BBB dysfunction in high-cholesterol-fed wild-type mice was not associated with memory impairment. It is important to mention some previous studies [13, 14] that used a more prolonged period of exposure to a high cholesterol diet (at least eight weeks), then observed memory impairments in wild-type mice. Moreover, Machado et al. [52] demonstrated that three-month-old C57BL/6 mice exposed to a high cholesterol diet for 30 days did not present with memory damage. However, the authors also observed that the treatment of the mice with a non-effective dose of scopolamine caused cognitive impairment, which pointed to the possibility that prior biochemical brain alterations made them more susceptible to memory dysfunction. In the present study, young adult LDLr–/– mice displayed recognition, spatial, and working memory deficits when submitted to object recognition, object location, and spontaneous alternation tasks, respectively. These findings were in line with our previous work [12, 53]. We also found a correlation between plasma cholesterol levels and cognitive decline, i.e., higher cholesterol levels were related to a lower discriminatory score in the novel object recognition task. We also observed that increased BBB permeability in the hippocampus was negatively correlated with the mice’s memory performance. The BBB disruption seemed to be critically related to hypercholesterolemia-induced cognitive impairment.
Another behavioral alteration displayed by LDLr–/– mice was an increase in locomotor activity, a hyperactive phenotype [26]. Additionally, we observed that the locomotor activity in LDLr–/– mice was even greater when they were given a high cholesterol diet. In fact, we observed a positive correlation between cholesterol levels and the total number of crossings in the open-field arena. We speculated that the increased total number of crossings in the open-field arena found in hypercholesterolemic LDLr–/– mice was due to a lack of spatial habituation [54, 55].
The association between BBB disruption and cognitive impairment that we observed in LDLr–/– mice was not accompanied by any alterations in cerebral Aβ levels, i.e., amyloid pathology. Recently, we showed that memory impairment in three- and fourteen-month-old LDLr–/– mice were linked to an exacerbation of neuronal apoptosis, without changes in Aβ levels, in the prefrontal cortex and hippocampus [11]. Our data accord with the findings regarding from ApoE–/– mice, another hyperlipidemia mouse model. These mice also developed secondary neurodegenerative changes after BBB breakdown in the absence of Aβ pathology [56]. The loss of BBB integrity and consequent neuronal death are possibly the cause of cognitive dysfunction induced by hypercholesterolemia, mainly FH. However, it is worthy of notice that diet-induced hypercholesterolemia might be involved in a modification in Aβ levels. Thirumangalakudi et al. [13] demonstrated that a two-month hypercholesterolemic diet caused an increase in Aβ1–40 levels, but not Aβ1–42, in the cerebral cortex of LDLr–/– mice. It is necessary to mention that these authors did not compare the content of Aβ between wild type and LDLr–/– mice. Moreover, five months’ exposure to a high cholesterol diet (5% cholesterol) in rats led to increased Aβ immunoreactivity in the cortex [35].
The mechanisms that are involved in the connection between BBB disruption and cognitive impairments induced by hypercholesterolemia need to be investigated further. In several diseases, neuroinflammation is known to link increased BBB permeability and brain injury [57]. Many studies have demonstrated that the extravasation of plasma content into the brain through a disrupted BBB has the potential to cause neuroinflammation [58–60]. Also, a leaking BBB induces neuroinflammatory responses both by allowing peripheral inflammatory cells to infiltrate the brain parenchyma and activating glial cells. It is known that neuroinflammation coincides with the vascularization of the brain [61]. Lectin-positive cells observed herein in the hippocampi of hypercholesterolemic mice might connote a possible rearrangement of the hippocampal microvasculature, allowing the infiltration of circulating cells through the brain parenchyma and thereby initiating the process of neuroinflammation. Thus, a vicious cycle is created, because that inflammation compromises the BBB [62]. Microglia and astrocytes activation are the main components of neuroinflammation. As per our previous work [10], we observed intense astrogliosis in the hippocampi of LDLr–/– mice. This is characterized by a reduction in individual astrocyte domains. In this regard, we showed previously that in vitro LDL treatment had a prominent effect on astrocytes morphology [63]. We also reported dysfunctional hippocampal neurogenesis in young adult LDLr–/– mice [63]. Importantly, neuroinflammation associated with astrogliosis can alter the process of neurogenesis [64]. Furthermore, herein, wild-type mice fed with a high cholesterol diet also exhibited a slight but not significant increase in hippocampal astrocyte density.
