A Potential Role for Sirtuin-1 in Alzheimer’s Disease: Reviewing the Biological and Environmental Evidence
Abstract
Sirtuin-1 (Sirt1), encoded by the SIRT1 gene, is a conserved Nicotinamide adenine dinucleotide (NAD+) dependent deacetylase enzyme, considered as the master regulator of metabolism in humans. Sirt1 contributes to a wide range of biological pathways via several mechanisms influenced by lifestyle, such as diet and exercise. The importance of a healthy lifestyle is of relevance to highly prevalent modern chronic diseases, such as Alzheimer’s disease (AD). There is growing evidence at multiple levels for a role of Sirt1/SIRT1 in AD pathological mechanisms. As such, this review will explore the relevance of Sirt1 to AD pathological mechanisms, by describing the involvement of Sirt1/SIRT1 in the development of AD pathological hallmarks, through its impact on the metabolism of amyloid-β and degradation of phosphorylated tau. We then explore the involvement of Sirt1/SIRT1 across different AD-relevant biological processes, including cholesterol metabolism, inflammation, circadian rhythm, and gut microbiome, before discussing the interplay between Sirt1 and AD-related lifestyle factors, such as diet, physical activity, and smoking, as well as depression, a common comorbidity. Genome-wide association studies have explored potential associations between SIRT1 and AD, as well as AD risk factors and co-morbidities. We summarize this evidence at the genetic level to highlight links between SIRT1 and AD, particularly associations with AD-related risk factors, such as heart disease. Finally, we review the current literature of potential interactions between SIRT1 genetic variants and lifestyle factors and how this evidence supports the need for further research to determine the relevance of these interactions with respect to AD and dementia.
INTRODUCTION
Silent information regulator 1 (Sirt1) protein, a member of the sirtuin family of class III histone deacetylases, has been found to contribute to a wide range of biological regulatory pathways via its role in epigenetic regulation (e.g., acetylation and methylation) of genes related to cell growth, inflammation, apoptosis, mitochondrial biogenesis, neurobiological processes, cell senescence, and longevity [1]. Additionally, an age-related reduction in serum Sirt1 protein in multiple tissues seems to be involved in age-related disorders [2], including Alzheimer’s disease (AD).
AD, the most common neurodegenerative cause of dementia, is clinically defined by loss of short-term memory, impaired judgement and problem solving, and changes in behavior and mood [3]. It is characterized by the accumulation of neurofibrillary tangles (NFTs), composed of hyperphosphorylated tau protein, and extracellular amyloid-β (Aβ) plaques, in the brain. Moreover, significant neuronal loss in the hippocampus and entorhinal cortex with substantial gliosis has been demonstrated. The onset of these pathological features of AD can occur up to two decades before symptoms appear [4]. Early-stage detection of AD is difficult and there is a lack of effective treatment, meaning individuals living with the condition often require a long period of nursing care, resulting in substantial economic burden [5]. As such, improved early detection and an increased understanding of risk factors at preclinical stages is critical for effective prevention and treatment.
There is a large body of literature describing the role of genetics and environmental/lifestyle factors as modulators of the pathological mechanisms of AD and dementia. Recently, much research has focused on the potential interaction between these factors. Environmental factors, and in particular lifestyle factors, are often modifiable, for example, through changes to diet, physical activity, and other behaviors. Therefore, understanding how their effect on AD interacts with an individual’s genetic background has the potential to enable individualized lifestyle interventions, which may provide more effective prevention or treatment [6–8]. In the current review (schematic summary Fig. 1), we will first briefly introduce the Sirtuins, particularly Sirt1 and preliminary evidence to support its relevance to AD. We then discuss the current evidence linking Sirt1 with AD in more detail, including AD-linked pathological mechanisms and biological processes associated with AD. In the case of biological processes, this association with AD is either through genetic studies or through relevance to the disease. Next, we will review what links several lifestyle factors, of relevance to AD, to Sirt1/SIRT1, before discussing the current literature linking genetic variation within the SIRT1 gene with AD and different AD-related risk factors and co-morbidities. Finally, we summarize evidence for the potential interaction between SIRT1 genetic variants and lifestyle factors, with reference to chronic diseases considered as potential risk factors for dementia and AD.
Fig. 1
Potential interactions between Sirt1/SIRT1, lifestyle factors, comorbidities, biological pathways, and Alzheimer’s disease. Aβ, amyloid-β.
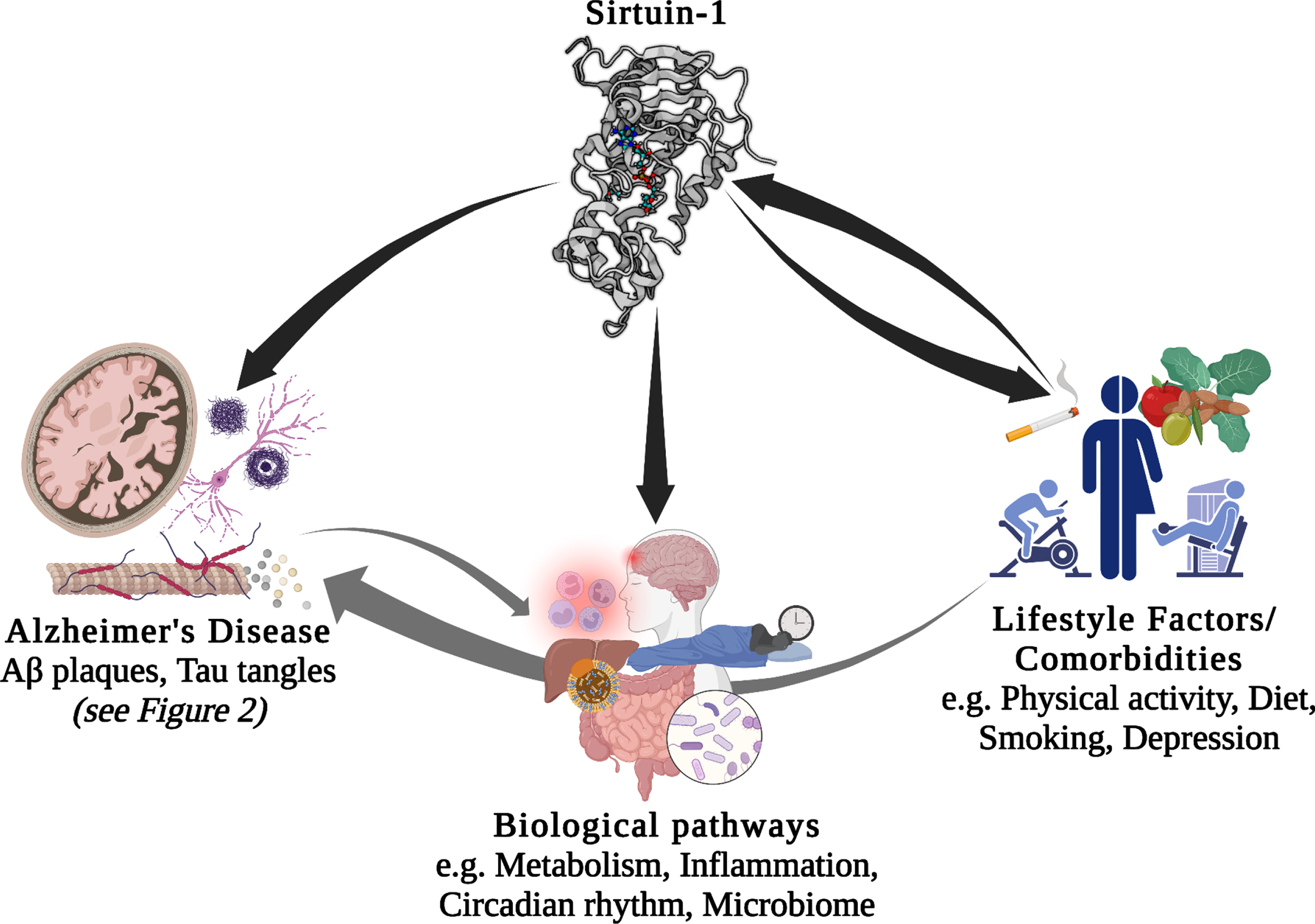
THE SIRTUINS AND SIRT1
Proteins in the sirtuin family are promising targets for improving aging phenotypes and ameliorating age-related diseases [9]. Seven members of the Sirtuin family of enzymes, are nicotinamide adenine dinucleotide (NAD)+-dependent class III histone deacetylases, and contribute to post-translational modifications (PTMs) of both nucleic and cytoplasmic proteins. Sirtuins remove acetyl groups from lysines through NAD; therefore, they contribute to a wide range of physiological processes, including regulating gene expression, metabolism, and senescence [1]. Sirt 6 and 7 are located in the nucleus, Sirt 2 in the cytoplasm, and Sirt 3–5 are in mitochondria. Sirt1 is found in both the nucleus and cytoplasm [10].
