Mitochondrial Interaction with Serotonin in Neurobiology and Its Implication in Alzheimer’s Disease
Abstract
Alzheimer’s disease (AD) is a lethal neurodegenerative disorder characterized by severe brain pathologies and progressive cognitive decline. While the exact cause of this disease remains unknown, emerging evidence suggests that dysregulation of neurotransmitters contributes to the development of AD pathology and symptoms. Serotonin, a critical neurotransmitter in the brain, plays a pivotal role in regulating various brain processes and is implicated in neurological and psychiatric disorders, including AD. Recent studies have shed light on the interplay between mitochondrial function and serotonin regulation in brain physiology. In AD, there is a deficiency of serotonin, along with impairments in mitochondrial function, particularly in serotoninergic neurons. Additionally, altered activity of mitochondrial enzymes, such as monoamine oxidase, may contribute to serotonin dysregulation in AD. Understanding the intricate relationship between mitochondria and serotonin provides valuable insights into the underlying mechanisms of AD and identifies potential therapeutic targets to restore serotonin homeostasis and alleviate AD symptoms. This review summarizes the recent advancements in unraveling the connection between brain mitochondria and serotonin, emphasizing their significance in AD pathogenesis and underscoring the importance of further research in this area. Elucidating the role of mitochondria in serotonin dysfunction will promote the development of therapeutic strategies for the treatment and prevention of this neurodegenerative disorder.
INTRODUCTION
Serotonin, also known as 5-hydroxytryptamine, is a member of the monoamine neurotransmitter family [1, 2]. In the central nervous system, serotonin is predominantly synthesized and released by the dorsal raphe nucleus, where serotonin-producing neurons reside [1, 2]. As a critical component of the diffuse nervous system, serotonin signaling contributes to the modulation of multiple key brain processes, including mood and emotion, cognition and memory, appetite and satiety, the sleep-wake cycle, and social interactions [3–10]. Accordingly, serotonin plays a pivotal role in brain health, with its dysregulation has been linked to a plethora of neurological and psychiatric disorders including Alzheimer’s disease (AD) [11–13]. While serotonin itself is not the underlying cause of AD, the contribution of serotonin signaling deregulation to the progression of symptoms reveals unique therapeutic opportunities; these serotonin signaling-targeting therapies having demonstrated substantial therapeutic benefits [14–16]. Therefore, elucidating the regulatory mechanisms of serotonin has a positive impact on our understanding of both neurobiology and neurodegeneration.
Mitochondria are vital organelles that support neuronal functions through their roles in producing energy, regulating calcium homeostasis, and maintaining oxidation-reduction (redox) balance [17, 18]. Mitochondrial dysfunction has been consistently identified as a key factor underlying synaptic injury and neuronal stress in AD, which has been extensively reviewed elsewhere [19–22]. Recently, not only has the impact of serotonin on mitochondrial fitness emerged, but mounting evidence also suggests the importance of mitochondrial function in the regulation of serotonin homeostasis [11, 23]. Reflecting the growing interest in the role of serotonin in neurological decline and wellbeing, this review aims to summarize recent research progress in the intertwined relationship between brain mitochondria and serotonin. Additionally, we discuss the association between mitochondrial dysfunction and serotonin dysregulation in AD and its therapeutic potential for the treatment of this devastating neurological disorder.
MITOCHONDRIA AND SEROTONIN REGULATION IN NEUROBIOLOGY
Mitochondrial function and neuronal serotonin transmission and recycling
Serotonin is synthesized through a two-step reaction. Firstly, tryptophan hydroxylation converts tryptophan into 5-hydroxytryptophan (5-HTP) by tryptophan hydroxylase isoform 2. This is followed by aromatic L-amino acid decarboxylase-mediated 5-HTP decarboxylation [24, 25]. In the presynapse, the synthesized serotonin is then immediately packaged into synaptic vesicles by the vesicular monoamine transporter (VMAT), especially VMAT2, and subsequently released into the synaptic cleft, where it interacts with serotonin receptors in the postsynaptic membrane [26]. A significant portion of the released serotonin is recaptured by presynapses via the serotonin transporter (SERT) [27, 28] and under further degradation by mitochondrial enzymes including monoamine oxidase isoform A (MAO-A) [29], thus completing the cycle of serotonin metabolism. Although the evidence supporting the direct involvement of mitochondria in serotonin synthesis is currently limited, the role of mitochondria in the regulation of serotonin transmission and breakdown is becoming increasingly evident.
VMAT2 is the major protein responsible for the loading of serotonin into synaptic vesicles for storage and subsequent release [26]. Previous studies have determined that the function of VMAT2 is fueled by the ATP-consuming V-type H+-ATPase [30] and regulated by phosphorylation modification [31, 32], both of which are ATP-dependent processes. Additionally, synaptic-vesicular transport requires high energy expenditure [33]. Given that mitochondria are the primary providers of ATP in the presynapse [34], it is plausible that mitochondrial ATP production is crucial for serotonin storage and transport. Indeed, in our recent study, we have observed impaired serotonin release in mouse hippocampal slices subjected to stress induced by carbonyl cyanide-p-trifluoromethoxy phenylhydrazone, a mitochondrial uncoupling agent [11]. These findings suggest that mitochondrial function plays an integral role in serotonin release and support the notion of mitochondrial involvement in serotonin neurotransmission. Moreover, the activity of SERT relies on the maintenance of a cross-membrane Na+/K+ gradient, which is created by the energy-consuming Na+/K+ ATPase pumps [35]. As a result, impaired mitochondrial bioenergetics could also potentially compromise serotonin recycling and reuptake processes.
It is worth noting that mitochondria serve as a stabilizer of Ca2+ homeostasis in neurons [36, 37]. The VMAT2-mediated filling and release of serotonin within synaptic vesicles involve Ca2+ signaling, including the participation of Ca2+-dependent activator proteins of secretion 1 and 2 [38]. Furthermore, the exocytosis of synaptic vesicles is modulated by Ca2+ signaling, which can be influenced by mitochondrial sequestration of Ca2+ [39]. In this regard, mitochondrial regulation of Ca2+ may also contribute to serotonin transmission, and impaired mitochondrial Ca2+ retention capacity can impede serotonin regulation. The direct link between mitochondrial Ca2+ handling capacity and serotonin release is determined in mice with heterogeneous loss of mitochondrial adenine nucleotide translocase 1 (ANT1) [40]. Serotonin-producing cells in the dorsal raphe nucleus demonstrate susceptibility to ANT1 deficiency-induced mitochondrial dysfunction, which coincides with dorsal raphe neuronal hyper-depolarization and resultant increases in serotonin turnover [40]. Due to the involvement of ANTs including ANT1 in the regulation of mitochondrial Ca2+ through mitochondrial permeability transition [41], it is proposed that the influence of this mitochondrial protein deficit on serotonin regulation is, at least in part, associated with Ca2+-related perturbations [40]. These findings therefore highlight the need for further comprehensive investigations into the role of mitochondrial Ca2+ handling in serotonin signaling.
Mitochondrial enzymes and serotonin degradation
MAO-A, an isoform of MAO, is an outer mitochondrial membrane-bound enzyme that converts serotonin to its metabolite, 5-hydroxy-3-indolacetaldehyde (5-HIAL) [42]. This toxic biogenic aldehyde is further processed into the less toxic 5-hydroxy-3-indolacetic acid by a mitochondrial matrix protein, aldehyde dehydrogenase 2 (ALDH2), for excretion [28, 43]. Previous studies have consistently reported that loss of function of MAO-A reduces serotonin breakdown in the brain, leading to behavioral abnormalities in humans and rodents [44–49]. Furthermore, suppressed ALDH2 activity disrupts serotonin metabolism, resulting in the accumulation of the toxic serotonin metabolite 5-HIAL and leading to pathological consequences [28, 50, 51]. The significance of these mitochondrial enzymes in serotonin metabolism thus underscores the key role of mitochondria in maintaining neuronal serotonin homeostasis.
SEROTONIN AND MITOCHONDRIAL REGULATION IN NEUROBIOLOGY
Despite the role of mitochondria in regulating serotonin transmission and metabolism, the impact of serotonin on neuronal mitochondrial fitness has been accentuated in recent years [23, 52, 53]. A recent finding demonstrated that serotonin induces mitochondrial biogenesis in mouse cortical neurons [23]. Such a mitochondrial biogenesis-promoting effect of serotonin is achieved through the stimulation of serotonin receptor 2A (5-HT2A), the receptor’s downstream signaling involving sirtuin 1, and the peroxisome proliferator-activated receptor gamma coactivator 1 alpha [23]. Interestingly, serotonin signaling-induced mitochondrial biogenesis and subsequent alterations in cellular metabolism, favoring increased oxidative phosphorylation, have also been reported in breast cancer [54]. This suggests that the role of serotonin in regulating mitochondria is not exclusive to neural cells. Additionally, the influence of the SERT on mitochondria has been reported [55]. A previous study showed a negative correlation between SERT expression and mitochondrial copy number in the brains of male rats, while reduced SERT expression led to a decrease in mitochondrial copy number in females [55]. Regardless of the unclear mechanisms underlying the impact of SERT on mitochondria and the sex-related dimorphic regulation, these findings implicate the involvement of serotonin signaling in mitochondrial biology. Moreover, Reddy and the colleagues in a recent study reported that citalopram, a selective inhibitor of SERT, promotes mitochondrial fusion, potentiates mitochondrial generation, and enhances mitochondrial turnover through mitophagy in a mouse hippocampal cell line [56]. Although it cannot be excluded the non-SERT-related off-target effects of citalopram on mitochondria, these results echo the impact of SERT expression modulation on mitochondrial regulation [55], further supporting the potential association of serotonin signaling with mitochondrial fitness even in non-serotonin-producing neural cells.