Reactive astrocytes can release a cascade of pro-inflammatory and neurotoxic factors including inflammatory interleukins and reactive species, all of which can disrupt the BBB, enhance the brain inflammatory cascade, and cause neuronal dysfunction and death [65, 66]. Previously, we showed that LDLr–/– mice presented with an increased level of reactive species in their prefrontal cortices and hippocampi [10]. In the present study, we observed that LDLr–/– mice fed with a regular diet presented an increased gene expression of NOS-2 (inducible NOS) in their prefrontal cortices. The activity of this isoform of NOS is related to cells’ inflammatory response. Importantly, it is known that the production of nitrite oxide, induced by NOS-2, is related to brain damage associated with systemic inflammation [67]. Indeed, the regulation of the inducible NOS isoform, highly implicated in neuroinflammatory processes associated with glial cells, takes place at the transcriptional level [68]. Herein, we also visualized that a compensatory response in LDLr–/– mice exposed to a hypercholesterolemic diet led to a decrease in gene expression of NOS-2 in their prefrontal cortices.
IL-6 also plays an important part in the neuroinflammatory process, but this interleukin presents significant roles in brain function. In physiological conditions, it is involved in neural plasticity, long-term potentiation, and memory [69]. Furthermore, it can exert opposite actions triggering either neuronal survival after injury or producing neuronal degeneration and cell death in neurodegenerative disorders [70]. Although IL-6 is mostly regarded as a pro-inflammatory cytokine, other of its signaling properties can induce anti-inflammatory effects, making it a pleiotropic cytokine [71]. Herein, we observed a significant decrease in IL-6 mRNA levels in the hippocampi and prefrontal cortices of LDLr–/– mice, regardless of diet. Similarly, Rutkowsky and colleagues [15] discovered a reduction in IL-6 gene expression in the brains of LDLr–/– mice. Given the the dual effects of IL-6, it is not possible to conclude whether the observed reduction in hippocampal IL-6 levels of LDLr–/– mice has beneficial or hazardous consequences. Moreover, IL-1β is a cytokine intimately involved in the intensification of acute neuroinflammatory processes [72]. However, we did not observe any alterations in the mRNA levels of IL-1β in the hippocampi or prefrontal cortices of LDLr–/– mice, regardless of diet. While this was the case in Rutkowsky et al.’s study [15], Thirumangalakudi et al. [13] did report an increase in the expression of IL-1β in the hippocampus of LDLr–/– mice. We observed a significant increase in mRNA levels of NOS-2 and IL-1β in the prefrontal cortex of C57BL/6 mice fed with a high cholesterol diet. The increased permeability of the BBB in C57BL/6 wild-type mice fed with a high cholesterol diet appeared to be correlated with an induction of the inflammatory molecules’ response.
Another relevant finding in our study was the greater susceptibility of the hippocampus to the effects of hypercholesterolemia than the prefrontal cortex. We observed that the dysfunction in the BBB (i.e., decreased levels of claudin-5 and occludin mRNA levels), astrogliosis, and increased lectin-positive cells were more evident in the hippocampi of hypercholesterolemic mice, mainly of the LDLr–/– strain. This is significant, because the hippocampus is considered to be one of the most vulnerable brain regions in the early phases of neurodegenerative dementias, including Alzheimer’s disease [73]. Furthermore, it also seems to be more affected by metabolic disorders. For instance, Kanoski et al. [74] demonstrated that a high fat diet intake caused BBB breakdown in the hippocampi of rats, but not in their prefrontal cortices or striata. However, it is essential to mention that we did not discard the impact of hypercholesterolemia in the prefrontal cortex. In the sodium fluorescein assay, we observed an increased BBB leakage in the prefrontal cortex of hypercholesterolemic mice. These data are in agreement with the damage induced by hypercholesterolemia that we already demonstrated in this brain structure [14, 11]. Our previous data showed that Swiss mice exposed to a high cholesterol diet presented increased acetylcholinesterase activity in the prefrontal cortex [14]. Additionally, we recently observed exacerbation of apoptosis in the prefrontal cortex of LDLr–/– mice [11].