Among sirtuins, Sirt1 is the most studied and is expressed in adult brain, where it is predominantly found in neuronal nuclei [11]. Sirt1 can catalyze deacetylation of site-specific lysine residues of both histone and non-histone proteins, providing a potential connection between epigenetics, metabolism, and health status. Specifically, the NAD+/NADH ratio is a potential link between metabolism and epigenetic regulation of gene expression by contributing to histone acetylation [12]. Histone acetylation is an important epigenetic mechanism that alters chromatin structure and modulates gene expression by opening or closing the chromatin, playing a significant role in cell cycle and cell differentiation [13]. In culture media, SIRT1 overexpression improves dendritic spine density, neurite outgrowth and survival, and influences plasticity via brain-derived neurotrophic factor (BDNF) [14]. Neuroprotectivity of Sirt1 seems to be mediated by acetylation of neuronal p53, which suppresses induction of apoptosis [14]. Further, it has been suggested that Sirt1 is deployed to DNA damage sites to repair DNA, decreasing its activity in other loci of the genome and leading to “transcriptional noise”, which is a hallmark of cellular senescence [15]. Moreover, the activity of Sirt1 enzymes decreases with age [2], making it a good candidate biomarker for the study of age-related diseases, including AD [16].
SIRT1 AND ALZHEIMER’S DISEASE
AD pathological mechanisms
The contribution of Sirt1 to AD pathological mechanisms has been proposed in several studies. Sirt1 plays important roles in both Aβ and tau metabolism through various biological mechanisms (Fig. 2). It has been suggested to modulate the key enzymes/enzyme complexes integral to the metabolism of the amyloid-β protein precursor (AβPP), namely beta-secretase 1 (BACE1) and α-secretase (ADAM10, A Disintegrin and metalloproteinase domain-containing protein 10) [9], and thus may play an important role in regulating Aβ production. Studies of AD cases showed that in brain regions with Aβ accumulation, BACE1, which is the rate-limiting enzyme in Aβ metabolism is overexpressed [17]. Expression of BACE1 has been suggested to be regulated by peroxisome proliferator-activated receptor gamma (PPARγ), and its coactivator (PGC-1α), which when deacetylated by Sirt1, reduces BACE1 expression [17]. Moreover, ADAM10 cleaves AβPP to produce soluble AβPP (sAβPP), a neuroprotective fragment. The upregulation of ADAM10, through the deacetylation of retinoic acid receptor beta (RARβ) by Sirt1, could potentially protect the brain from Aβ accumulation through the potentiating the non-amyloidogenic cleavage pathway of AβPP and, in turn through increasing sAβPP, provides a neuroprotective mechanism against AD [18]. This is further supported by a photobiomodulation therapy study that showed that the activation of Sirt1 was responsible for the induced effects of reduced Aβ production, with knocking down of SIRT1 resulting in an antagonistic effect [19]. Taken together, there is growing evidence to support the key-role of Sirt1 in influencing the function of ADAM10 in the context of AD.
Fig. 2
Potential associations between Sirt1 and Alzheimer’s disease hallmark pathological features. Suggested roles that Sirt1-mediated deacetylation plays in the development of the pathological hallmarks of Alzheimer’s disease, including the development of Aβ plaques and neurofibrillary tangles, through the modulation of AβPP cleavage pathways and tau degradation. Aβ, amyloid-β; AβPP, amyloid-β protein precursor; Ac, acetyl-; ADAM10, A Disintegrin and metalloproteinase domain-containing protein 10/α-secretase; BACE1, beta-secretase 1; NAD+, Nicotinamide adenine dinucleotide; NADH, reduced nicotinamide adenine dinucleotide; PPARγ, Peroxisome proliferator-activated receptor-gamma; PGC-1α, peroxisome proliferator-activated receptor-gamma coactivator 1α; p, phospho-; RARβ, Retinoic acid receptor beta; Ub, ubiquitin.
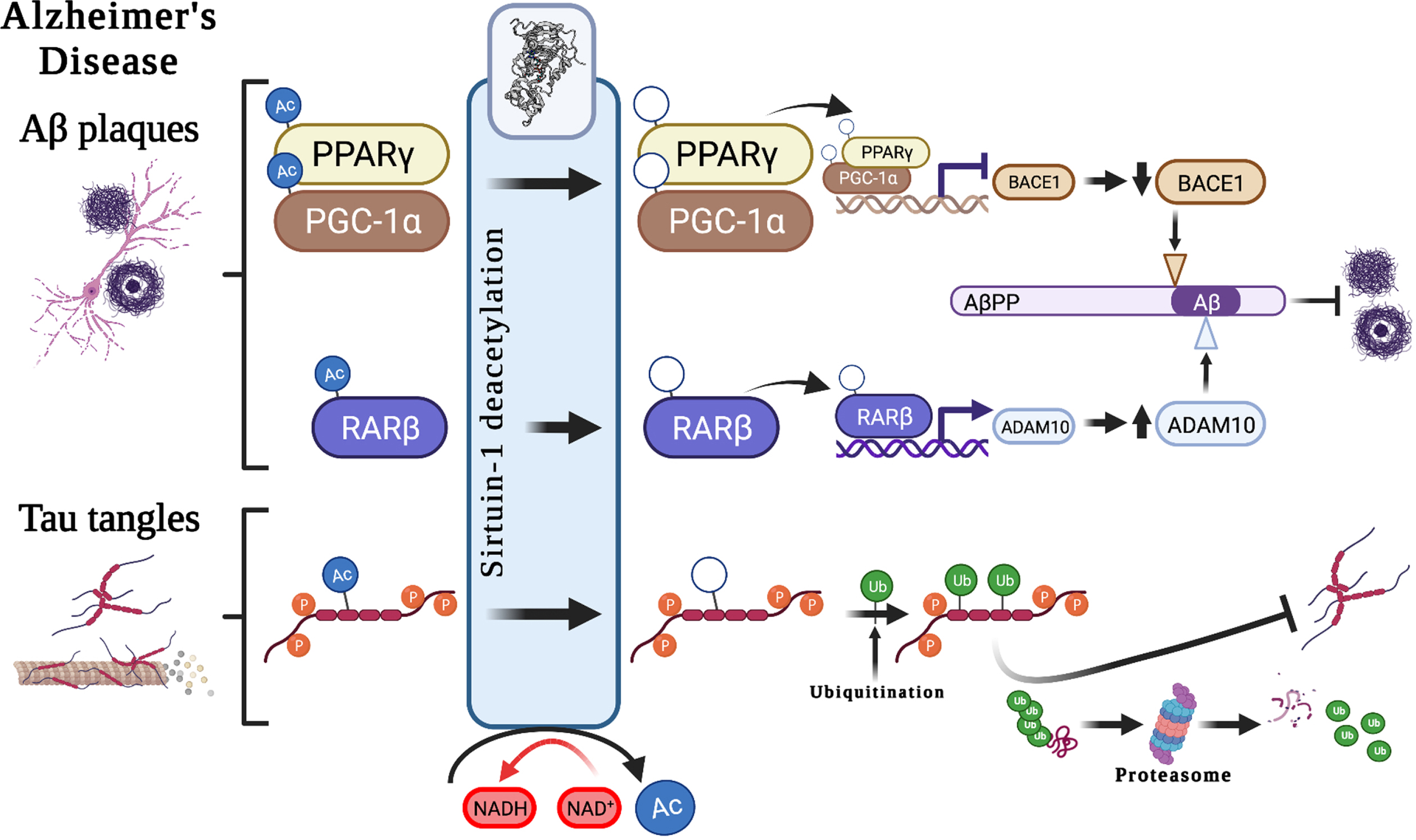
Sirt1 also has autophagy-enhancing effects, thereby contributing to Aβ degradation [20]. In mammals, polyubiquitination can occur through autophagy–lysosomal pathways at lysine residues, interfering with acetylation. It is through this mechanism that decreased Sirt1 levels may result in hyperacetylation of substrates, such as phosphorylated tau [21]. Acetylated tau (ac-tau) has been reported to be increased in individuals with early and moderate Braak stages of tauopathy (a postmortem method for staging of AD progression based on anatomical localization of pathological features [22]), at earlier stages than the formation of NFTs. Moreover, Sirt1 has been shown to remove acetyl groups from tau protein, and an in vivo study showed Sirt1 deficiency increased ac-tau levels. As such, the inhibition of Sirt1, or the decline in Sirt1 enzyme activity observed with age [2], may result in a reduction in the deacetylation of tau, which in turn might block tau polyubiquitination and tau proteasomal degradation and lead to p-tau accumulation and, finally, the formation of NFTs [21].