MITOCHONDRIA AND SEROTONIN IN ALZHEIMER’S DISEASE
Serotonin deficiency in AD
AD is a chronic neurodegenerative disorder characterized by pathological features, including amyloid-β (Aβ) aggregation, abnormal tau phosphorylation, synaptic and neuronal degeneration. In addition to the defining manifestation of progressive memory loss, patients with AD frequently demonstrate psychiatric symptoms, including mood and emotional fluctuations, social behavioral changes, as well as sleep disturbances [57–64]. Both depression and anxiety have been repeatedly identified in patients with AD or even in the prodromal stage of AD [65–70]. Previous studies reported depressive and anxiety symptoms in a significant portion of patients in different stages of AD with mild to severe cognitive deficits [71–73]. Furthermore, meta-analytic studies have identified mood disorders including depression and anxiety as strong risk factors for the development of AD [74–79]. Because of the well-established central role of serotonin dysregulation in mood disturbances including depression and anxiety problems [80–83], the strong negative association of depression and anxiety with cognitive performance in AD patients determined in previous clinical studies [68, 70] justifies further investigations of serotonin dysregulation in this neurodegenerative disorder. Although AD is not a typical neurotransmitter disorder, serotonin deficiency in the brains of AD patients has long been observed to be associated with mood disturbances and cognitive impairment [84, 85]. The loss of serotoninergic neurons in the raphe nucleus was first reported four decades ago and has been consistently observed to coincide with reduced serotonin and its metabolites since then [85–93]. The lesions in the raphe nucleus in patients in the early stage of AD further implicate an association of serotonin dysregulation with the development of this neurodegenerative disorder [94, 95]. Consistent with the findings in patients [85, 93, 96–98], a reduction in serotonin-producing neurons in the raphe nucleus and a decrease in serotonergic fiber density in multiple brain regions including the neocortex and hippocampus has been determined in several mouse models of AD-like brain amyloidosis [11, 99]. These findings suggest detrimental impacts of Aβ on the fitness of serotonergic neurons. In addition to Aβ deposition, abnormal tau aggregation constitutes another AD-related pathological characteristic [100]. Previous studies have also determined neurofibrillary tangles composed of hyperphosphorylated tau in the dorsal raphe nucleus in AD patients [101–104] and proposed an association of tauopathy with AD-related serotoninergic neuron degeneration and serotonin deprivation [104, 105]. The direct link between tau abnormalities and serotonin dysregulation was determined in further animal experiments. Marcinkiewcz’s group showed that overexpression of human tau in mice induces degenerative changes of serotonin-producing neurons [106]. Accordingly, tau depletion prevents chronic stress-mediated mouse brain serotonin loss [107]. Moreover, manipulating serotonin signaling reciprocally promotes the development of tau aggregation [108, 109]. These findings in together highlight the interactions between serotoninergic system and tau pathology. However, a previous study reported a paradoxical increase in hippocampal serotoninergic fibers in 3xTg mice, another familial AD mouse model with brain amyloidosis and tauopathy [110]. This discrepancy between 3xTg mice and other mouse models with brain amyloidosis and/or tauopathy may reflect differences in the ability of different mouse models to recapitulate AD pathologies, raising interesting questions regarding mouse model selection for studying AD-related disruption of the serotoninergic system. Of note, despite the deleterious impact of Aβ and tau on serotoninergic neurons, we cannot fully refute the possibility that tau and Aβ or amyloid-β protein precursor may have unknown interference with each other’s effect on serotoninergic neurons, impeding the degeneration of these neurons in 3xTg mice. This needs further investigation. In addition to perturbations in serotonin and serotoninergic neurons, lowered levels of the SERT have been identified in multiple brain regions in patients at various stages of AD [85, 91]. Of note, some serotonin-enhancing agents such as citalopram also display a Aβ-lowering capability [56, 111, 112], possibly through the activation of serotonin receptors including 5-HT2 R and 5-HT4 R [113, 114]. Moreover, serotonin receptor modulators also demonstrate therapeutic effects against tau pathology [108]. In addition to the therapeutic benefits observed with selective serotonin reuptake inhibitors (SSRIs) and serotonin receptor modulators in AD patients and animal models [14–16, 108, 111, 115–117], clinical studies have also found protective effects of SSRIs against cognitive decline and cortical atrophy in older adults with concurrent mild cognitive impairment and depression [15]. Therefore, these findings strongly demonstrate serotonin dysregulation in the etiopathogenesis of this neurodegenerative disorder.
Mitochondria dysfunction and serotonin deficiency in AD-related conditions
Although mitochondria dysfunction has been well-documented in AD paradigms [19, 118–120], previous studies on mitochondrial dysfunction in AD overwhelmingly focused on mitochondria in pyramidal neurons in the neocortex and hippocampus. It should be noted that, despite the vulnerability of the limbic system to neuronal stress, degenerative changes and AD-associated pathologies in the brain stem, including the serotonin-producing raphe nucleus, are also prominent in patients with AD [93, 96, 97]. To this end, although the investigation of mitochondrial function in serotoninergic neurons in AD-related conditions has been seldom explored, the mitochondrial sensitivity to AD-associated pathologies and the reported deleterious impacts of mitochondrial dysfunction on raphe neuron functions [40] thus warrant a hypothesis of a role of mitochondrial dysfunction in serotoninergic neuronal stress in this neurodegenerative disorder.
Hippocampal lesions are a key pathological feature underlying the symptoms of AD [121–123]. The hippocampus, a vital brain region involved in memory storage, information processing, and mood modulation [124–127], heavily relies on serotonin for its optimal functioning [128–130], aligning with the findings that serotoninergic neurons innervate the neurons in the hippocampus, and all types of serotonin receptors are present in this region [131, 132]. SSRIs, a class of medications that enhance serotonin levels, have shown therapeutic effect in alleviating AD symptoms [14–16, 111, 115–117], further emphasizing the impact of serotonin dysregulation on hippocampal-related cognitive and mood disturbances associated with the disease. Our recent study has provided evidence of concurrent impairments in hippocampal serotonin release and mitochondrial morphological control within hippocampal serotoninergic fibers in 5xFAD mice, a mouse model of familial AD-like brain amyloidosis [11]. Serotoninergic neurons in Aβ-rich environments exhibited reduced mitochondrial bioenergetics and increased mitochondrial fragmentation [11]. Additionally, the administration of a mitochondria-uncoupling agent resulted in the suppression of serotonin release in hippocampal slices [11]. Considering the significance of mitochondrial health in serotonin transmission, these findings offer important insights into the contribution of mitochondrial dysfunction to serotonin dysregulation in this neurodegenerative disease, warranting further investigation into the role of mitochondrial dysfunction in raphe nucleus neurodegeneration within AD-relevant pathological settings. Moreover, given the role of serotonin in promoting neuronal mitochondrial biogenesis, there may exist a vicious cycle of mitochondrial dysfunction and serotonin failure that reinforces each other, leading to damage in both serotoninergic and non-serotonergic neurons in AD brains.
Other than the direct influence of mitochondrial dysfunction on serotonin transmission, changes in MAO, a mitochondrial enzyme responsible for serotonin metabolism, have been implicated in AD-relevant settings. In addition, altered MAO activity has a correlation with AD neuropsychiatric symptoms and pathological changes, including amyloid deposition and neurofibrillary tangles [133–137]. So far, there is no evidence of the impact of MAO alterations on serotonin metabolism in AD brains. However, the administration of MAO inhibitors does exhibit clinical benefits to some extent in mitigating AD symptoms, including psychiatric symptoms [138, 139]. Although serotonin is not the sole substrate of MAO [29], the therapeutic effect of MAO inhibitors in alleviating psychiatric symptoms arguably implies an influence of MAO changes on serotonin signaling in AD. Nevertheless, declining serotonin metabolites in AD patients may not solely arise from lowered serotonin synthesis, but also from enhanced serotonin degradation by MAO. In this context, we cannot refute the possibility that MAO, especially MAO-A, is also hyperactive in serotoninergic neurons, leading to pathological consequences such as serotonin degradation and subsequent disturbances in serotonin signaling in AD-related conditions. However, it should be noted that a previous study reported a simultaneous decline in both serotonin and MAO-B in platelets from very late-stage AD patients. So far, there are mixed results regarding MAO-B expression in neural cells. MAO-B expression has been previously identified in monoaminergic including serotoninergic neurons [140–143]. In contrast, other reports including a recent neuroimaging study showed that MAO-B is not expressed by neurons [144, 145]. Regardless of the yet-unsettled question of MAO-B expression in neurons, there is no evidence of a role of MAO-B in neuronal serotonin metabolism; and MAO-A is the only genetically determined MAO family member to be responsible for serotonin degradation to date [146]. In this regard, the concurrence of platelet serotonin and MAO-B reduction may have limited capacity to indicate serotonin metabolism in AD platelets but is rather a reflection of systemic degenerative changes in the end stage of this neurodegenerative disorder. Given the strong relevance of MAO-A to serotonin regulation, the findings of the negative impact of MAO-A activity on mitochondrial bioenergetics in cortical neurons [147] further strengthened the hypothesis that MAO-A hyperactivity may exacerbate mitochondrial dysfunction, resulting in a downward spiral of worsened serotonin dysregulation in AD.