In summary, our results point to BBB disruption as a critical event in brain alteration caused by diet-induced hypercholesterolemia. Most importantly, a greater disruption of BBB integrity was observed in three-month-old LDLr–/– mice which, consequently, might have played a primary role in the cognitive dysfunction already observed in young adults with FH (Fig. 5). Finally, neuroinflammation is an important mechanism related to BBB disturbance and cognitive impairment in FH. Our study provides relevant new data about the role of neuroinflammation and BBB dysfunction in the onset of cognitive impairment caused by hypercholesterolemia. However, the study has some limitations with regard to the relationship and causality of these effects. More research is needed to unravel the deep molecular mechanisms underlying the involvement of BBB disruption and neuroinflammation in the development of cognitive impairment caused by hypercholesterolemia.
Fig. 5
An illustrative scheme summarizing the main findings in C57BL/6 and LDLr–/– mice after a high cholesterol diet. The LDLr–/– mice, which already presented increased levels of plasma cholesterol, after a high cholesterol diet exhibited severe hypercholesterolemia. The hypercholesterolemia in LDLr–/– induced leakage of BBB was associated with intense astrogliosis, increased microvessels content, and decreased levels of IL-6, which ultimately caused cognitive impairments. The LDLr–/– mice exposed to high cholesterol diet exhibited a further increase in the leakage to sodium fluorescein in the hippocampus and prefrontal cortex, which was associated with an upregulation in the gene expression of claudin-5 and occludin in the hippocampus. On the other hand, C57BL/6 mice fed with a high cholesterol diet displayed moderate hypercholesterolemia that was also associated with BBB disruption, but not with significant induction of the neuroinflammatory process and, therefore, did not cause alterations on cognitive function.
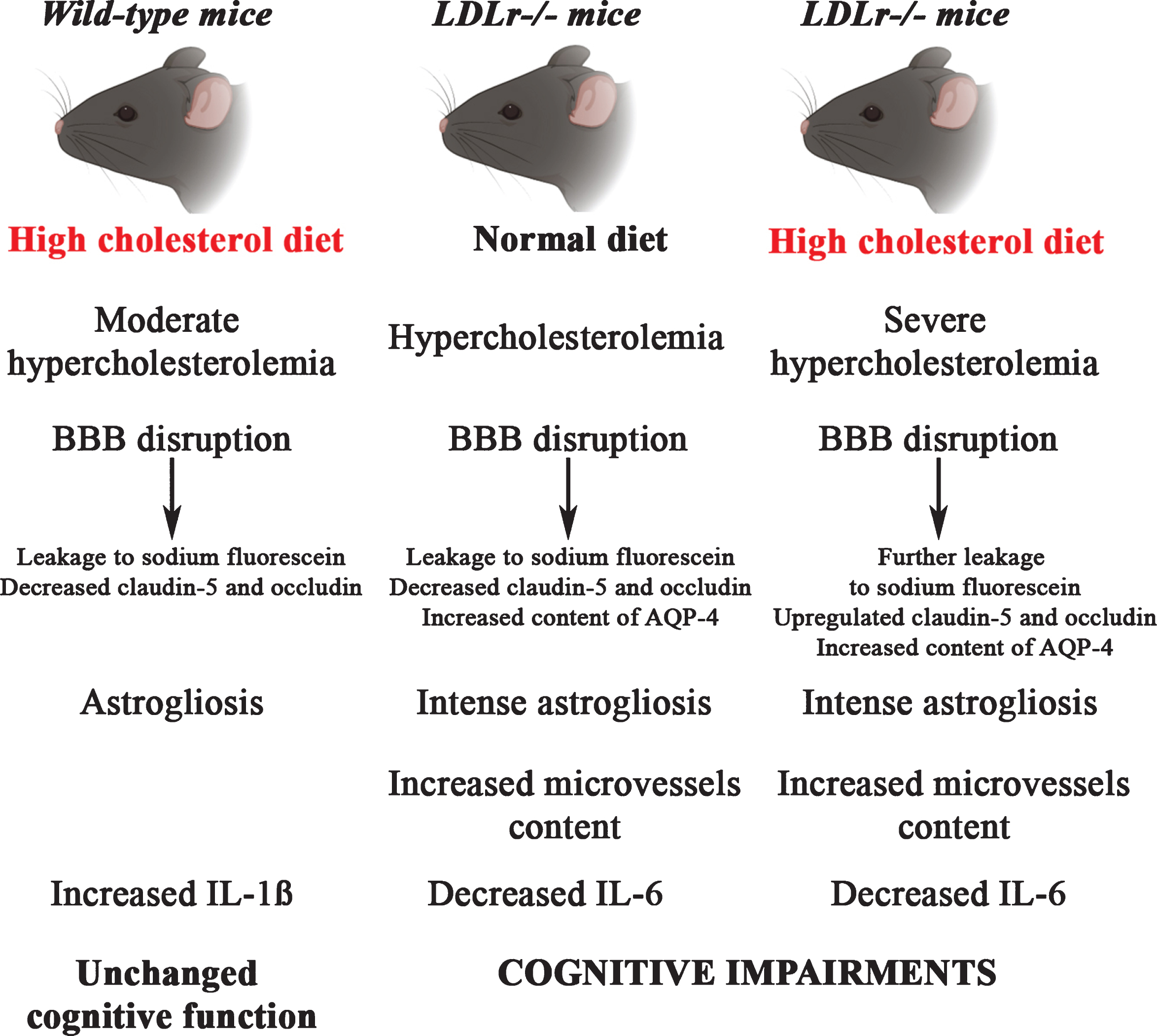
Authors’ disclosures available online (https://www.j-alz.com/manuscript-disclosures/20-0541r2).