Evidence for the importance of Sirt1 in memory and learning, the cognitive processes that are impaired in AD, is also emerging. Spatial learning and both short-term and long-term memory were disrupted n male mice, aged 5-6 months, with a brain-specific SIRT1 gene knockout [23, 24]. Although the brain for these mice had normal morphology and dendritic spine structure they showed a decrease in dendritic branching, branch length, and complexity of neuronal dendritic arbors. Also, there was downregulation of hippocampal genes contributing to synaptic function, lipid metabolism, and myelination. These findings provide evidence to support the important role of Sirt1 in neuronal development and plasticity. Moreover, epigenetic mechanisms such as DNA methylation contribute to memory formation and maintenance suggesting, Sirt1 may make a significant contribution through its role as an epigenetic “coordinator” [25].
Genetically linked AD biological pathways
Genome-wide association studies (GWAS) and analyses of gene expression data have identified multiple pathways associated with AD. Four such pathways include those responsible for cholesterol metabolism, immune response, regulation of endocytosis, and protein ubiquitination. Sirt1 is also known to modulate these biological pathways, particularly through deacetylation of many non-histone proteins, such as transcriptional nuclear receptors, that are involved in metabolism, inflammation, and apoptosis, and potentially age-related diseases such as AD [26]. The transcriptional regulatory proteins with the best evidence for modulation by Sirt 1 are PGC-1α, NF-κB (Nuclear factor kappa-light-chain-enhancer of activated B cells), p53 (tumor protein P53), FOXOs (Forkhead box O transcription factors), CRTC2 (CREB (cAMP response element-binding protein) Regulated Transcription Coactivator 2, LXRs (liver X receptors), FXR (farnesoid X receptor), and SREBPs (Sterol regulatory-element binding proteins) [27]. Here we review experimental evidence for Sirt1/SIRT1 activities in two key pathways regulating metabolism (specifically cholesterol metabolism) and inflammation, which we hypothesize may link Sirt1 to AD.
Sirt1 and metabolism
The deacetylation activity of Sirt1 links the enzyme to protein substrates, such as NAD [28, 29], an important coenzyme in all living cells. Through electron transfer, switching from an oxidizing form [NAD+] to a reducing form [NADH] and vice versa [29], it is involved in numerous metabolism pathways, including being a necessary co-enzyme for Sirt1 activity. Sirt1 regulates and controls many key transcription factors and co-factors involving in metabolic homeostasis; due to this significant downstream effects, Sirt1 is increasingly considered as a master regulator for metabolism [30]. Accordingly, Sirt1/SIRT1 has been widely investigated in the field of metabolism in different key organs and tissues including the liver, pancreas, muscle, adipose tissue (see Table 1), and brain. Specifically, SIRT1 is expressed in the hypothalamus, where it is distributed in the ventromedial, arcuate, paraventricular and dorsomedial nuclei, not only supporting its critical role in metabolic regulation in the brain [31] but also more broadly links Sirt1 to the hypothalamic–pituitary–gonadal and -adrenal axes, and thermoregulation, circadian rhythms and satiety, several of which we discuss later. Generally, disruption in energy metabolism is common among AD population such as eating behaviors [32]. Brain Aβ plaques and NFTs are common in AD’s hypothalamus and metabolic disorders such as obesity, insulin resistant, and diabetes worsen Aβ accumulation and tau tangles [33–35]. Unsurprisingly, Sirt1 is closely related to metabolic disorders including T2DM, obesity, dyslipidemia, and fatty liver disease [30]; all of which increase dementia risk.
Table 1
The contribution of Sirt1 to metabolism in different organs and tissues
Organ or Tissue | Biological effect(s) | Target molecule(s) |
Brain | Regulation of food intake [180] | FOXO1, NF-κB |
Leptin signals [180] | ||
Energy expenditure in POMC neurons [181] | ||
Liver | Gluconeogenesis [182] | PGC-1α, FOXO1, CRTC2 |
Glycolysis [183] | PGAM-1 | |
Lipogenesis [184] | SREBP1c | |
Fatty acid oxidation [121] | PGC-1α, PPARα | |
Cholesterol efflux [185] | LXRα | |
Pancreas | Insulin secretion in β-cells [186] | FOXO, UCP2 |
Immune cells | Inflammation pathways [187] | NF-κB |
Adipocytes | Fat storage in WAT, browning of fat, differentiation of BAT [188] | PPARγ, prdm16, PGC-1α |
Heart | Hypertrophy [189] | PPARγ |
Ischemic tolerance [190] | eNOS | |
Skeletal Muscle | Glycolysis [191] | LKB1, AMPK, PCG-1α, |
Fatty acid oxidation [191] | LKB1, AMPK, PCG-1α, PPARα |
AMPK, Adenosine monophosphate-activated protein kinase; CRTC2, CREB (cAMP response element-binding protein) Regulated Transcription Coactivator 2; eNOS, endothelial nitric oxide synthase; FOXOs, Forkhead box O transcription factors; FXR, farnesoid X receptor; LKB1, Liver Kinase B1; LXR α, liver X receptor alpha; NF-κB, Nuclear factor kappa-light-chain-enhancer of activated B cells; p53, tumour protein P53; PGAM-1, Phosphoglycerate Mutase 1; PPARα, Peroxisome proliferator-activated receptor (PPAR)-alpha; PPARγ; PPAR gamma; PGC-1α, PPARγ coactivator 1-alpha; prdm16, PR/SET Domain 16; SREBP1c, Sterol regulatory-element binding proteins 1c.
Sirt1 and cholesterol metabolism
Cholesterol homeostasis is necessary for brain development and neuronal functioning, so it is precisely regulated. Increased levels of circulating cholesterol have been associated with an elevated risk of dementia and AD, and several studies have found a reduced risk of AD in patients using cholesterol-lowering drugs [36]. Any cholesterol that is excess to the body’s need is oxidized into the oxysterols, 24-hydroxycholesterol (24-OHC) and 27-hydroxycholesterol (27-OHC), by the enzymes CYP46A1 and CYP27A1, respectively [37]. Both of these metabolites influence cholesterol biosynthesis in the brain [38], with 27–OHC suggested to elevate the risk of dementia and AD, while 24-OHC is suggested to have neuroprotective effects [37, 39, 40]. While there is an efflux of 24–OHC from the brain to the peripheral circulation, there is also an influx of the neurotoxic 27–OHC to the brain [41, 42]. There is a close correlation between cholesterol and 27-OHC in the circulation and hypercholesterolemia is likely to result in an increased uptake of 27-OHC, which appears to mediate the effect of hypercholesterolemia; therefore, this sterol could be one of the primary pathogenetic marker for AD incidence [43].
A beneficial effect of 24-OHC might be through modulating the deacetylase activity of Sirt1, which in turn contributes to accumulation of AD pathological features. Specifically, a recent animal study in mice revealed that 24-OHC may act as a neuroprotective metabolite by potentiating Sirt1 activity, which in turn prevented the hyperphosphorylation of tau. The underlying mechanism for the protective effect of 24-OHC is proposed to be through improved Sirt1-dependent deacetylation, which prevents the neurotoxic degradation of p-tau, making p-tau more susceptible to ubiquitination and reducing tau tangles in neurons [40]. Sirt1 also plays a crucial role in cholesterol metabolism by deacetylating, and therefore activating LXRs, which are a key regulator of lipid metabolism [44]. The main function of LXRs include reverse hepatic cholesterol transport, regulating efflux of high density lipoprotein cholesterol-containing lipoproteins, degrading lipoproteins, and removing cholesterol from the body by conversion to bile acids [44]. A recent study showed that a mutation of LXRα at Lys-432 disrupts the deacetylation activity of Sirt1, and thus impaired LXR transcription, highlighting the importance of LXR deacetylation by Sirt1 [45].
Sirt1 and inflammation
Sirt1 is associated with many chronic inflammatory diseases including obesity, heart disease, and type 2 diabetes mellitus (T2DM), all of which are either common co-morbidities of, or major risk factors for, dementia and AD [46–48]. Evidence has emerged that Aβ accumulation is modulated by inflammation in brain cells, leading to neuronal loss and the progression of AD [49, 50]. The amyloidogenic pathway can stimulate inflammation processes and induce the brain’s immune system to release pro-inflammatory cytokines [51, 52]. Further, epidemiological studies have shown that non-steroidal anti-inflammatory drugs (NSAIDs) are associated with less cognitive deficits in older adults and reduced AD risk [53]. Sirt1 can reprogram inflammatory responses by influencing histones and transcription factors, such as NF-κB [54]. There is a strong association between inflammatory events in the immune system, metabolic pathways, and mitochondria to meet the nutritional requirements of the immune system. The sirtuin family appears to be involved in the regulation of these complex networks [54, 55]. NF-κB is considered to be a master inflammation regulator where, in response to pro-inflammatory cytokines, it translocates to the nucleus and induces the transcription of a cascade of pro-inflammatory cytokines and chemokines [56, 57]. Moreover, NF-κB may also mediate synaptic plasticity, learning, and memory [58]. A recent study has demonstrated impairment of NF-κB in AD associated with the characteristic reduction in ability to form new memories [58]. Sirt1 impacts NF-κB by deacetylation of p65 at lysine 310 [59], suppressing NF-κB transcriptional activation which is induced by the pro-inflammatory cytokine tumor necrosis factor alpha (TNFα). The inhibition of NF-κB signaling has been suggested to be a mechanism through which Sirt1 could protect neuronal cells against microglia-dependent Aβ toxicity [60]. Elevated Sirt1 levels could also suppress the inflammatory response in the hippocampus and thus augment cognitive abilities [61].