Fig. 1
Schematic diagram of the interactions between serotonin regulation and mitochondria in serotoninergic neurons in neurobiology and AD. In physiological conditions, mitochondria in serotoninergic neurons support the metabolism of serotonin and brain serotonin homeostasis, leading to normal mood and cognition. The beneficial effect of serotonin system on mitochondrial fitness thus forms a virtuous cycle with mitochondria-related serotonin regulation. In AD-relevant pathological settings, Mitochondria in serotoninergic neurons demonstrate impaired function and enhanced MAO-A activity in response to AD-associated pathological molecules including Aβ and pathological tau, resulting in serotonin deficiency. The serotonin system dysregulation eventually promotes mood disturbances and cognitive impairment, which reinforces each other. Furthermore, brain amyloidosis and tauopathy as well as serotoninergic neuronal mitochondrial dysfunction are exacerbated by serotonin dysregulation, culminating in a vicious cycle.
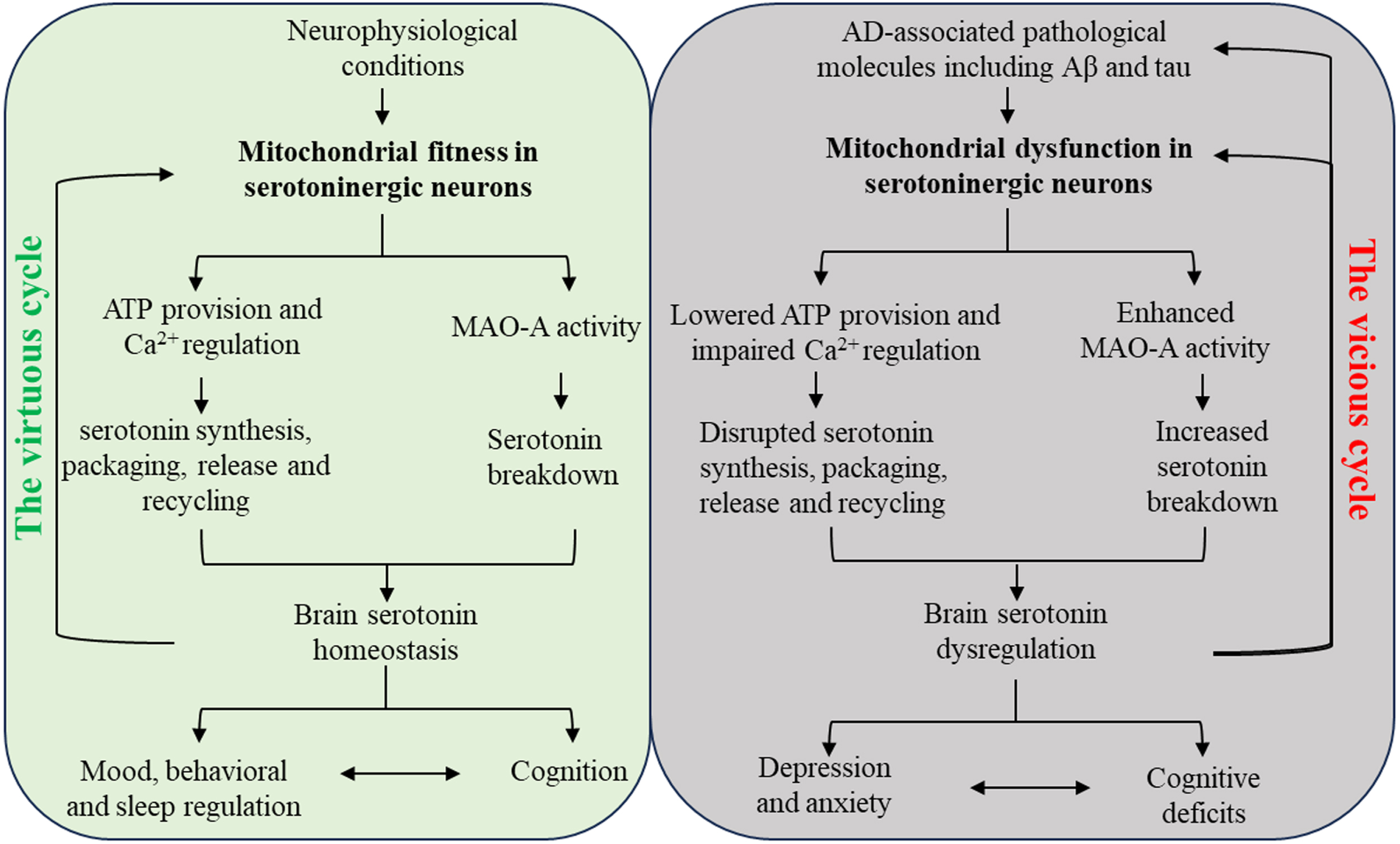
CONCLUSIVE REMARKS AND FUTURE PERSPECTIVES
In conclusion, the interplay between mitochondria and serotonin signifies a complex relationship between these two entities with substantial implications for neurobiology. In the context of AD, this delicate balance between serotonin and mitochondrial function can be disrupted, giving rise to a vicious cycle of mitochondrial dysfunction and serotonin failure that mutually reinforce each other, resulting in pathological consequences in this neurodegenerative disorder (Fig. 1). There are several outstanding questions that remain unresolved. It is unclear whether mitochondrial dysfunction or serotonin dysregulation in raphe neurons occurs first during the development of this age-related disorder. Of note, the scientific community has yet to reach a consensus on whether raphe neurons are sensitive to the aging process has not yet reached a consensus [148, 149]. However, it is commonly accepted that aging renders neuronal mitochondria vulnerable to functional deficits [150–154]. In this regard, it can be hypothesized that age-related mitochondrial dysfunction is an initiating factor for the damage of raphe neurons, leading to serotonin failure in the elderly at risk of AD. However, we cannot fully exclude the possibility that mitochondrial dysfunction or serotonin dysregulation in raphe neurons develops independently and exacerbates each other with AD progression, given the yet-elusive complicated regulation of serotonin metabolism and early demonstration of raphe nucleus degeneration in early AD [94, 95]. Another question that merits consideration is the influence of disrupted serotonin and mitochondrial interactions on the development of neuroinflammation in AD brains. Growing evidence suggests that both serotonin dysregulation and mitochondrial dysfunction contribute to inflammatory neuronal damage [155–160]. In this context, the investigation of serotonin and mitochondrial dysfunction should not be limited to neurons but should also be extended to glial cell pathology in AD. Furthermore, mitochondria in neurons within the diffuse modulatory nervous system have long been neglected in the study of mitochondrial dysfunction in AD. It is important to recognize that dysfunction in serotoninergic, dopaminergic, and cholinergic neurons within the diffuse modulatory nervous system also play a significant role in AD-associated pathology and contribute to the development of the disease [84, 161–163]. Therefore, there is an urgent need for neuron type-specific investigations to comprehensively understand the mitochondrial pathways involved in this devastating neurological disorder. Lastly, it is increasingly recognized that serotonin-modulating therapies, especially SSRIs, demonstrate racial and ethnic disparities in their efficacy [164, 165]. Moreover, SSRIs and serotonin–norepinephrine reuptake inhibitors (SNRIs) may risk the elderly to increase adverse cardiovascular events [166, 167]. Given these critical concerns about SSRIs and SNRIs, targeting mitochondrial dysfunction may hold promise to be a better strategy for the restoration of brain serotonin homeostasis for the amelioration of AD cognitive and mood symptoms.
In summary, understanding the complex relationship between mitochondria and serotonin is essential for unraveling the mechanisms behind serotonin dysregulation in AD. Further research is needed to explore these mechanisms and their potential as therapeutic targets to restore serotonin homeostasis and alleviate AD symptoms. The findings presented in this review underscore the significance of developing novel therapeutic strategies that target both mitochondrial dysfunction and serotonin dysregulation for the prevention and treatment of AD.
ACKNOWLEDGMENTS
The authors have no acknowledgments to report.
FUNDING
This work was supported by research funding from Brightfocus Foundation research grant A2022036 S to LG, KU career development grant 2302009 to LG, NIH P30 AG072973 to the University of Kansas Alzheimer’s Disease Research Center’s Research Education Component, and REC fellowship to JT.
CONFLICT OF INTEREST
The authors have no competing interests to disclose.