ACKNOWLEDGMENTS
This research was supported by the Fundação de Apoio à Pesquisa do Distrito Federal (FAPDF grant 00193-00001324/2019-27), Fundação de Apoio à Pesquisa de Santa Catarina (FAPESC grant 16802017 06/2016), Conselho Nacional de Desenvolvimento Científico e Tecnológico (CNPq grant 424809-2018-4), Brazilian National Institute of Science and Technology on Neuromodulation (485489/2014-1) and in Excitotoxicity and Neuroprotection (465671/2014-4), and Coordenação de Aperfeiçoamento de Pessoal de Nível Superior (CAPES). Additionally, we gratefully acknowledge Prof. Dr. Connie McManus (Institute of Biological Sciences, University of Brasilia, Brazil) for the valid discussions about the statical analysis, which contributed to the quality of our article.
REFERENCES
[1] | Simons K , Ikonen E ((2000) ) How cells handle cholesterol. Science 290: , 1721–1726. |
[2] | Goldstein JL , Brown MS ((2015) ) A century of cholesterol and coronaries: From plaques to genes to statins. Cell 161: , 161–172. |
[3] | Brown MS , Goldstein JL ((1986) ) A receptor-mediated pathway for cholesterol homeostasis. Science 232: , 34–47. |
[4] | Defesche JC , Gidding SS , Harada-Shiba M , Hegele RA , Santos RD , Wierzbicki AS ((2017) ) Familial hypercholesterolaemia. Nat Rev Dis Primers 3: , 17093. |
[5] | Vallejo-Vaz AJ , Ray KK ((2018) ) Epidemiology of familial hypercholesterolaemia: Community and clinical. Atherosclerosis 277: , 289–297. |
[6] | Zambón D , Quintana M , Mata P , Alonso R , Benavent J , Cruz-Sánchez F , Gich J , Pocoví M , Civeira F , Capurro S , Bachman D , Sambamurti K , Nicholas J , Pappolla MA ((2010) ) Higher incidence of mild cognitive impairment in familial hypercholesterolemia. Am J Med 123: , 267–274. |
[7] | Ariza M , Cuenca N , Mauri M , Jurado MA , Garolera M ((2016) ) Neuropsychological performance of young familial hypercholesterolemia patients. Eur J Intern Med 34: , e29–e31. |
[8] | Mulder M , Jansen PJ , Janssen BJ , van de Berg WD , van der Boom H , Havekes LM , de Kloet RE , Ramaekers FC , Blokland A ((2004) ) Low-density lipoprotein receptor-knockout mice display impaired spatial memory associated with a decreased synaptic density in the hippocampus. Neurobiol Dis 16: , 212–219. |
[9] | de Oliveira J , Hort MA , Moreira EL , Glaser V , Ribeiro-do-Valle RM , Prediger RD , Farina M , Latini A , de Bem AF ((2011) ) Positive correlation between elevated plasma cholesterol levels and cognitive impairments in LDL receptor knockout mice: Relevance of cortico-cerebral mitochondrial dysfunction and oxidative stress. Neuroscience 197: , 99–106. |
[10] | de Oliveira J , Moreira EL , dos Santos DB , Piermartiri TC , Dutra RC , Pinton S , Tasca CI , Farina M , Prediger RD , de Bem AF ((2014) ) Increased susceptibility to amyloid-b-induced neurotoxicity in mice lacking the low-density lipoprotein receptor. J Alzheimers Dis 41: , 43–60. |
[11] | de Oliveira J , Engel DF , de Paula GC , Melo HM , Lopes SC , Ribeiro CT , Delanogare E , Moreira JCF , Gelain DP , Prediger RD , Gabilan NH , Moreira ELG , Ferreira ST , de Bem AF ((2020) ) LDL receptor deficiency does not alter brain amyloid-β levels but causes an exacerbation of apoptosis. J Alzheimers Dis 73: , 585–596. |