Other biological pathways relevant to AD
Circadian rhythm and the gut microbiome are two examples of AD-related biological processes that can be influenced by lifestyle [62, 63]. Moreover, growing evidence highlights the importance of Sirt1/SIRT1 for the regulation of circadian oscillation and gut microbial diversity [64, 65]. Here we summarize biological mechanisms that link these processes with Sirt1 and AD.
Sirt1 and circadian rhythm
Some behaviors follow a daily rhythm, including sleeping and eating [66]. The circadian clock is a complex biological system that enables adaption of human cells to 24-hour day and night cycles. The cellular circadian system comprises both a positive and negative limb. The positive limb is composed of BMAL1 (brain and muscle ARNT-like1) and CLOCK (Circadian Locomotor Output Cycles Kaput), while the negative limb is composed of two cryptochrome (CRY1 and CRY2) and three period (PER1, PER2, and PER3) proteins. These two limbs form a negative feedback loop where CLOCK and BMAL1 bind together and enhance the transcription of PER and CRY genes. The resultant PER and CRY proteins form complexes along with other proteins (PER complex) to suppress the activity of CLOCK-BMAL1 until the PERs and CRYs degrade [67]. The suprachiasmatic nucleus (SCN) in the hypothalamus regulates mammalian circadian timekeeping [68]. There is a reciprocal link between metabolic homeostasis, SCN and peripheral tissues regarding the circadian clock and neurological regulation [62, 69]. However, aging, along with features of modern life such as prolonged exposure to light at night and shift work, predispose humans to circadian dysfunction [70].
There is growing evidence to suggest that circadian dysfunction is both a cause and a consequence of neurodegeneration. Neurodegenerative diseases likely impair the central clock and disrupt the links between different brain regions and peripheral organs. On the other hand, disruptions to rhythmic process such as sleep and metabolism may increase the susceptibility of the brain to further degeneration [62]. Animal studies provide evidence that circadian dysfunction can contribute to AD pathology either directly, by exacerbating Aβ deposition, or indirectly via effects on the metabolic cycle [62]. Sirt1 is considered a potential regulator of circadian gene expression through PGC1-α, thereby modulating the transcription of core CLOCK genes, including CLOCK, BMAL1, PER2, CRY1, and ROR γ (retinoic acid-related orphan receptor gamma) [64]. In mice, aging was accompanied by decreased Sirt1 levels in the SCN and therefore a reduction in circadian regulatory proteins in the brain, highlighting the significant contribution of Sirt1 to age-related reduction in circadian function [71, 72]. The activity of Sirt1 also depends on the cellular level of NAD+ with production modulated by the circadian machinery, creating a critical link between the circadian clock, metabolism, and Sirt1 activation [10]. Therefore, it seems that any age-related impairment in the circadian system and Sirt1/SIRT1, in addition to that discussed above, might further impact metabolism and lead to health issues.
Sirt1 and gut microbiome
The gut microbiome, referred to as the ’forgotten organ’, comprises all microbes living in the gastrointestinal (GI) tract, including bacteria, viruses, fungi, and archaea [73, 74]. GI microbiota affect human health, either directly by generating biological substances such as essential amino acids, vitamins and lipids, or indirectly by mediating metabolism, intestinal epithelium barrier function, and immune responses [74]. Gut microbial diversity varies among individuals due to genetic and lifestyle factors [74], and typically declines with age [75, 76]. Improvements to detrimental lifestyle factors such as poor diet, suboptimal sleep, circadian rhythm disturbance, chronic noise, and sedentary behavior have been shown to improve and protect against age-related decline in gut microbial diversity [77]. Strong evidence for a genetic contribution to individual variation in the gut microbiome comes from twin studies, where the microbiome composition of monozygotic twins is more similar than that of dizygotic twins [78, 79]. A recent study found lower GI microbial diversity in AD patients compared to controls [63], with AD patients showing a higher frequency of Bacteroidetes, and reduced frequencies of Firmicutes and Bifidobacterium [63].
One possible explanation for the reduced number and diversity of gut microbes in people with AD, in addition to concomitant dietary-decline, is the reduction of their adhesion to the intestinal wall due to changes in the chemical composition and structure of the colon mucous membrane [80]. Alternations in gut microbiota play roles in disease progression, probably through influencing on immune system and systemic inflammation [81]. Although, the exact mechanisms which the gut impacts on neuropathology, emerging evidence propose the role of a gut-brain axis allowing bi-directional link between the gut and brain [82], both at the genetic [83] and microbial diversity level [84]. Sirt1 might impact gut microbiome homeostasis by improving intestinal barrier functions [85, 86], which may mechanistically result through Sirt1 deacetylation of SPDEF (SAM pointed domain containing ETS transcription factor), which is involved in the differentiation of the epithelial lining of the small intestine [87]. Other potential mechanisms by which Sirt1 may affect the gut microbiome include an anti-inflammatory effect of Sirt1 to reduce gut-related reactive oxygen species [65], its anti-apoptotic role to improve intestinal structure [86], and regulatory effects on tight junction-related genes [88].
SIRT1, LIFESTYLE FACTORS, AND AD COMORBIDITIES
A suboptimal lifestyle might be one of the main modifiable risk factors for global burden of chronic diseases and accounts for about 63% of all deaths [89]. There is growing evidence to suggest that addressing lifestyle factors such as, but not limited to, diet, physical activity, and sleep could be a viable preventative strategy for AD [90]. In the following sections, we summarize previous studies of potential associations between Sirt1/SIRT1 and lifestyle factors with respect to dementia and AD-related risk factors. Lifestyle factors that will be reviewed here in relation to Sirt1/SIRT1 and AD include dietary factors, physical activity, and smoking, while we have reviewed sleep previously [91]. There are several known common comorbidities linked with AD, with evidence at the mechanistic, epidemiological, and genetic level associating multiple disorders, such as those of the cardiovascular system, and gastrointestinal tract as well as T2DM and depression. In this review, we highlight the role of Sirt1 in depression, the most common psychiatric disease in the population and estimated to affect around 40% of people with AD.
Sirt1 and dietary factors
Caloric restriction, defined as up to a 50% reduction in calorie consumption, has been suggested to have significant neuroprotective effects [92] in addition to being associated with an increased lifespan, delayed aging, and protection from age-related diseases, such as AD [93, 94]. Young AD transgenic mouse models who were subject to caloric restriction displayed reduced accumulation of Aβ plaques and activation of astrocytes [95, 96]. This was associated with upregulation of genes linked to neurogenesis and neuroplasticity, and downregulation of inflammatory genes [97]. A recent study showed that habitually higher dietary energy intake results in an increased risk of AD incidence in individuals carrying the apolipoprotein E (APOE) ɛ4 allele [98], a major genetic risk factor for AD. Similarly, in individuals with mild cognitive impairment (which often but not always precedes dementia) who followed a high calorie diet, the risk of developing AD increased two fold compared with subjects following a lower calorie diet [99]. Recent research has shown that caloric restriction in transgenic mice could simulate SIRT1 overexpression, improving glucose metabolism and extending lifespan [100].
One of the best-described molecular mechanisms by which caloric restriction affects aging is via the Sirt1 signaling pathway (AMPK (Adenosine monophosphate-activated protein kinase)-Sirt1-PGC-1α) [101]. Both Sirt1 and AMPK are highly conserved energy sensors that respond to elevated levels of NAD+ and AMP, respectively. During fasting, elevated AMP/ATP (Adenosine triphosphate) ratio (an energy indicator) results in AMPK activation. AMPK directly phosphorylates PGC-1α, preparing this biomolecule to be deacetylated by Sirt1 and thus fully activated [102]. Sirt1’s deacetylation of PGC-1α has been extensively implicated in metabolic regulation and biogenesis of mitochondria and proposed as a potential mechanism linking Sirt1 to a role in caloric restriction and longevity [103]. Human studies have reported that DNA content in mitochondria increased by 35% in the skeletal muscle biopsies collected from participation with CR, demonstrating increased mitochondrial mass which is followed by increases in SIRT1 gene expression [104]. Despite these findings, significant further research is needed to clarify whether Sirt1 activation is a promising target to achieve good health and longevity in human being [105].