REFERENCES
[1] | Vertes RP ((1991) ) A PHA-L analysis of ascending projections of the dorsal raphe nucleus in the rat. J Comp Neurol 313: , 643–668. |
[2] | McLaughlin DP , Little KY , Lopez JF , Watson SJ ((1996) ) Expression of serotonin transporter mRNA in human brainstem raphe nuclei. Neuropsychopharmacology 15: , 523–529. |
[3] | Gottlieb LS , Broitman SA , Vitale JJ , Zamcheck W ((1960) ) Failure of endogenous serotonin to produce lesions of the carcinoid syndrome. Studies on mouse mastocytoma. Arch Pathol 69: , 77–81. |
[4] | Park HS , Kim TW , Park SS , Lee SJ ((2020) ) Swimming exercise ameliorates mood disorder and memory impairment by enhancing neurogenesis, serotonin expression, and inhibiting apoptosis in social isolation rats during adolescence. J Exerc Rehabil 16: , 132–140. |
[5] | Pak K , Shin S , Kim K , Seok JW , Kim SJ , Kim IJ ((2019) ) Memory performance associates with serotonin transporter in midbrain and pons in healthy subjects. Hell J Nucl Med 22: , 194–199. |
[6] | Liu Y , Liu J , Jiao SR , Liu X , Guo Y , Zhang J , Yang J , Xie W , Wang HS , Zhang L ((2019) ) Serotonin(1A) receptors in the dorsal hippocampus regulate working memory and long-term habituation in the hemiparkinsonian rats. Behav Brain Res 376: , 112207. |
[7] | Voigt JP , Fink H ((2015) ) Serotonin controlling feeding and satiety. Behav Brain Res 277: , 14–31. |
[8] | Voigt JP , Raasch W , Hortnagl H , Bader M , Fink H , Johren O ((2008) ) Changes in the brain serotonin satiety system in transgenic rats lacking brain angiotensinogen. J Neuroendocrinol 20: , 182–187. |
[9] | Nagano M , Takumi T , Suzuki H ((2018) ) Critical roles of serotonin-oxytocin interaction during the neonatal period in social behavior in 15q dup mice with autistic traits. Sci Rep 8: , 13675. |
[10] | Petersen CL , Davis SED , Patel B , Hurley LM ((2021) ) Social experience interacts with serotonin to affect functional connectivity in the social behavior network following playback of social vocalizations in mice. eNeuro 8: , ENEURO.0247-20.2021. |
[11] | Tian J , Stucky CS , Wang T , Muma NA , Johnson M , Du H ((2023) ) Mitochondrial dysfunction links to impaired hippocampal serotonin release in a mouse model of Alzheimer’s disease. J Alzheimers Dis 93: , 605–619. |
[12] | Aaldijk E , Vermeiren Y ((2022) ) The role of serotonin within the microbiota-gut-brain axis in the development of Alzheimer’s disease: A narrative review. Ageing Res Rev 75: , 101556. |
[13] | Elsworthy RJ , Aldred S ((2019) ) Depression in Alzheimer’s disease: An alternative role for selective serotonin reuptake inhibitors? J Alzheimers Dis 69: , 651–661. |
[14] | Xie Y , Liu PP , Lian YJ , Liu HB , Kang JS ((2019) ) The effect of selective serotonin reuptake inhibitors on cognitive function in patients with Alzheimer’s disease and vascular dementia: Focusing on fluoxetine with long follow-up periods. Signal Transduct Target Ther 4: , 30. |
[15] | Brendel M , Sauerbeck J , Greven S , Kotz S , Scheiwein F , Blautzik J , Delker A , Pogarell O , Ishii K , Bartenstein P , Rominger A , Alzheimer’s Disease Neuroimaging Initiative ((2018) ) Serotonin selective reuptake inhibitor treatment improves cognition and grey matter atrophy but not amyloid burden during two-year follow-up in mild cognitive impairment and Alzheimer’s disease patients with depressive symptoms. J Alzheimers Dis 65: , 793–806. |
[16] | Cumbo E , Cumbo S , Torregrossa S , Migliore D ((2019) ) Treatment effects of vortioxetine on cognitive functions in mild Alzheimer’s disease patients with depressive symptoms: A 12 month, open-label, observational study. J Prev Alzheimers Dis 6: , 192–197. |
[17] | Lopez-Domenech G , Kittler JT ((2023) ) Mitochondrial regulation of local supply of energy in neurons. Curr Opin Neurobiol 81: , 102747. |
[18] | Duarte FV , Ciampi D , Duarte CB ((2023) ) Mitochondria as central hubs in synaptic modulation. Cell Mol Life Sci 80: , 173. |
[19] | Pradeepkiran JA , Baig J , Selman A , Reddy PH (2023) Mitochondria in aging and Alzheimer’s disease: Focus on mitophagy. Neuroscientist, doi: 10.1177/10738584221139761. |
[20] | Swerdlow RH ((2018) ) Mitochondria and mitochondrial cascades in Alzheimer’s disease. J Alzheimers Dis 62: , 1403–1416. |
[21] | Guo L , Tian J , Du H ((2017) ) Mitochondrial dysfunction and synaptic transmission failure in Alzheimer’s disease. J Alzheimers Dis 57: , 1071–1086. |
[22] | Wang W , Zhao F , Ma X , Perry G , Zhu X ((2020) ) Mitochondria dysfunction in the pathogenesis of Alzheimer’s disease: Recent advances. Mol Neurodegener 15: , 30. |
[23] | Fanibunda SE , Deb S , Maniyadath B , Tiwari P , Ghai U , Gupta S , Figueiredo D , Weisstaub N , Gingrich JA , Vaidya ADB , Kolthur-Seetharam U , Vaidya VA ((2019) ) Serotonin regulates mitochondrial biogenesis and function in rodent cortical neurons via the 5-HT(2A) receptor and SIRT1-PGC-1alpha axis. Proc Natl Acad Sci U S A 116: , 11028–11037. |
[24] | Walther DJ , Peter JU , Bashammakh S , Hortnagl H , Voits M , Fink H , Bader M ((2003) ) Synthesis of serotonin by a second tryptophan hydroxylase isoform. Science 299: , 76. |
[25] | Nikishin DA , Alyoshina NM , Semenova ML , Shmukler YB ((2019) ) Analysis of expression and functional activity of aromatic L-amino acid decarboxylase (DDC) and serotonin transporter (SERT) as potential sources of serotonin in mouse ovary. Int J Mol Sci 20: , 3070. |
[26] | Eiden LE , Weihe E ((2011) ) VMAT2: A dynamic regulator of brain monoaminergic neuronal function interacting with drugs of abuse. Ann N Y Acad Sci 1216: , 86–98. |
[27] | Johnson PL , Molosh AI , Federici LM , Bernabe C , Haggerty D , Fitz SD , Nalivaiko E , Truitt W , Shekhar A ((2019) ) Assessment of fear and anxiety associated behaviors, physiology and neural circuits in rats with reduced serotonin transporter (SERT) levels. Transl Psychiatry 9: , 33. |
[28] | Choi IG , Kee BS , Son HG , Ham BJ , Yang BH , Kim SH , Lee JS , Son BK , Lee BY , Lee SY , Chai YG , Shin HD ((2006) ) Genetic polymorphisms of alcohol and aldehyde dehydrogenase, dopamine and serotonin transporters in familial and non-familial alcoholism. Eur Neuropsychopharmacol 16: , 123–128. |
[29] | Shader RI ((2023) ) Monoamine Oxidase Interactions. J Clin Psychopharmacol 43: , 393. |
[30] | Parsons SM ((2000) ) Transport mechanisms in acetylcholine and monoamine storage. FASEB J 14: , 2423–2434. |
[31] | Zhang YW , Turk BE , Rudnick G ((2016) ) Control of serotonin transporter phosphorylation by conformational state. Proc Natl Acad Sci U S A 113: , E2776–2783. |
[32] | Ramamoorthy S , Giovanetti E , Qian Y , Blakely RD ((1998) ) Phosphorylation and regulation of antidepressant-sensitive serotonin transporters. J Biol Chem 273: , 2458–2466. |
[33] | Li S , Sheng ZH ((2022) ) Energy matters: Presynaptic metabolism and the maintenance of synaptic transmission. Nat Rev Neurosci 23: , 4–22. |
[34] | Li S , Xiong GJ , Huang N , Sheng ZH ((2020) ) The cross-talk of energy sensing and mitochondrial anchoring sustains synaptic efficacy by maintaining presynaptic metabolism. Nat Metab 2: , 1077–1095. |
[35] | Pivovarov AS , Calahorro F , Walker RJ ((2018) ) Na(+)/K(+)-pump and neurotransmitter membrane receptors. Invert Neurosci 19: , 1. |
[36] | Du H , Guo L , Fang F , Chen D , Sosunov AA , McKhann GM , Yan Y , Wang C , Zhang H , Molkentin JD , Gunn-Moore FJ , Vonsattel JP , Arancio O , Chen JX , Yan SD ((2008) ) Cyclophilin D deficiency attenuates mitochondrial and neuronal perturbation and ameliorates learning and memory in Alzheimer’s disease. Nat Med 14: , 1097–1105. |
[37] | Ashrafi G , de Juan-Sanz J , Farrell RJ , Ryan TA ((2020) ) Molecular tuning of the axonal mitochondrial Ca(2+) uniporter ensures metabolic flexibility of neurotransmission. Neuron 105: , 678–687.e675. |