[12] | Moreira EL , de Oliveira J , Nunes JC , Santos DB , Nunes FC , Vieira DS , Ribeiro-do-Valle RM , Pamplona FA , de Bem AF , Farina M , Walz R , Prediger RD ((2012) ) Age-related cognitive decline in hypercholesterolemic LDL receptor knockout mice (LDLr–/–): Evidence of antioxidante imbalance and increased acetylcholinesterase activity in the prefrontal cortex. J Alzheimers Dis 32: , 495–511. |
[13] | Thirumangalakudi L , Prakasam A , Zhang R , Bimonte-Nelson H , Sambamurti K , Kindy MS , Bhat NR ((2008) ) High cholesterol-induced neuroinflammation and amyloid precursor protein processing correlate with loss of working memory in mice. J Neurochem 106: , 475–485. |
[14] | Moreira EL , de Oliveira J , Engel DF , Walz R , de Bem AF , Farina M , Prediger RD. ((2014) ) Hypercholesterolemia induces short-term spatial memory impairments in mice: Up-regulation of acetylcholinesterase activity as an early and causal event? J Neural Transm (Vienna) 121: , 415–426. |
[15] | Rutkowsky JM , Lee LL , Puchowicz M , Golub MS , Befroy DE , Wilson DW , Anderson S , Cline G , Bini J , Borkowski K , Knotts TA , Rutledge JC , Mouse Metabolic Phenotyping Center Imaging Working Group ((2018) ) Reduced cognitive function, increased blood-brain-barrier transport and inflammatory responses, and altered brain metabolites in LDLr–/–and C57BL/6 mice fed a western diet. PLoS One 13: , e0191909. |
[16] | Sweeney MD , Sagare AP , Zlokovic BV ((2018) ) Blood-brain barrier breakdown in Alzheimer disease and other neurodegenerative disorders. Nat Rev Neurol 14: , 133–150. |
[17] | Murai T , Okuda S , Tanaka T , Ohta H ((2007) ) Characteristics of object location memory in mice: Behavioral and pharmacological studies. Physiol Behav 90: , 116–124. |
[18] | Assini FL , Duzzioni M , Takahashi RN ((2009) ) Object location memory in mice: Pharmacological validation and further evidence of hippocampal CA1 participation. Behav Brain Res 204: , 206–211. |
[19] | Ainge JA , Heron-Maxwell C , Theofilas P , Wright P , de Hoz L , Wood ER ((2006) ) The role of the hippocampus in object recognition in rats: Examination of the influence of task parameters and lesion size. Behav Brain Res 167: , 183–195. |
[20] | Greco SJ , Bryan KJ , Sarkar S , Zhu X , Smith MA , Ashford JW , Johnston JM , Tezapsidis N , Casadesus G ((2010) ) Leptin reduces pathology and improves memory in a transgenic mouse model of Alzheimer’s disease. J Alzheimers Dis 19: , 1155–1167. |
[21] | Spowart-Manning L , van der Staay FJ ((2004) ) The T-maze continuous alternation task for assessing the effects of putative cognition enhancers in the mouse. Behav Brain Res 151: , 37–46. |
[22] | Hawkins BT , Ocheltree SM , Norwood KM , Egleton RD ((2007) ) Decreased blood-brain barrier permeability to fluorescein in streptozotocin-treated rats. Neurosci Lett 411: , 1–5. |
[23] | Bohara M , Kambe Y , Nagayama T , Tokimura H , Arita K , Miyata A ((2014) ) C-type natriuretic peptide modulates permeability of the blood-brain barrier. J Cereb Blood Flow Metab 34: , 589–596. |
[24] | De Paula GC , de Oliveira J , Engel DF , Lopes SC , Moreira ELG , Figueiredo CP , Prediger RD , Fabro de Bem A ((2020) ) Red wine consumption mitigates the cognitive impairments in low-density lipoprotein receptor knockout (LDLr–/–) mice. Nutr Neurosci 7: , 1–11. |
[25] | De Oliveira J , Moreira EL , Mancini G , Hort MA , Latini A , Ribeiro-do-Valle RM , Farina M , da Rocha JB , de Bem AF ((2013) ) Diphenyl diselenide prevents cortico-cerebral mitochondrial dysfunction and oxidative stress induced by hypercholesterolemia in LDL receptor knockout mice. Neurochem Res 38: , 2028–2036. |