The Mediterranean diet (MeDi) is common in countries bordering the Mediterranean Sea [106]. It includes large quantities of fruits and vegetables, and omega-3 fatty acids from olive oil, which is believed to improve plasma antioxidant capacity [107]. Adherence to the MeDi has previously been linked with improved cognitive abilities, reduced inflammation, a lower risk of neurodegenerative diseases such as AD [108, 109], slower rate of brain Aβ accumulation, increased cortical thickness, and reduced brain atrophy [110] and improved longevity [111, 112]. It has been suggested that polyphenols in the MeDi have significant antioxidant properties, particularly resveratrol [113]. In an intervention study, it was shown that administration of resveratrol (10 mg/kg) over a period of 8 months could improve the integrity of the hippocampus and consequently cognitive performance in rats [114]. Resveratrol is a well-established activator of Sirt1 and appears to influence longevity through the activation of sirtuin pathways [115].
The Western diet, which is characterized by high intake of saturated fats and refined sugars and low intake of fiber, is a hallmark of the modern lifestyle and is emerging as a risk factor for AD and dementia [116]. Several studies have reported an association between the Western diet and various chronic diseases, including AD [116–118]. Both human and animal studies have shown that the Western diet can increase Aβ deposition and tau phosphorylation, and exacerbate cognitive decline, while following a diet lower in fat and sugar can improve dementia and cognition [119]. A proposed hypothesis is that oxidative stress is one of the primary consequences of a high energy intake and plays a critical role in cognitive impairment [120]. Sirt1 as a main regulator of metabolism modulates fatty acid metabolism via an interaction with several metabolic sensors, including AMPK and PGC-1α [121–123]. Other potential contributions of Sirt1 to fat metabolism include improving insulin signaling through fat mobilization and mammalian target of rapamycin signaling [124], and elevating insulin release in the pancreas, where it acts as an anti-inflammatory factor [125].
Sirt1 and physical activity
A sedentary lifestyle is a common feature of modern society and is a public health concern [126]. Being physically inactive has been shown to be a major risk factor for cognitive decline, dementia, and AD, while physical exercise appears to be an effective strategy to improve cognitive abilities [127, 128]. Skeletal muscle and brain are post-mitotic tissues and have limited ability for cellular renewal, making them particularly susceptible to age-related decline [129]. Both animal and human studies have shown that being physically active directly influences brain structure and function [130]. There is emerging evidence for neuroprotective effects of regular exercise, including improving oxidative stress status [131], elevating BDNF levels/expression [132], and mitochondrial biogenesis [133]. It is proposed that the beneficial effects of regular exercise on brain health and longevity could be due to Sirt1-related mechanisms.
Physical exercise causes cellular metabolic stress, which in turn impacts the Sirtuins. Metabolic stress increases ATP demand and leads to elevated levels of the Sirt1 substrate, NAD+. Consequently, elevated Sirt1 enzyme contributes to biogenesis of mitochondria through a PGC-1α-dependent pathway [134]. In a 6-week high intensity training study, an increase in mitochondrial activity and an elevation of PGC-1α protein was detected in skeletal muscle 4 days after training [135]. Sirt1 protein was also found to be decreased post-intervention while its total activity in muscles and activity per protein increased [135]. However, contradictory studies report that either Sirt1 protein increased after physical exercise [136], or that there were no reported changes in Sirt1 mRNA expression [137]. Of note, increased mRNA is not paralleled by increased protein because there are complex regulatory points for protein transcription after gene expression. Moreover, a recent study showed that 30 minutes post-exercise Sirt1 protein remained unchanged and its level increased after 120 minutes of recovery [136], therefore, sampling time point may impact outcome, as may the type of exercise prescribed and the cohort being investigated.
There is evidence to support the notion that exercise can improve learning processes and memory formation by increasing BDNF in the hippocampus [138]. Indeed, it seems that the important mechanism linking the neuroprotective effects of exercise to Sirt1/SIRT1 is mediated through BDNF [139], which plays a crucial role in neuronal plasticity and neuronal survival [140]. Sirt1 is thought to be a regulator of BDNF transcription [141], enhancing memory function and spatial learning. A recent animal study reported that the metabolite lactate, produced in muscles during exercise, might be a stimulus for BDNF and thus improved spatial learning and memory formation [138]. Importantly, the activity of lactate depends on Sirt1 deacetylase activity [138]. The most studied BDNF genetic polymorphism is rs6265, which results in a Methionine for Valine substitution at position 66 (Val66Met). The 66Met allele is associated with increased susceptibility to cognitive deficits and neuropsychiatric disorders [142]. Importantly, a recent study revealed significantly lower expression of vascular SIRT1 in transgenic mice homozygous for the 66Met allele [143]. Another study explored the possible influence of aging and moderate exercise on Sirt1 activity by measuring the acetylation rate of proteins in the cerebellum [129]. The authors found that Sirt1 levels did not change with age, but a positive association between hand grip strength and protein acetylation was reported, suggesting that age-related decline in Sirt1 activity in the cerebellum could damage motor function [129].
Sirt1 and smoking
It is estimated that around 2 billion people worldwide smoke tobacco, leading to around 4 million deaths annually [144]. Illnesses that have been linked to smoking include heart disease and cancer, along with a wide range of neurological disorders [145, 146] such as dementia and AD [147]. Previous studies have demonstrated that active or former smokers have a significantly higher risk for AD (relative risk = 1.72; 95% CI 1.33–2.12) [148], which is markedly higher in those who carry the APOE ɛ4 allele (OR = 6.56; 95% CI = 1.80–23.94) [149]. It has been proposed that cerebral oxidative stress is the main pathophysiological mechanism involved in the neurocognitive and neurobiological impairments observed in smokers [146]. The brain is a highly energy-demanding organ with a high rate of metabolism, producing a high burden of free radicals. Given brain tissue is rich in polyunsaturated fatty acids, it is highly susceptible to peroxidation and oxidative stress [150, 151].
Sirt1 has been reported to be reduced in smokers [152], who are characterized by an imbalance of oxidant/antioxidant capacity [153, 154]. In the presence of oxidative stress, Sirt1 shifts to the nucleus, to act as an antioxidant. It appears that under oxidative stress, Sirt1 can stimulate antioxidant enzymes such as Superoxide dismutase-2 and -3 (SOD2 and SOD3) by deacetylation of FOXO3a [155]. Evidence suggests that the anti-senescence pathway Sirt1/FOXO3 is impaired in smokers, leading to disruption of the antioxidant/pro-oxidant balance [156]. Further, Sirt1 protein has been suggested to protect endothelial cells against smoking–related oxidative stress. In an animal study, rats treated with cigarette smoke showed increased reactive oxygen species, inflammatory markers and induced apoptosis, in arteries and coronary arterial endothelial cells [157]. Following treatment with the Sirt1 activator resveratrol, antioxidant, anti-inflammatory, and anti-apoptotic effects were observed via Sirt1 activation [157]. It is hypothesized that oxidative stress due to smoking decreases SIRT1 expression and increases acetylated endothelial nitric oxide synthase, which is followed by endothelial dysfunction [158].
Sirt1 and depression
Depression is prevalent in older adults and is more common among people suffering from dementia, with a rate of 30% among AD patients [159]. Depressive symptoms are often detected before memory impairment [160] and could contribute to memory decline by slowing down the generation of nerve cells [161]. It has therefore been suggested that depressive symptoms could be an early sign of memory decline, and older adults who report early signs of depression may be at risk of developing dementia. [160]. Several animal and human studies have revealed a crucial role for Sirt1 and its activators in depression and other mood disorders [162, 163]. Neuroinflammation stimulates the brain’s immune system, including microglial activation and astrocyte hypertrophy, which are known to be key contributors to stress-induced depression [164]. Treatment with the Sirt1 activator resveratrol has been reported to attenuate microglia and astrocyte activation, and increase hippocampal neurogenesis in older rats, which improved depression-like behaviors [162, 163], highlighting Sirt1 as a potential target for future antidepressant drugs [51–53]. In a mouse model of depression, chronic stress interrupted Sirt1 activity in the hippocampus, resulting in depressive behaviors [165]. By contrast, increased Sirt1 activation improved depression-related behaviors [165]. Increased Sirt1-mediated phosphorylation of extracellular signal-regulated protein kinases 1 and 2 (ERK1/2) in the chronic stress state was proposed as the underlying mechanism, as activation and blocking of hippocampal ERK2 were followed by anti-depressive and pro-depressive behaviors, respectively [165]. Notably, mood impairments are also linked to changes in circadian rhythms, and circadian disorders in various physiological processes are reported in depression. As discussed earlier, there is emerging evidence to support an effect of Sirt1 on circadian rhythm by modulating the key circadian rhythm components of CLOCK and BMAL1 [166, 167].