[38] | Brunk I , Blex C , Speidel D , Brose N , Ahnert-Hilger G ((2009) ) Ca2+-dependent activator proteins of secretion promote vesicular monoamine uptake. J Biol Chem 284: , 1050–1056. |
[39] | Vos M , Lauwers E , Verstreken P ((2010) ) Synaptic mitochondria in synaptic transmission and organization of vesicle pools in health and disease. Front Synaptic Neurosci 2: , 139. |
[40] | Kato TM , Kubota-Sakashita M , Fujimori-Tonou N , Saitow F , Fuke S , Masuda A , Itohara S , Suzuki H , Kato T ((2018) ) Ant1 mutant mice bridge the mitochondrial and serotonergic dysfunctions in bipolar disorder. Mol Psychiatry 23: , 2039–2049. |
[41] | Karch J , Bround MJ , Khalil H , Sargent MA , Latchman N , Terada N , Peixoto PM , Molkentin JD ((2019) ) Inhibition of mitochondrial permeability transition by deletion of the ANT family and CypD. Sci Adv 5: , eaaw4597. |
[42] | Lairez O , Calise D , Bianchi P , Ordener C , Spreux-Varoquaux O , Guilbeau-Frugier C , Escourrou G , Seif I , Roncalli J , Pizzinat N , Galinier M , Parini A , Mialet-Perez J ((2009) ) Genetic deletion of MAO-A promotes serotonin-dependent ventricular hypertrophy by pressure overload. J Mol Cell Cardiol 46: , 587–595. |
[43] | Helander A , Walzer C , Beck O , Balant L , Borg S , von Wartburg JP ((1994) ) Influence of genetic variation in alcohol and aldehyde dehydrogenase on serotonin metabolism. Life Sci 55: , 359–366. |
[44] | Brunner HG , Nelen M , Breakefield XO , Ropers HH , van Oost BA ((1993) ) Abnormal behavior associated with a point mutation in the structural gene for monoamine oxidase A. Science 262: , 578–580. |
[45] | Evrard A , Malagie I , Laporte AM , Boni C , Hanoun N , Trillat AC , Seif I , De Maeyer E , Gardier A , Hamon M , Adrien J ((2002) ) Altered regulation of the 5-HT system in the brain of MAO-A knock-out mice. Eur J Neurosci 15: , 841–851. |
[46] | Lajard AM , Bou C , Monteau R , Hilaire G ((1999) ) Serotonin levels are abnormally elevated in the fetus of the monoamine oxidase-A-deficient transgenic mouse. Neurosci Lett 261: , 41–44. |
[47] | Wiesbeck GA , Wodarz N , Weijers HG , Dursteler-MacFarland KM , Wurst FM , Walter M , Boening J ((2006) ) A functional polymorphism in the promoter region of the monoamine oxidase A gene is associated with the cigarette smoking quantity in alcohol-dependent heavy smokers. Neuropsychobiology 53: , 181–185. |
[48] | Gutierrez B , Arias B , Gasto C , Catalan R , Papiol S , Pintor L , Fananas L ((2004) ) Association analysis between a functional polymorphism in the monoamine oxidase A gene promoter and severe mood disorders. Psychiatr Genet 14: , 203–208. |
[49] | Huang YY , Cate SP , Battistuzzi C , Oquendo MA , Brent D , Mann JJ ((2004) ) An association between a functional polymorphism in the monoamine oxidase a gene promoter, impulsive traits and early abuse experiences. Neuropsychopharmacology 29: , 1498–1505. |
[50] | Lin CY , Yu RL , Wu RM , Tan CH ((2019) ) Effect of ALDH2 on sleep disturbances in patients with Parkinson’s disease. Sci Rep 9: , 18950. |
[51] | Jamal M , Ameno K , Miki T , Tanaka N , Ito A , Ono J , Takakura A , Kumihashi M , Kinoshita H ((2016) ) Ethanol and acetaldehyde differentially alter extracellular dopamine and serotonin in Aldh2-knockout mouse dorsal striatum: A reverse microdialysis study. Neurotoxicology 52: , 204–209. |
[52] | Fanibunda SE , Vaidya VA ((2021) ) Serotonin minting new mitochondria in cortical neurons: Implications for psychopathology. Neuropsychopharmacology 46: , 259–260. |
[53] | Yabut JM , Crane JD , Green AE , Keating DJ , Khan WI , Steinberg GR ((2019) ) Emerging roles for serotonin in regulating metabolism: New implications for an ancient molecule. Endocr Rev 40: , 1092–1107. |
[54] | Sola-Penna M , Paixao LP , Branco JR , Ochioni AC , Albanese JM , Mundim DM , Baptista-de-Souza D , Figueiredo CP , Coelho WS , Marcondes MC , Zancan P ((2020) ) Serotonin activates glycolysis and mitochondria biogenesis in human breast cancer cells through activation of the Jak1/STAT3/ERK1/2 and adenylate cyclase/PKA, respectively. Br J Cancer 122: , 194–208. |
[55] | Thorne BN , Ellenbroek BA , Day DJ ((2022) ) The serotonin reuptake transporter modulates mitochondrial copy number and mitochondrial respiratory complex gene expression in the frontal cortex and cerebellum in a sexually dimorphic manner. J Neurosci Res 100: , 869–879. |
[56] | Reddy AP , Yin X , Sawant N , Reddy PH ((2021) ) Protective effects of antidepressant citalopram against abnormal APP processing and amyloid beta-induced mitochondrial dynamics, biogenesis, mitophagy and synaptic toxicities in Alzheimer’s disease. Hum Mol Genet 30: , 847–864. |
[57] | Bertrand E , Dourado MC , Laks J , Morris RG , Landeira-Fernandez J , Mograbi DC ((2016) ) Mood-congruent recollection and anosognosia in Alzheimer’s disease. Cortex 84: , 55–62. |
[58] | Mitolo M , Tonon C , La Morgia C , Testa C , Carelli V , Lodi R ((2018) ) Effects of light treatment on sleep, cognition, mood, and behavior in Alzheimer’s disease: A systematic review. Dement Geriatr Cogn Disord 46: , 371–384. |
[59] | Martinez M , Multani N , Anor CJ , Misquitta K , Tang-Wai DF , Keren R , Fox S , Lang AE , Marras C , Tartaglia MC ((2018) ) Emotion detection deficits and decreased empathy in patients with Alzheimer’s disease and Parkinson’s disease affect caregiver mood and burden. Front Aging Neurosci 10: , 120. |
[60] | An H , Choi B , Park KW , Kim DH , Yang DW , Hong CH , Kim SY , Han SH ((2017) ) The Effect of escitalopram on mood and cognition in depressive Alzheimer’s disease subjects. J Alzheimers Dis 55: , 727–735. |
[61] | Padovani A , Antonini A , Barone P , Bellelli G , Fagiolini A , Ferini Strambi L , Sorbi S , Stocchi F ((2023) ) Exploring depression in Alzheimer’s disease: An Italian Delphi Consensus on phenomenology, diagnosis, and management. Neurol Sci 44: , 3123–3131. |
[62] | Novais F , Starkstein S ((2015) ) Phenomenology of depression in Alzheimer’s disease. J Alzheimers Dis 47: , 845–855. |
[63] | Starkstein SE , Mizrahi R , Power BD ((2008) ) Depression in Alzheimer’s disease: Phenomenology, clinical correlates and treatment. Int Rev Psychiatry 20: , 382–388. |
[64] | Swartz JR , Miller BL , Lesser IM , Booth R , Darby A , Wohl M , Benson DF ((1997) ) Behavioral phenomenology in Alzheimer’s disease, frontotemporal dementia, and late-life depression: A retrospective analysis. J Geriatr Psychiatry Neurol 10: , 67–74. |
[65] | Sanchez-Nieto D , Castano-Castano S , Navarro-Martos R , Obrero-Gaitan E , Cortes-Perez I , Nieto-Escamez F ((2023) ) An intervention on anxiety symptoms in moderate Alzheimer’s disease through virtual reality: A feasibility study and lessons learned. Int J Environ Res Public Health 20: , 2727. |
[66] | Demnitz-King H , Saba L , Lau Y , Munns L , Zabihi S , Schlosser M , Del-Pino-Casado R , Orgeta V , Marchant NL ((2023) ) Association between anxiety symptoms and Alzheimer’s disease biomarkers in cognitively healthy adults: A systematic review and meta-analysis. J Psychosom Res 166: , 111159. |
[67] | Cacabelos R , Carril JC , Corzo L , Pego R , Cacabelos N , Alcaraz M , Muniz A , Martinez-Iglesias O , Naidoo V ((2023) ) Pharmacogenetics of anxiety and depression in Alzheimer’s disease. Pharmacogenomics 24: , 27–57. |
[68] | Botto R , Callai N , Cermelli A , Causarano L , Rainero I ((2022) ) Anxiety and depression in Alzheimer’s disease: A systematic review of pathogenetic mechanisms and relation to cognitive decline. Neurol Sci 43: , 4107–4124. |