[26] | Elder GA , Ragnauth A , Dorr N , Franciosi S , Schmeidler J , Haroutunian V , Buxbaum JD ((2008) ) Increased locomotor activity in mice lacking the low-density lipoprotein receptor. Behav Brain Res 191: , 256–265. |
[27] | Czupalla CJ , Liebner S , Devraj K ((2014) ) In vitro models of the blood-brain barrier. Methods Mol Biol 1135: , 415–437. |
[28] | DiSabato DJ , Quan N , Godbout JP ((2016) ) Neuroinflammation: The devil is in the details. J Neurochem 139: , 136–153. |
[29] | Rizor A , Pajarillo E , Johnson J , Aschner M , Lee E ((2019) ) Astrocytic oxidative/nitrosative stress contributes to Parkinson’s disease pathogenesis: The dual role of reactive astrocytes. Antioxidants (Basel) 8: , 265. |
[30] | Saha RN , Pahan K ((2006) ) Regulation of inducible nitric oxide synthase gene in glial cells. Antioxid Redox Signal 8: , 929–947. |
[31] | Pećin I , Hartgers ML , Hovingh GK , Dent R , Reiner Ž ((2017) ) Prevention of cardiovascular disease in patients with familial hypercholesterolaemia: The role of PCSK9 inhibitors. Eur J Prev Cardiol 24: , 1383–1401. |
[32] | Ishibashi S , Brown MS , Goldstein JL , Gerard RD , Hammer RE , Herz J ((1993) ) Hypercholesterolemia in low density lipoprotein receptor knockout mice and its reversal by adenovirus-mediated gene delivery. J Clin Invest 92: , 883–893. |
[33] | Wang CY , Liao JK ((2012) ) A mouse model of diet-induced obesity and insulin resistance. Methods Mol Biol 821: , 421–433. |
[34] | Hyoju SK , Zaborin A , Keskey R , Sharma A , Arnold W , van den Berg F , Kim SM , Gottel N , Bethel C , Charnot-Katsikas A , Jianxin P , Adriaansens C , Papazian E , Gilbert JA , Zaborina O , Alverdy JC ((2019) ) Mice fed an obesogenic Western diet, administered antibiotics, and subjected to a sterile surgical procedure develop lethal septicemia with multidrug-resistant pathobionts. mBio 10: , e00903–19. |
[35] | Ullrich C , Pirchl M , Humpel C ((2010) ) Hypercholesterolemia in rats impairs the cholinergic system and leads to memory deficits. Mol Cell Neurosci 45: , 408–417. |
[36] | Kaya M , Ahishali B ((2011) ) Assessment of permeability in barrier type of endothelium in brain using tracers: Evans blue, sodium fluorescein, and horseradish peroxidase. Methods Mol Biol 763: , 369–382. |
[37] | Kanoski SE , Davidson TL ((2011) ) Western diet consumption and cognitive impairment: Links to hippocampal dysfunction and obesity. Physiol Behav 103: , 59–68. |
[38] | Deng J , Zhao F , Yu X , Zhao Y , Li D , Shi H , Sun Y ((2014) ) Expression of aquaporin 4 and breakdown of the blood-brain barrier after hypoglycemia-induced brain edema in rats. PLoS One 9: (9), e107022. |
[39] | Mader S , Brimberg L ((2019) ) Aquaporin-4 water channel in the brain and its implication for health and disease. Cells 8: , 90. |
[40] | Förster C ((2008) ) Tight junctions and the modulation of barrier function in disease. Histochem Cell Biol 130: , 55–70. |
[41] | Stamatovic SM , Johnson AM , Keep RF , Andjelkovic AV ((2016) ) Junctional proteins of the blood-brain barrier: New insights into function and dysfunction. Tissue Barriers 4: , e1154641. |
[42] | Berndt P , Winkler L , Cording J , Breitkreuz-Korff O , Rex A , Dithmer S , Rausch V , Blasig R , Richter M , Sporbert A , Wolburg H , Blasig IE , Haseloff RF ((2019) ) Tight junction proteins at the blood-brain barrier: Far more than claudin-5. Cell Mol Life Sci 76: , 1987–2002. |