SIRT1 GENETIC VARIATION, AD, AND AD-RELATED RISK FACTORS
Single nucleotide polymorphisms (SNPs) in the SIRT1 gene, located on Chromosome 10 (10q21.3), have been associated with several AD-related risk factors, including cardiovascular disease and diabetes [168]. However, there is a lack of literature linking genetic variation in SIRT1 directly with AD. SIRT1 genetic variants reported by previous studies are summarized in Table 2, along with the diseases, disorders, or phenotypes they are associated with, many of which have themselves been reported as potential risk factors for AD. As such, we have reviewed these genetic associations to provide evidence to suggest that further investigation of SIRT1 genetic variation is warranted, particularly in the context of gene-environment (GxE) interactions and AD.
Table 2
Association of SIRT1 genetic variants with diseases and traits related to aging and Alzheimer’s disease
SNP | Chromosome 10 Position | Population (sample size) | Associated with: |
rs7895833G>A,C | GRCh37:69623057GRCh38:67863299 | 1. South African Indians (281) | 1. Coronary artery disease[192] |
2. Netherlands (791) | 2. Survival rate in T2DM [193] | ||
3. Netherlands (2347) | 3. BMI, obesity [193] | ||
rs12415800 | GRCh37:69624180 | Han Chinese(340) | Depressive disorder, aberrant gray matter volume, cognition[194] |
G>A,C | GRCh38:67864422 | ||
rs10997854 | GRCh37:69641369 | Ashkenazi Jewish (392) | Blood lipid [195] |
C>A,T | GRCh38:67881611 | ||
rs142194353 | GRCh37:69641539 | Ashkenazi Jewish (392) | Blood lipid, no longevity [195] |
C>T | GRCh38:67881781 | ||
rs12778366 | GRCh37:69643079 | 1. North Indian (1542) | 1. T2DM [196] |
T>C,G | GRCh38:67883321 | 2. Netherlands (535) | 2. Longevity, glucose tolerance [170] |
3. Switzerland (535) | 3. Social phobia, panic disorder [197] | ||
rs3758391 | GRCh37:69643342 | 1. Japanese (304) | 1. Diabetic [198] |
T>C | GRCh38:67883584 | 2. Dutch, Leiden (1245) | 2. CVD and cognitive ability [199] |
3. Mexican (519) | 3. Diabetes [47] | ||
4. Iranian (500) | 4. COPD [172] | ||
rs3740051 | GRCh37:69643959 | Japanese (1304) | Diabetic nephropathy [198] |
A>G,T | GRCh38:67884201 | ||
rs33957861 | GRCh37:69646976 | Han Chinese (635) | NFLD [200] |
C>T | GRCh38:67887218 | ||
rs144124002 | GRCh37:69647253 | Turkish (410) | COPD, CVD [201] |
A>G | GRCh38:67887495 | ||
rs2236319 | GRCh37:69649220 | 1. Japanese (1304) | 1. Diabetic nephropathy [198] |
A>G | GRCh38:67889462 | 2. Turkish (410) | 2. Depression [201] |
3. Austrian (1742) | 3. Carotid atherosclerosis [46] | ||
rs7896005 | GRCh37:69651125 | Russians of European Descent (1268) | Depression [202] |
A>G,T | GRCh38:67891367 | ||
rs36107781 | GRCh37:69651319 | Russians of European Descent (1268) | Depression [202] |
T>C | GRCh38:67891561 | ||
rs12413112G>A | GRCh37:69651866GRCh38:67892108 | 1. Caucasian(1013)2. Austrian | 1. Energy expenditure, insulin sensitivity, blood sugar, fat liver, lifestyle-induced improvement [48] |
(1742) | 2. Carotid atherosclerosis [46] | ||
3. Han Chinese (635) | 3. NFLD [200] | ||
rs11599176 | GRCh37:69653775 | Han Chinese (635) | NFLD [200] |
A>G | GRCh38:67894017 | ||
rs35689145 | GRCh37:69659767 | Han Chinese (635) | NFLD [200] |
G>A,C | GRCh38:67900009 | ||
rs10823108 | GRCh37:69660494 | Chinese (1066) | Diabetic nephropathy [203] |
G/A,C | GRCh38:67900736 | ||
rs7069102 | GRCh37:69663120 | 1. Turkish (200) | 1. COPD [204] |
C>G | GRCh38:67903362 | 2. Japanese (1022) | 2. Basal energy expenditure [48] |
3. German (1068) | 3. Obesity [205] | ||
rs2273773 | GRCh37:69666598 | 1. Japanese (1304) | 1. Diabetic nephropathy [198] |
T>C | GRCh38:67906841 | 2. Austrian (1742) | 2. Carotid atherosclerosis [46] |
3. Japanese (1022) | 3. Coronary artery calcification [204] | ||
4. Belgian (3575) | 4. BMI in women [205] | ||
rs773025707 | GRCh37:69667780 | Russians of European Descent (1268) | Depression [202] |
GRCh38:67908022 | |||
rs34701705 | GRCh37:69667783 | Russians of European Descent (1468) | Depression [202] |
T>C | GRCh38:67908026 | ||
rs10997870 | GRCh37:69668014 | Switzerland (3420) | Anxiety disorder, panic disorder [197] |
G>T | GRCh38:67908257 | ||
rs10823112 | GRCh37:69670816 | Chinese (611) | T2DM [206] |
A/G | GRCh38:67911059 | ||
rs1467568 | GRCh37:69675158 | 1. Austrian (1742) | 1. Carotid atherosclerosis [46] |
A>G | GRCh38:67915401 | 2. South African Indians (287) | 2. Coronary artery disease [192] |
3. Netherlands(791) | 3. Survival in diabetics /interaction with lifestyle [193] | ||
4. Netherlands (2347) | 4. BMI, obesity [193] | ||
rs4746720 | GRCh37:69676830 | 1. Chinese (500) | 1. Longevity [207] |
T>C,G | GRCh38:67917073 | 2. Chinese (482) | 2. Longevity [208] |
rs10997875 | GRCh37:69679824 | Japanese (1216) | Depression [209] |
T>A,C | GRCh38:67920067 | ||
rs497849 | GRCh37:69753620 | Netherlands (791) | Survival in diabetes [193] |
C/T | GRCh38:67993863 |
SNP, single nucleotide polymorphism; NFLD, non-alcoholic fatty liver disease; COPD, chronic obstructive pulmonary disease; CVD, cardiovascular disease; T2DM, type 2 diabetes mellitus; BMI, body mass index.
As earlier mentioned SIRT1 has a crucial role in metabolism and metabolism-related disorders such as T2DM, which themselves have strong links or are considered as comorbidities or risk-factors for AD. As such, considering GWAS that have revealed an association of the SIRT1 genetic variants on metabolism, is of relevance to AD. Recent study examined SNP rs2273773 with different metabolic phenotypes, including metabolic syndrome, seasonal variation in body weight, fasting blood glucose, and energy expenditure [169]. In a Dutch study of 1,390 participants, an association between SIRT1 genetic variants and glucose tolerance was reported, which had implications for long-term survival. In this study, carriers of the minor allele at SIRT1 rs12778366 had higher glucose tolerance and reduced mortality risk compared with wild type subjects, and the protective effect of this variant was significant in overweight and obese participants [170]. A Finnish cohort study, which investigated three SIRT1 SNPs (rs3740051, rs2236319, and rs2272773) showed that the minor allele of these SNPs was strongly predictive of elevated basal energy expenditure in healthy subjects with diabetic parents [171].
With respect to the cardiovascular system and given that cardiovascular disease and other vascular factors are considered as significant risk factors for AD, the role of Sirt1/SIRT1 is important to consider. It has been shown that Sirt1/SIRT1 protects cardiomyocytes and endothelial cells against stresses and improves survival at the systemic level [172]. At the genetic level, in a recent study among 1,705 men aged over 70 years, SIRT1 SNPs and serum Sirt1 protein were evaluated and reported two SNPs (rs3740051 and rs3758391) to be associated with arthritis, heart attack, and deafness [173]. Moreover, analysis for association of SIRT1 SNPs with cognitive abilities revealed that rs2273773-C homozygotes had a lower score on the Mini-Mental State Examination (MMSE; measure of global cognitive function) than rs2273773-T homozygotes [173]. Similarly, in a longitudinal investigation of 4,573 Rotterdam Study participants, variation at three SIRT1 SNPs (rs7895833, rs1467568, and rs497849) was evaluated against all-cause mortality [174]. While there was no association between SIRT1 genetic variation and all-cause mortality in the whole population, diabetic patients carrying risk variants had 50% higher risk of all-cause mortality, especially cardiovascular mortality. This risk was greater in smokers and individuals with lower intake of the water-soluble B vitamin, niacin [174], the former reiterating the link between Sirt1 and smoking discussed previously.