[69] | Johansson M , Stomrud E , Johansson PM , Svenningsson A , Palmqvist S , Janelidze S , van Westen D , Mattsson-Carlgren N , Hansson O ((2022) ) Development of apathy, anxiety, and depression in cognitively unimpaired older adults: Effects of Alzheimer’s disease pathology and cognitive decline. Biol Psychiatry 92: , 34–43. |
[70] | Patel P , Masurkar AV ((2021) ) The relationship of anxiety with Alzheimer’s disease: A narrative review. Curr Alzheimer Res 18: , 359–371. |
[71] | Zhao QF , Tan L , Wang HF , Jiang T , Tan MS , Tan L , Xu W , Li JQ , Wang J , Lai TJ , Yu JT ((2016) ) The prevalence of neuropsychiatric symptoms in Alzheimer’s disease: Systematic review and meta-analysis. J Affect Disord 190: , 264–271. |
[72] | Chen P , Guarino PD , Dysken MW , Pallaki M , Asthana S , Llorente MD , Love S , Vertrees JE , Schellenberg GD , Sano M ((2018) ) Neuropsychiatric symptoms and caregiver burden in individuals with Alzheimer’s disease: The TEAM-AD VA Cooperative Study. J Geriatr Psychiatry Neurol 31: , 177–185. |
[73] | Asmer MS , Kirkham J , Newton H , Ismail Z , Elbayoumi H , Leung RH , Seitz DP ((2018) ) Meta-analysis of the prevalence of major depressive disorder among older adults with dementia. J Clin Psychiatry 79: , 17r11772. |
[74] | Yang H , Hong W , Chen L , Tao Y , Peng Z , Zhou H ((2020) ) Analysis of risk factors for depression in Alzheimer’s disease patients. Int J Neurosci 130: , 1136–1141. |
[75] | Chan C , Rosenberg PB ((2019) ) Depression synergy with amyloid and increased risk of cognitive decline in preclinical Alzheimer disease. JAMA Netw Open 2: , e198970. |
[76] | Underwood EA , Davidson HP , Azam AB , Tierney MC ((2019) ) Sex differences in depression as a risk factor for Alzheimer’s disease: A systematic review. Innov Aging 3: , igz015. |
[77] | Ni H , Xu M , Zhan GL , Fan Y , Zhou H , Jiang HY , Lu WH , Tan L , Zhang DF , Yao YG , Zhang C ((2018) ) The GWAS risk genes for depression may be actively involved in Alzheimer’s disease. J Alzheimers Dis 64: , 1149–1161. |
[78] | Burke SL , Cadet T , Alcide A , O’Driscoll J , Maramaldi P ((2018) ) Psychosocial risk factors and Alzheimer’s disease: The associative effect of depression, sleep disturbance, and anxiety. Aging Ment Health 22: , 1577–1584. |
[79] | Vilalta-Franch J , Lopez-Pousa S , Llinas-Regla J , Calvo-Perxas L , Merino-Aguado J , Garre-Olmo J ((2013) ) Depression subtypes and 5-year risk of dementia and Alzheimer disease in patients aged 70 years. Int J Geriatr Psychiatry 28: , 341–350. |
[80] | Albert PR , Vahid-Ansari F , Luckhart C ((2014) ) Serotonin-prefrontal cortical circuitry in anxiety and depression phenotypes: Pivotal role of pre- and post-synaptic 5-HT1A receptor expression. Front Behav Neurosci 8: , 199. |
[81] | Deakin JF ((1998) ) The role of serotonin in panic, anxiety and depression. Int Clin Psychopharmacol 13 Suppl 4: , S1–5. |
[82] | Deakin J ((1998) ) The role of serotonin in depression and anxiety. Eur Psychiatry 13 Suppl 2: , 57s–63s. |
[83] | Baldwin D , Rudge S ((1995) ) The role of serotonin in depression and anxiety. Int Clin Psychopharmacol 9 Suppl 4: , 41–45. |
[84] | Chakraborty S , Lennon JC , Malkaram SA , Zeng Y , Fisher DW , Dong H ((2019) ) Serotonergic system, cognition, and BPSD in Alzheimer’s disease. Neurosci Lett 704: , 36–44. |
[85] | Smith GS , Barrett FS , Joo JH , Nassery N , Savonenko A , Sodums DJ , Marano CM , Munro CA , Brandt J , Kraut MA , Zhou Y , Wong DF , Workman CI ((2017) ) Molecular imaging of serotonin degeneration in mild cognitive impairment. Neurobiol Dis 105: , 33–41. |
[86] | Cross AJ ((1990) ) Serotonin in Alzheimer-type dementia and other dementing illnesses. Ann N Y Acad Sci 600: , 405–415; discussion 415-407. |
[87] | Palmer AM , Francis PT , Benton JS , Sims NR , Mann DM , Neary D , Snowden JS , Bowen DM ((1987) ) Presynaptic serotonergic dysfunction in patients with Alzheimer’s disease. J Neurochem 48: , 8–15. |
[88] | Francis PT , Palmer AM , Sims NR , Bowen DM , Davison AN , Esiri MM , Neary D , Snowden JS , Wilcock GK ((1985) ) Neurochemical studies of early-onset Alzheimer’s disease. Possible influence on treatment. N Engl J Med 313: , 7–11. |
[89] | Garcia-Alloza M , Gil-Bea FJ , Diez-Ariza M , Chen CP , Francis PT , Lasheras B , Ramirez MJ ((2005) ) Cholinergic-serotonergic imbalance contributes to cognitive and behavioral symptoms in Alzheimer’s disease. Neuropsychologia 43: , 442–449. |
[90] | Reinikainen KJ , Soininen H , Riekkinen PJ ((1990) ) Neurotransmitter changes in Alzheimer’s disease: Implications to diagnostics and therapy. J Neurosci Res 27: , 576–586. |
[91] | Bowen DM , Allen SJ , Benton JS , Goodhardt MJ , Haan EA , Palmer AM , Sims NR , Smith CC , Spillane JA , Esiri MM , Neary D , Snowdon JS , Wilcock GK , Davison AN ((1983) ) Biochemical assessment of serotonergic and cholinergic dysfunction and cerebral atrophy in Alzheimer’s disease. J Neurochem 41: , 266–272. |
[92] | Khan AF , Adewale Q , Baumeister TR , Carbonell F , Zilles K , Palomero-Gallagher N , Iturria-Medina Y , Alzheimer’s Disease Neuroimaging Initiative ((2022) ) Personalized brain models identify neurotransmitter receptor changes in Alzheimer’s disease. Brain 145: , 1785–1804. |
[93] | Hendricksen M , Thomas AJ , Ferrier IN , Ince P , O’Brien JT ((2004) ) Neuropathological study of the dorsal raphe nuclei in late-life depression and Alzheimer’s disease with and without depression. Am J Psychiatry 161: , 1096–1102. |
[94] | Simic G , Stanic G , Mladinov M , Jovanov-Milosevic N , Kostovic I , Hof PR ((2009) ) Does Alzheimer’s disease begin in the brainstem? Neuropathol Appl Neurobiol 35: , 532–554. |
[95] | Ji X , Wang H , Zhu M , He Y , Zhang H , Chen X , Gao W , Fu Y , Alzheimer’s Disease Neuroimaging Initiative ((2021) ) Brainstem atrophy in the early stage of Alzheimer’s disease: A voxel-based morphometry study. Brain Imaging Behav 15: , 49–59. |
[96] | Yamamoto T , Hirano A ((1985) ) Nucleus raphe dorsalis in Alzheimer’s disease: Neurofibrillary tangles and loss of large neurons. Ann Neurol 17: , 573–577. |
[97] | Curcio CA , Kemper T ((1984) ) Nucleus raphe dorsalis in dementia of the Alzheimer type: Neurofibrillary changes and neuronal packing density. J Neuropathol Exp Neurol 43: , 359–368. |
[98] | Smith GS , Protas H , Kuwabara H , Savonenko A , Nassery N , Gould NF , Kraut M , Avramopoulos D , Holt D , Dannals RF , Nandi A , Su Y , Reiman EM , Chen K ((2023) ) Molecular imaging of the association between serotonin degeneration and beta-amyloid deposition in mild cognitive impairment. Neuroimage Clin 37: , 103322. |
[99] | Liu Y , Yoo MJ , Savonenko A , Stirling W , Price DL , Borchelt DR , Mamounas L , Lyons WE , Blue ME , Lee MK ((2008) ) Amyloid pathology is associated with progressive monoaminergic neurodegeneration in a transgenic mouse model of Alzheimer’s disease. J Neurosci 28: , 13805–13814. |
[100] | Bengoa-Vergniory N , Velentza-Almpani E , Silva AM , Scott C , Vargas-Caballero M , Sastre M , Wade-Martins R , Alegre-Abarrategui J ((2021) ) Tau-proximity ligation assay reveals extensive previously undetected pathology prior to neurofibrillary tangles in preclinical Alzheimer’s disease. Acta Neuropathol Commun 9: , 18. |
[101] | Ehrenberg AJ , Nguy AK , Theofilas P , Dunlop S , Suemoto CK , Di Lorenzo Alho AT , Leite RP , Diehl Rodriguez R , Mejia MB , Rub U , Farfel JM , de Lucena Ferretti-Rebustini RE , Nascimento CF , Nitrini R , Pasquallucci CA , Jacob-Filho W , Miller B , Seeley WW , Heinsen H , Grinberg LT ((2017) ) Quantifying the accretion of hyperphosphorylated tau in the locus coeruleus and dorsal raphe nucleus: The pathological building blocks of early Alzheimer’s disease. Neuropathol Appl Neurobiol 43: , 393–408. |
[102] | Uematsu M , Nakamura A , Ebashi M , Hirokawa K , Takahashi R , Uchihara T ((2018) ) Brainstem tau pathology in Alzheimer’s disease is characterized by increase of three repeat tau and independent of amyloid beta. Acta Neuropathol Commun 6: , 1. |