[43] | Greene C , Campbell M ((2016) ) Tight junction modulation of the blood brain barrier: CNS delivery of small molecules. Tissue Barriers 4: , e1138017. |
[44] | Greene C , Hanley N , Campbell M ((2019) ) Claudin-5: Gatekeeper of neurological function. Fluids Barriers CNS 16: , 3. |
[45] | Jiao H , Wang Z , Liu Y , Wang P , Xue Y ((2011) ) Specific role of tight junction proteins claudin-5, occludin, and ZO-1 of the blood-brain barrier in a focal cerebral ischemic insult. J Mol Neurosci 44: , 130–139. |
[46] | Ohtsuki S , Sato S , Yamaguchi H , Kamoi M , Asashima T , Terasaki T ((2007) ) Exogenous expression of claudin-5 induces barrier properties in cultured rat brain capillary endothelial cells. J Cell Physiol 210: , 81–86. |
[47] | Franciosi S , Gama Sosa MA , English DF , Oler E , Oung T , Janssen WG , De Gasperi R , Schmeidler J , Dickstein DL , Schmitz C , Gandy S , Hof PR , Buxbaum JD , Elder GA ((2009) ) Novel cerebrovascular pathology in mice fed a high cholesterol diet. Mol Neurodegener 4: , 42. |
[48] | Yamamoto M , Guo DH , Hernandez CM , Stranahan AM ((2019) ) Endothelial Adora2a activation promotes blood-brain barrier breakdown and cognitive impairment in mice with diet-induced insulin resistance. J Neurosci 39: , 4179–4192. |
[49] | Daneman R , Prat A ((2015) ) The blood-brain barrier. Cold Spring Harb Perspect Biol 7: , a020412. |
[50] | Sweeney MD , Zhao Z , Montagne A , Nelson AR , Zlokovic BV ((2019) ) Blood-brain barrier: From physiology to disease and back. Physiol Rev 99: , 21–78. |
[51] | Saito S , Ihara M ((2016) ) Interaction between cerebrovascular disease and Alzheimer pathology. Curr Opin Psychiatry 29: , 168–173. |
[52] | Machado AE , de Sousa G , Mancini G , Faria MS , de Bem AF , Moreira ELG ((2018) ) Hypercholesterolemia impairs contextual fear conditioning memory formation in female mice: Evidence for cholinergic dysfunction. Neuroreport 29: , 1140–1143. |
[53] | Lopes JB , de Oliveira J , Engel DF , de Paula GC , Moreira EL , de Bem AF ((2015) ) Efficacy of donepezil for cognitive impairments in familial hypercholesterolemia: Preclinical proof of concept. CNS Neurosci Ther 21: , 964–966. |
[54] | Sanderson DJ , Bannerman DM ((2012) ) The role of habituation in hippocampus-dependent spatial working memory tasks: Evidence from GluA1 AMPA receptor subunit knockout mice. Hippocampus 22: , 981–994. |
[55] | Rial D , Castro AA , Machado N , GarÇão P , GonÇalves FQ , Silva HB , Tomé AR , Köfalvi A , Corti O , Raisman-Vozari R , Cunha RA , Prediger RD ((2014) ) Behavioral phenotyping of Parkin-deficient mice: Looking for early preclinical features of Parkinson’s disease. PLoS One 9: , e114216. |
[56] | Bell RD , Winkler EA , Singh I , Sagare AP , Deane R , Wu Z , Holtzman DM , Betsholtz C , Armulik A , Sallstrom J , Berk BC , Zlokovic BV ((2012) ) Apolipoprotein E controls cerebrovascular integrity via cyclophilin A. Nature 485: , 512–516. |
[57] | Kim SY , Buckwalter M , Soreq H , Vezzani A , Kaufer D ((2012) ) Blood-brain barrier dysfunction-induced inflammatory signaling in brain pathology and epileptogenesis. Epilepsia 6: , 37–44. |
[58] | Zlokovic BV ((2008) ) The blood-brain barrier in health and chronic neurodegenerative disorders. Neuron 57: , 178–201. |