Recently, it has been reported that increased Sirt1 levels in the brain, particularly in the lateral hypothalamic and the dorsomedial nuclei, slows down aging and increases longevity in mice [175]. Several GWAS have also assessed SIRT1 SNPs in relation to lifespan and longevity, though there is a lack of consensus in the findings. In a Chinese case-control study of longevity, including 500 individuals, the SIRT1 rs4746720 variant was reported to be associated with human longevity [176]. Similarly, Kim et al. evaluated the allele and genotype frequencies of SIRT1 rs7896005 in Caucasian subjects. The authors found that the frequency of the minor allele of rs7896005 was associated with greater mean longevity [177]. However, a further study including 616 long-lived Chinese (age: 102.4±2.3 years) and 846 controls (age: 48.9±10.6 years) showed no association between 8 SIRT1 SNPs and human lifespan, at the allele, genotype or haplotype level [178].
While genetic variation in SIRT1 appears to be involved in the incidence and progression of diseases and phenotypes that themselves are risk factors for AD, there is a lack of literature directly providing evidence for associations of SIRT1 variation with either AD risk or its related pathological phenotypes, with the exception of the association of SIRT1 rs2273773-C homozygotes with lower MMSE score [173]. This raises the possibility that Sirt1/SIRT1 may impact AD in a more complex manner, potentially via interaction with environmental or lifestyle factors (i.e., GxE). As discussed earlier, one such group of factors that has gained considerable attention in AD is that of modifiable lifestyle factors or behaviors, including diet, physical activity, and sleep. These factors also offer promise as the foundation of intervention strategies aimed at delaying the onset of, or even preventing, AD. In the following section, we consider how Sirt1/SIRT1 may alter the effects of such modifiable lifestyle factors and behaviors.
SIRT1 genetic variation and lifestyle interactions
Studies of DNA sequence variation are enabling progress towards personalized medicine, which aims to determine an individual’s predisposition to various diseases as well as predict their likely response to external factors such as different nutrients, drugs, pathogens, and chemicals. While these approaches, when focused on pharmacological responses, can provide opportunities to target specific treatments to an individual to achieve the greatest efficacy and/or avoid potential side-effects, when focused on genetics and environmental factors such as lifestyle, personalized medicine can contribute to identifying the most effective non-pharmacological intervention strategy for the individual [179].
Currently there is a lack of studies investigating whether genetic variation in SIRT1 can modify the effect of lifestyle factors (e.g., diet, sleep, and physical activity) on dementia and AD development. However, there is some evidence that interactions between SIRT1 gene variations and lifestyle factors can affect outcomes of other chronic diseases, some of which are themselves AD risk factors. In addition to the investigation in the Rotterdam Study [174] discussed above, which reported on the impact of SIRT1 interactions with smoking on all-cause mortality, a further study by Weyrich et al. investigated whether SIRT1 genetic variation could explain differences in the effect of a 9-month-long lifestyle intervention (including caloric restriction and increased physical activity) on metabolic outcomes in 196 participants of the Tubingen Family Study who were at high risk of diabetes [48]. At baseline, carriers of the minor allele (A) of SIRT1 rs12413112 had significantly lower basal energy expenditure and a higher respiratory quotient than homozygotes for the major allele (G). Participants carrying the rs12413112-A allele were also poorer responders to the lifestyle intervention than GG homozygotes, showing less improvement in fasting glucose and insulin sensitivity, and a much smaller reduction in liver fat content [48], all factors associated with increased AD risk. These findings provide impetus for future studies that should be aimed at exploring the interaction between genetic variation in SIRT1 and lifestyle factors, such as diet, sleep, and physical activity, and how this interaction may impact AD risk and pathophysiology.
FUTURE RESEARCH DIRECTIONS
This review has summarized the growing body of literature, from both in vitro and in vivo (animal and human) studies, that evidences a multi-factorial relationship between Sirt1/SIRT1, and AD etiology. This evidence supports a direct relationship with AD, but also indirectly through associations with AD related biological pathways and comorbidities, both at the functional and genetic level. There is also developing evidence that this relationship is complex and modulated through the impact of environmental and lifestyle factors. However, further research is required to fully elucidate this relationship, particularly as the direct relationship of SIRT1 gene-lifestyle interactions with AD has not received significant attention. Several lines of further investigation are thus recommended. One obvious recommendation for future research is to comprehensively examine genetic variation in SIRT1 in relation to AD-related phenotypes (i.e., cognition and brain-imaging) in comprehensively phenotyped cohorts. This should be done both independently and as an interaction with modifiable lifestyle factors (e.g., physical activity, sleep, and diet) to provide more conclusive evidence as to whether there is a either a direct genetic relationship or whether SIRT1 genetic variation may moderate lifestyle associations. This could then be extended to the epigenetic level, to determine whether this is a potential mechanism through which lifestyle factors influence AD-related phenotypes. While observational studies could provide significant evidence to support the relationship between Sirt1/SIRT1 in AD, cohorts in which lifestyle interventions have been undertaken could also provide an indication to any mechanisms underpinning associations (e.g., epigenetic, gene expression) and/or whether SIRT1 genetic variation impacts the efficacy of such interventions. Taken together, this evidence could provide support for targeting specific lifestyle interventions to specific groups of individuals based on underlying genetics. Further, it could also provide evidence to support the investigation of activators of Sirt1, e.g., dietary approaches (e.g., caloric restriction), resveratrol, etc., and their efficacy for delaying or potentially even preventing the onset of dementia due to AD.
CONCLUSION
Across this review, we have presented several lines of functional evidence that Sirt1 is associated with various biological processes that are relevant to AD, including Aβ and tau metabolism, cellular and cholesterol metabolism, inflammation, and circadian rhythm. We have also summarized literature that suggests environmental factors, particularly lifestyle factors, directly impact Sirt1 levels and/or function. Further, while there is a lack of literature directly linking SIRT1 genetic variation with AD, there is evidence that SIRT1 variation is directly linked to diseases and phenotypes that themselves are risk factors for AD. There is also evidence, albeit limited, that SIRT1 genetic variation modifies the impact of lifestyle factors on chronic disease processes which are linked to AD risk. When considering this multifactorial evidence collectively, we conclude that the interaction of SIRT1 genotype with lifestyle represents a promising, yet significantly under-investigated, target for furthering our understanding of AD risk and progression. Further research in this area is warranted to aid in the development of potential personalized lifestyle intervention strategies for AD.
ACKNOWLEDGMENTS
Figures 1 and 2 were created with BioRender.com. The authors have no further acknowledgments to report relating to this manuscript.
FUNDING
MM is supported by an Edith Cowan University Higher Degree by Research scholarship. TP is supported by an Edith Cowan University Strategic Research Fellowship. SRRS is supported by a National Health and Medical Research Council (NHMRC) Emerging Leadership Investigator Grant (GNT1197315). The authors have no other funding to report that is relevant to this manuscript.
CONFLICT OF INTEREST
The authors have no conflict of interest to report.