[103] | Grinberg LT , Rub U , Ferretti RE , Nitrini R , Farfel JM , Polichiso L , Gierga K , Jacob-Filho W , Heinsen H , Brazilian Brain Bank Study G ((2009) ) The dorsal raphe nucleus shows phospho-tau neurofibrillary changes before the transentorhinal region in Alzheimer’s disease. A precocious onset? Neuropathol Appl Neurobiol 35: , 406–416. |
[104] | Eser RA , Ehrenberg AJ , Petersen C , Dunlop S , Mejia MB , Suemoto CK , Walsh CM , Rajana H , Oh J , Theofilas P , Seeley WW , Miller BL , Neylan TC , Heinsen H , Grinberg LT ((2018) ) Selective vulnerability of brainstem nuclei in distinct tauopathies: A postmortem study. J Neuropathol Exp Neurol 77: , 149–161. |
[105] | Halliday GM , McCann HL , Pamphlett R , Brooks WS , Creasey H , McCusker E , Cotton RG , Broe GA , Harper CG ((1992) ) Brain stem serotonin-synthesizing neurons in Alzheimer’s disease: A clinicopathological correlation. Acta Neuropathol 84: , 638–650. |
[106] | Khan KM , Balasubramanian N , Gaudencio G , Wang R , Selvakumar GP , Kolling L , Pierson S , Tadinada SM , Abel T , Hefti M , Marcinkiewcz CA ((2023) ) Human tau-overexpressing mice recapitulate brainstem involvement and neuropsychiatric features of early Alzheimer’s disease. Acta Neuropathol Commun 11: , 57. |
[107] | Lopes S , Vaz-Silva J , Pinto V , Dalla C , Kokras N , Bedenk B , Mack N , Czisch M , Almeida OF , Sousa N , Sotiropoulos I ((2016) ) Tau protein is essential for stress-induced brain pathology. Proc Natl Acad Sci U S A 113: , E3755–3763. |
[108] | Labus J , Rohrs KF , Ackmann J , Varbanov H , Muller FE , Jia S , Jahreis K , Vollbrecht AL , Butzlaff M , Schill Y , Guseva D , Bohm K , Kaushik R , Bijata M , Marin P , Chaumont-Dubel S , Zeug A , Dityatev A , Ponimaskin E ((2021) ) Amelioration of tau pathology and memory deficits by targeting 5-HT7 receptor. Prog Neurobiol 197: , 101900. |
[109] | John NJ , Lew GM , Goya L , Timiras PS ((1991) ) Effects of serotonin on tyrosine hydroxylase and tau protein in a human neuroblastoma cell line. Adv Exp Med Biol 296: , 69–80. |
[110] | Noristani HN , Meadows RS , Olabarria M , Verkhratsky A , Rodriguez JJ ((2011) ) Increased hippocampal CA1 density of serotonergic terminals in a triple transgenic mouse model of Alzheimer’s disease: An ultrastructural study. Cell Death Dis 2: , e210. |
[111] | Sheline YI , West T , Yarasheski K , Swarm R , Jasielec MS , Fisher JR , Ficker WD , Yan P , Xiong C , Frederiksen C , Grzelak MV , Chott R , Bateman RJ , Morris JC , Mintun MA , Lee JM , Cirrito JR ((2014) ) An antidepressant decreases CSF Abeta production in healthy individuals and in transgenic AD mice. Sci Transl Med 6: , 236re234. |
[112] | Cirrito JR , Disabato BM , Restivo JL , Verges DK , Goebel WD , Sathyan A , Hayreh D , D’Angelo G , Benzinger T , Yoon H , Kim J , Morris JC , Mintun MA , Sheline YI ((2011) ) Serotonin signaling is associated with lower amyloid-beta levels and plaques in transgenic mice and humans. Proc Natl Acad Sci U S A 108: , 14968–14973. |
[113] | Cochet M , Donneger R , Cassier E , Gaven F , Lichtenthaler SF , Marin P , Bockaert J , Dumuis A , Claeysen S ((2013) ) 5-HT4 receptors constitutively promote the non-amyloidogenic pathway of APP cleavage and interact with ADAM10. ACS Chem Neurosci 4: , 130–140. |
[114] | Tesseur I , Pimenova AA , Lo AC , Ciesielska M , Lichtenthaler SF , De Maeyer JH , Schuurkes JA , D’Hooge R , De Strooper B ((2013) ) Chronic 5-HT4 receptor activation decreases Abeta production and deposition in hAPP/PS1 mice. Neurobiol Aging 34: , 1779–1789. |
[115] | Giannoni P , Gaven F , de Bundel D , Baranger K , Marchetti-Gauthier E , Roman FS , Valjent E , Marin P , Bockaert J , Rivera S , Claeysen S ((2013) ) Early administration of RS 67333, a specific 5-HT4 receptor agonist, prevents amyloidogenesis and behavioral deficits in the 5XFAD mouse model of Alzheimer’s disease. Front Aging Neurosci 5: , 96. |
[116] | Mowla A , Mosavinasab M , Haghshenas H , Borhani Haghighi A ((2007) ) Does serotonin augmentation have any effect on cognition and activities of daily living in Alzheimer’s dementia? A double-blind, placebo-controlled clinical trial. J Clin Psychopharmacol 27: , 484–487. |
[117] | Reddy AP , Sawant N , Morton H , Kshirsagar S , Bunquin LE , Yin X , Reddy PH ((2021) ) Selective serotonin reuptake inhibitor citalopram ameliorates cognitive decline and protects against amyloid beta-induced mitochondrial dynamics, biogenesis, autophagy, mitophagy and synaptic toxicities in a mouse model of Alzheimer’s disease. Hum Mol Genet 30: , 789–810. |
[118] | Khatoon R , Pahuja M , Parvez S ((2020) ) Cross talk between mitochondria and other targets in Alzheimer’s disease. J Environ Pathol Toxicol Oncol 39: , 261–279. |
[119] | Shevtsova EF , Maltsev AV , Vinogradova DV , Shevtsov PN , Bachurin SO ((2021) ) Mitochondria as a promising target for developing novel agents for treating Alzheimer’s disease. Med Res Rev 41: , 803–827. |
[120] | Yan R , Wang W , Yang W , Huang M , Xu W (2023) Mitochondria-related candidate genes and diagnostic model to predict late-onset Alzheimer’s disease and mild cognitive impairment. J Alzheimers Dis, doi: 10.3233/JAD-230314. |
[121] | Tian J , Guo L , Sui S , Driskill C , Phensy A , Wang Q , Gauba E , Zigman JM , Swerdlow RH , Kroener S , Du H ((2019) ) Disrupted hippocampal growth hormone secretagogue receptor 1alpha interaction with dopamine receptor D1 plays a role in Alzheimer’s disease. Sci Transl Med 11: , eaav6278. |
[122] | Rao YL , Ganaraja B , Murlimanju BV , Joy T , Krishnamurthy A , Agrawal A ((2022) ) Hippocampus and its involvement in Alzheimer’s disease: A review. 3 Biotech 12: , 55. |
[123] | Du Y , Zhang S , Fang Y , Qiu Q , Zhao L , Wei W , Tang Y , Li X ((2021) ) Radiomic features of the hippocampus for diagnosing early-onset and late-onset Alzheimer’s disease. Front Aging Neurosci 13: , 789099. |
[124] | Karayol R , Medrihan L , Warner-Schmidt JL , Fait BW , Rao MN , Holzner EB , Greengard P , Heintz N , Schmidt EF ((2021) ) Serotonin receptor 4 in the hippocampus modulates mood and anxiety. Mol Psychiatry 26: , 2334–2349. |
[125] | Qasim SE , Mohan UR , Stein JM , Jacobs J ((2023) ) Neuronal activity in the human amygdala and hippocampus enhances emotional memory encoding. Nat Hum Behav 7: , 754–764. |
[126] | Danieli K , Guyon A , Bethus I ((2023) ) Episodic memory formation: A review of complex Hippocampus input pathways. Prog Neuropsychopharmacol Biol Psychiatry 126: , 110757. |
[127] | Kirkby LA , Luongo FJ , Lee MB , Nahum M , Van Vleet TM , Rao VR , Dawes HE , Chang EF , Sohal VS ((2018) ) An amygdala-hippocampus subnetwork that encodes variation in human mood. Cell 175: , 1688–1700 e1614. |
[128] | Wang L , He Z , Zhu Z , Yuan W , Cai W , Li L , Zhang J , Hou W , Yang Y , Zhang X , Guo Q , Wang X , Lian Z , Tai F ((2019) ) The serotonin system in the hippocampus CA3 involves in effects of CSDS on social recognition in adult female mandarin voles (Microtus mandarinus). Prog Neuropsychopharmacol Biol Psychiatry 95: , 109704. |
[129] | Dale E , Pehrson AL , Jeyarajah T , Li Y , Leiser SC , Smagin G , Olsen CK , Sanchez C ((2016) ) Effects of serotonin in the hippocampus: How SSRIs and multimodal antidepressants might regulate pyramidal cell function. CNS Spectr 21: , 143–161. |
[130] | Heo S , Patil SS , Jung G , Hoger H , Lubec G ((2011) ) A serotonin receptor 1A containing complex in hippocampus of PWD/PhJ mice is linked to training effects in the Barnes maze. Behav Brain Res 216: , 389–395. |