[59] | Tucsek Z , Toth P , Sosnowsk D , Gautam T , Mitschelen M , Koller A , Szalai G , Sonntag WE , Ungvari Z , Csiszar A ((2014) ) Obesity in aging exacerbates blood brain barrier disruption, neuroinflammation and oxidative stress in the mouse hippocampus: Effects on expression of genes involved in betaamyloid generation and Alzheimer’s disease. J Gerontol A Biol Sci Med Sci 69: , 1212–1226. |
[60] | Fulop GA , Ahire C , Csipo T , Tarantini S , Kiss T , Balasubramanian P , Yabluchanskiy A , Farkas E , Toth A , Nyúl-Tóth Á , Toth P , Csiszar A , Ungvari Z ((2019) ) Cerebral venous congestion promotes blood-brain barrier disruption and neuroinflammation, impairing cognitive function in mice. Geroscience 41: , 575–589. |
[61] | Andjelkovic AV , Nikolic B , Pachter JS , Zecevic N ((1998) ) Macrophages/microglial cells in human central nervous system during development: An immunohistochemical study. Brain Res 814: , 13–25. |
[62] | Chen X , Ghribi O , Geiger JD ((2010) ) Caffeine protects against disruptions of the blood-brain barrier in animal models of Alzheimer’s and Parkinson’s diseases. J Alzheimers Dis 20: (Suppl 1), S127–141. |
[63] | Engel DF , Grzyb AN , de Oliveira J , Pötzsch A , Walker TL , Brocardo PS , Kempermann G , de Bem AF ((2019) ) Impaired adult hippocampal neurogenesis in a mouse model of familial hypercholesterolemia: A role for the LDL receptor and cholesterol metabolism in adult neural precursor cells. Mol Metab 30: , 1–15. |
[64] | Mathews KJ , Allen KM , Boerrigter D , Ball H , Shannon Weickert C , Double KL ((2017) ) Evidence for reduced neurogenesis in the aging human hippocampus despite stable stem cell markers. Aging Cell 16: , 1195–1199. |
[65] | Sochocka M , Diniz BS , Leszek J ((2017) ) Inflammatory response in the CNS: Friend or foe? Mol Neurobiol 54: , 8071–8089. |
[66] | Li K , Li J , Zheng J , Qin S ((2019) ) Reactive astrocytes in neurodegenerative diseases. Aging Dis 10: , 664–675. |
[67] | Czapski GA , Cakala M , Chalimoniuk M , Gajkowska B , Strosznajder JB ((2007) ) Role of nitric oxide in the brain during lipopolysaccharide-evoked systemic inflammation. J Neurosci Res 85: , 1694–1703. |
[68] | Yuste JE , Tarragon E , Campuzano CM , Ros-Bernal F ((2015) ) Implications of glial nitric oxide in neurodegenerative diseases. Front Cell Neurosci 9: , 322. |
[69] | Eyre H , Baune BT ((2012) ) Neuroplastic changes in depression: A role for the immune system. Psychoneuroendocrinology 37: , 1397–1416. |
[70] | Gadient RA , Otten UH ((1997) ) Interleukin-6 (IL-6)–a molecule with both beneficial and destructive potentials. Prog Neurobiol 52: , 379–390. |
[71] | Scheller J , Chalaris A , Schmidt-Arras D , Rose-John S ((2011) ) The pro- and anti-inflammatory properties of the cytokine interleukin-6. Biochim Biophys Acta 1813: , 878–888. |
[72] | Shaftel SS , Griffin WS , O’Banion MK ((2008) ) The role of interleukin-1 in neuroinflammation and Alzheimer disease: An evolving perspective. J Neuroinflammation 5: , 7. |
[73] | Price JL , Ko AI , Wade MJ , Tsou SK , McKeel DW , Morris JC ((2001) ) Neuron number in the entorhinal cortex and CA1 in preclinical Alzheimer disease. Arch Neurol 58: , 1395–402. |
[74] | Kanoski SE , Zhang Y , Zheng W , Davidson TL ((2010) ) The effects of a high-energy diet on hippocampal function and blood-brain barrier integrity in the rat. J Alzheimers Dis 21: , 207–219. |