[131] | Claustre Y , Benavides J , Scatton B ((1991) ) Potential mechanisms involved in the negative coupling between serotonin 5-HT1A receptors and carbachol-stimulated phosphoinositide turnover in the rat hippocampus. J Neurochem 56: , 1276–1285. |
[132] | Surowka P , Noworyta K , Smaga I , Filip M , Rygula R ((2023) ) Trait sensitivity to negative feedback in rats is associated with increased expression of serotonin 5-HT(2A) receptors in the ventral hippocampus. Front Mol Neurosci 16: , 1092864. |
[133] | Kennedy BP , Ziegler MG , Alford M , Hansen LA , Thal LJ , Masliah E ((2003) ) Early and persistent alterations in prefrontal cortex MAO A and B in Alzheimer’s disease. J Neural Transm (Vienna) 110: , 789–801. |
[134] | Emilsson L , Saetre P , Balciuniene J , Castensson A , Cairns N , Jazin EE ((2002) ) Increased monoamine oxidase messenger RNA expression levels in frontal cortex of Alzheimer’s disease patients. Neurosci Lett 326: , 56–60. |
[135] | Saura J , Luque JM , Cesura AM , Da Prada M , Chan-Palay V , Huber G , Loffler J , Richards JG ((1994) ) Increased monoamine oxidase B activity in plaque-associated astrocytes of Alzheimer brains revealed by quantitative enzyme radioautography. Neuroscience 62: , 15–30. |
[136] | Quartey MO , Nyarko JNK , Pennington PR , Heistad RM , Klassen PC , Baker GB , Mousseau DD ((2018) ) Alzheimer disease and selected risk factors disrupt a co-regulation of monoamine oxidase-A/B in the hippocampus, but not in the cortex. Front Neurosci 12: , 419. |
[137] | Kim D , Baik SH , Kang S , Cho SW , Bae J , Cha MY , Sailor MJ , Mook-Jung I , Ahn KH ((2016) ) Close correlation of monoamine oxidase activity with progress of Alzheimer’s disease in mice, observed by in vivo two-photon imaging. ACS Cent Sci 2: , 967–975. |
[138] | Weinreb O , Amit T , Bar-Am O , Youdim MB ((2012) ) Ladostigil: A novel multimodal neuroprotective drug with cholinesterase and brain-selective monoamine oxidase inhibitory activities for Alzheimer’s disease treatment. Curr Drug Targets 13: , 483–494. |
[139] | Weinreb O , Amit T , Bar-Am O , Youdim MB ((2011) ) A novel anti-Alzheimer’s disease drug, ladostigil neuroprotective, multimodal brain-selective monoamine oxidase and cholinesterase inhibitor. Int Rev Neurobiol 100: , 191–215. |
[140] | Cho HU , Kim S , Sim J , Yang S , An H , Nam MH , Jang DP , Lee CJ ((2021) ) Redefining differential roles of MAO-A in dopamine degradation and MAO-B in tonic GABA synthesis. Exp Mol Med 53: , 1148–1158. |
[141] | Westlund KN , Denney RM , Rose RM , Abell CW ((1988) ) Localization of distinct monoamine oxidase A and monoamine oxidase B cell populations in human brainstem. Neuroscience 25: , 439–456. |
[142] | Fagervall I , Ross SB ((1986) ) A and B forms of monoamine oxidase within the monoaminergic neurons of the rat brain. J Neurochem 47: , 569–576. |
[143] | Thorpe LW , Westlund KN , Kochersperger LM , Abell CW , Denney RM ((1987) ) Immunocytochemical localization of monoamine oxidases A and B in human peripheral tissues and brain. J Histochem Cytochem 35: , 23–32. |
[144] | Youdim MB , Riederer P ((1993) ) The relevance of glial monoamine oxidase-B and polyamines to the action of selegiline in Parkinson’s disease. Mov Disord 8 Suppl 1: , S8–13. |
[145] | Yoshimoto M , Hirata M , Kagawa S , Magata Y , Ohmomo Y , Temma T ((2019) ) Synthesis and characterization of novel radiofluorinated probes for positron emission tomography imaging of monoamine oxidase B. J Labelled Comp Radiopharm 62: , 580–587. |
[146] | Shih JC , Chen K , Ridd MJ ((1999) ) Role of MAO A and B in neurotransmitter metabolism and behavior. Pol J Pharmacol 51: , 25–29. |
[147] | Kalimon OJ , Vekaria HJ , Gerhardt GA , Sullivan PG ((2023) ) Inhibition of monoamine oxidase-a increases respiration in isolated mouse cortical mitochondria. Exp Neurol 363: , 114356. |
[148] | Yamaguchi N , Nakajima N , Okada S , Yuri K ((2016) ) Effects of aging on stress-related responses of serotonergic neurons in the dorsal raphe nucleus of male rats. Neurobiol Stress 3: , 43–51. |
[149] | Kloppel S , Kovacs GG , Voigtlander T , Wanschitz J , Flicker H , Hainfellner JA , Guentchev M , Budka H ((2001) ) Serotonergic nuclei of the raphe are not affected in human ageing. Neuroreport 12: , 669–671. |
[150] | Grimm A , Eckert A ((2017) ) Brain aging and neurodegeneration: From a mitochondrial point of view. J Neurochem 143: , 418–431. |
[151] | Rango M , Bresolin N ((2018) ) Brain mitochondria, aging, and Parkinson’s disease. Genes (Basel) 9: , 250. |
[152] | Ross CM ((2005) ) Folate, mitochondria, ROS, and the aging brain. Am J Med 118: , 1174; author reply 1174–1175. |
[153] | Bennett JP Jr., Onyango IG ((2021) ) Energy, entropy and quantum tunneling of protons and electrons in brain mitochondria: Relation to mitochondrial impairment in aging-related human brain diseases and therapeutic measures. Biomedicines 9: , 225. |
[154] | Swerdlow RH ((2011) ) Brain aging, Alzheimer’s disease, and mitochondria. Biochim Biophys Acta 1812: , 1630–1639. |
[155] | Wu H , Denna TH , Storkersen JN , Gerriets VA ((2019) ) Beyond a neurotransmitter: The role of serotonin in inflammation and immunity. Pharmacol Res 140: , 100–114. |
[156] | Metaxas A , Anzalone M , Vaitheeswaran R , Petersen S , Landau AM , Finsen B ((2019) ) Neuroinflammation and amyloid-beta 40 are associated with reduced serotonin transporter (SERT) activity in a transgenic model of familial Alzheimer’s disease. Alzheimers Res Ther 11: , 38. |
[157] | Correia AS , Cardoso A , Vale N ((2023) ) Oxidative stress in depression: The link with the stress response, neuroinflammation, serotonin, neurogenesis and synaptic plasticity. Antioxidants (Basel) 12: , 470. |
[158] | Harland M , Torres S , Liu J , Wang X ((2020) ) Neuronal mitochondria modulation of LPS-induced neuroinflammation. J Neurosci 40: , 1756–1765. |
[159] | Wu J , Han C , Cao X , Lv Z , Wang C , Huo X , Feng L , Zhang B , Tian X , Ma X ((2022) ) Mitochondria targeting fluorescent probe for MAO-A and the application in the development of drug candidate for neuroinflammation. Anal Chim Acta 1199: , 339573. |
[160] | Obrador E , Salvador R , Lopez-Blanch R , Jihad-Jebbar A , Valles SL , Estrela JM ((2020) ) Oxidative stress, neuroinflammation and mitochondria in the pathophysiology of amyotrophic lateral sclerosis. Antioxidants (Basel) 9: , 901. |
[161] | Pan X , Kaminga AC , Wen SW , Wu X , Acheampong K , Liu A ((2019) ) Dopamine and dopamine receptors in Alzheimer’s disease: A systematic review and network meta-analysis. Front Aging Neurosci 11: , 175. |
[162] | D’Amelio M , Puglisi-Allegra S , Mercuri N ((2018) ) The role of dopaminergic midbrain in Alzheimer’s disease: Translating basic science into clinical practice. Pharmacol Res 130: , 414–419. |
[163] | Hampel H , Mesulam MM , Cuello AC , Farlow MR , Giacobini E , Grossberg GT , Khachaturian AS , Vergallo A , Cavedo E , Snyder PJ , Khachaturian ZS ((2018) ) The cholinergic system in the pathophysiology and treatment of Alzheimer’s disease. Brain 141: , 1917–1933. |
[164] | Lesser IM , Myers HF , Lin KM , Bingham Mira C , Joseph NT , Olmos NT , Schettino J , Poland RE ((2010) ) Ethnic differences in antidepressant response: A prospective multi-site clinical trial. Depress Anxiety 27: , 56–62. |
[165] | Knudsen HK , Ducharme LJ , Roman PM ((2007) ) Racial and ethnic disparities in SSRI availability in substance abuse treatment. Psychiatr Serv 58: , 55–62. |
[166] | Zivin K , Pfeiffer PN , Bohnert AS , Ganoczy D , Blow FC , Nallamothu BK , Kales HC ((2014) ) Safety of high-dosage citalopram. Am J Psychiatry 171: , 20–22. |
[167] | Crocco EA , Jaramillo S , Cruz-Ortiz C , Camfield K ((2017) ) Pharmacological management of anxiety disorders in the elderly. Curr Treat Options Psychiatry 4: , 33–46. |