REFERENCES
[1] | Bordone L , Guarente L ((2005) ) Calorie restriction, SIRT1 and metabolism: Understanding longevity. Nat Rev Mol Cell Biol 6: , 298. |
[2] | Quintas A , de Solís AJ , Díez-Guerra FJ , Carrascosa JM , Bogónez E ((2012) ) Age-associated decrease of SIRT1 expression in rat hippocampus: Prevention by late onset caloric restriction. Exp Gerontol 47: , 198–201. |
[3] | Tappen RM , Williams CL ((2009) ) Therapeutic conversation to improve mood in nursing home residents with Alzheimer’s disease. Res Gerontol Nurs 2: , 267–275. |
[4] | Newcombe EA , Camats-Perna J , Silva ML , Valmas N , Huat TJ , Medeiros R ((2018) ) Inflammation: The link between comorbidities, genetics, and Alzheimer’s disease. J Neuroinflammation 15: , 276. |
[5] | Pimenova AA , Raj T , Goate AM ((2018) ) Untangling genetic risk for Alzheimer’s disease. Biol Psychiatry 83: , 300–310. |
[6] | Cacabelos R , Torrellas C , Carrera I , Cacabelos P , Corzo L , Fernandez-Novoa L , Tellado I , C Carril J , Aliev G ((2016) ) Novel therapeutic strategies for dementia. CNS Neurol Disord Drug Targets 15: , 141–241. |
[7] | Coppedè F ((2014) ) The potential of epigenetic therapies in neurodegenerative diseases. Front Genet 5: , 220. |
[8] | Probst AV , Dunleavy E , Almouzni G ((2009) ) Epigenetic inheritance during the cell cycle. Nat Rev Mol Cell Biol 10: , 192. |
[9] | Qin W , Yang T , Ho L , Zhao Z , Wang J , Chen L , Zhao W , Thiyagarajan M , MacGrogan D , Rodgers JT ((2006) ) Neuronal SIRT1 activation as a novel mechanism underlying the prevention of Alzheimer disease amyloid neuropathology by calorie restriction. J Biol Chem 281: , 21745–21754. |
[10] | Imai S-i , Guarente L ((2014) ) NAD+ and sirtuins in aging and disease. Trends Cell Biol 24: , 464–471. |
[11] | Koronowski KB , Perez-Pinzon MA ((2015) ) Sirt1 in cerebral ischemia. Brain Circ 1: , 69. |
[12] | Wang G , Han JJ ((2022) ) Connections between metabolism and epigenetic modifications in cancer. Med Rev 1: , 199–221. |
[13] | Gujral P , Mahajan V , Lissaman AC , Ponnampalam AP ((2020) ) Histone acetylation and the role of histone deacetylases in normal cyclic endometrium. Reprod Biol Endocrinol 18: , 84. |
[14] | Corpas R , Revilla S , Ursulet S , Castro-Freire M , Kaliman P , Petegnief V , Giménez-Llort L , Sarkis C , Pallàs M , Sanfeliu C ((2017) ) SIRT1 overexpression in mouse hippocampus induces cognitiveenhancement through proteostatic and neurotrophic mechanisms. Mol Neurobiol 54: , 5604–5619. |
[15] | Oberdoerffer P , Michan S , McVay M , Mostoslavsky R , Vann J , Park S-K , Hartlerode A , Stegmuller J , Hafner A , Loerch P ((2008) ) SIRT1 redistribution on chromatin promotes genomic stability but alters gene expression during aging. Cell 135: , 907–918. |
[16] | Donmez G ((2012) ) The neurobiology of sirtuins and their role in neurodegeneration. Trends Pharmacol Sci 33: , 494–501. |
[17] | Wang R , Li JJ , Diao S , Kwak Y-D , Liu L , Zhi L , Büeler H , Bhat NR , Williams RW , Park EA ((2013) ) Metabolic stress modulates Alzheimer’s β-secretase gene transcription via SIRT1-PPARγ-PGC-1 in neurons. Cell Metab 17: , 685–694. |
[18] | McKenzie AJ , Spielman JL , Pointer BC , Lowry RJ , Bajwa E , Lee WC , Klegeris A ((2017) ) Neuroinflammation as a common mechanism associated with the modifiable risk factors for Alzheimer’s and Parkinson’s diseases. Curr Aging Sci 10: , 158–176. |
[19] | Zhang Z , Shen Q , Wu X , Zhang D , Xing D ((2020) ) Activation of PKA/SIRT1 signaling pathway by photobiomodulation therapy reduces Abeta levels in Alzheimer’s disease models. Aging Cell 19: , e13054. |
[20] | Gal J , Bang Y , Choi HJ ((2012) ) SIRT2 interferes with autophagy-mediated degradation of protein aggregates in neuronal cells under proteasome inhibition. Neurochem Int 61: , 992–1000. |
[21] | Min S-W , Cho S-H , Zhou Y , Schroeder S , Haroutunian V , Seeley WW , Huang EJ , Shen Y , Masliah E , Mukherjee C ((2010) ) Acetylation of tau inhibits its degradation and contributes to tauopathy. Neuron 67: , 953–966. |
[22] | Braak H , Braak E ((1991) ) Neuropathological stageing of Alzheimer-related changes. Acta Neuropathol 82: , 239–259. |
[23] | Michán S , Li Y , Chou MM-H , Parrella E , Ge H , Long JM , Allard JS , Lewis K , Miller M , Xu W ((2010) ) SIRT1 is essential for normalcognitive function and synaptic plasticity. J Neurosci 30: , 9695–9707. |
[24] | Gao J , Wang W-Y , Mao Y-W , Gräff J , Guan J-S , Pan L , Mak G , Kim D , Su SC , Tsai L-H ((2010) ) A novel pathway regulates memory and plasticity via SIRT1 and miR-134. Nature 466: , 1105–1109. |
[25] | Vaquero A , Scher M , Lee D , Erdjument-Bromage H , Tempst P , Reinberg D ((2004) ) Human SirT1 interacts with histone H1 and promotes formation of facultative heterochromatin. Mol Cell 16: , 93–105. |
[26] | Imai S-I , Armstrong CM , Kaeberlein M , Guarente L ((2000) ) Transcriptional silencing and longevity protein Sir2 is an NAD-dependent histone deacetylase. Nature 403: , 795–800. |
[27] | Kemper JK , Choi S-E , Kim DH ((2013) ) Sirtuin 1 deacetylase: A key regulator of hepatic lipid metabolism. Vitam Horm 91: , 385–404. |
[28] | Schug TT , Li X ((2011) ) Sirtuin 1 in lipid metabolism and obesity. Ann Med 43: , 198–211. |
[29] | Houtkooper RH , Cantó C , Wanders RJ , Auwerx J ((2010) ) The secret life of NAD+: An old metabolite controlling new metabolic signaling pathways. Endocrine Rev 31: , 194–223. |
[30] | Li X ((2013) ) SIRT1 and energy metabolism. Acta Biochim Biophys Sin 45: , 51–60. |
[31] | Ramadori G , Lee CE , Bookout AL , Lee S , Williams KW , Anderson J , Elmquist JK , Coppari R ((2008) ) Brain SIRT1: Anatomical distribution and regulation by energy availability. J Neurosci 28: , 9989–9996. |
[32] | Ikeda M , Brown J , Holland AJ , Fukuhara R , Hodges J ((2002) ) Changes in appetite, food preference, and eating habits in frontotemporal dementia and Alzheimer’s disease. J Neurol Neurosurg Psychiatry 73: , 371–376. |
[33] | Ishii M , Iadecola C ((2015) ) Metabolic and non-cognitive manifestations of Alzheimer’s disease: The hypothalamus as both culprit and target of pathology. Cell Metab 22: , 761–776. |
[34] | Moser VA , Pike CJ ((2017) ) Obesity accelerates Alzheimer-related pathology in APOE4 but not APOE3 mice. eNeuro 4: , ENEURO.0077-17.2017. |
[35] | Leboucher A , Laurent C , Fernandez-Gomez F-J , Burnouf S , Troquier L , Eddarkaoui S , Demeyer D , Caillierez R , Zommer N , Vallez E ((2013) ) Detrimental effects of diet-induced obesity on τ pathology are independent of insulin resistance in τ transgenic mice. Diabetes 62: , 1681–1688. |
[36] | Solomon A , Kåreholt I , Ngandu T , Winblad B , Nissinen A , Tuomilehto J , Soininen H , Kivipelto M ((2007) ) Serum cholesterol changes after midlife and late-life cognition: Twenty-one-year follow-up study. Neurology 68: , 751–756. |
[37] | Björkhem I , Cedazo-Minguez A , Leoni V , Meaney S ((2009) ) Oxysterols and neurodegenerative diseases. Mol Aspects Med 30: , 171–179. |
[38] | Ali Z , Heverin M , Olin M , Acimovic J , Lövgren-Sandblom A , Shafaati M , Båvner A , Meiner V , Leitersdorf E , Björkhem I ((2013) ) On the regulatory role of side-chain hydroxylated oxysterols in the brain. Lessons from CYP27A1 transgenic and cyp27a1–/–mice. J Lipid Res 54: , 1033–1043. |
[39] | Mateos L , Akterin S , Gil-Bea FJ , Spulber S , Rahman A , Björkhem I , Schultzberg M , Flores-Morales A , Cedazo-Mínguez A ((2009) ) Activity-regulated cytoskeleton-associated protein in rodent brain is down-regulated by high fat diet and by 27-hydroxycholesterol in vitro. Brain Pathol 19: , 69–80. |
[40] | Testa G , Staurenghi E , Giannelli S , Gargiulo S , Guglielmotto M , Tabaton M , Tamagno E , Gamba P , Leonarduzzi G ((2018) ) A silver lining for 24-hydroxycholesterol in Alzheimer’s disease: The involvement of the neuroprotective enzyme sirtuin 1. Redox Biol 17: , 423–431. |
[41] | Heverin M , Meaney S , Lutjohann D , Diczfalusy U , Wahren J , Bjorkhem I ((2005) ) Crossing the barrier: Net flux of 27-hydroxycholesterol into the human brain. J Lipid Res 46: , 1047–1052. |
[42] | Shafaati M , Marutle A , Pettersson H , Lovgren-Sandblom A , Olin M , Pikuleva I , Winblad B , Nordberg A , Bjorkhem I ((2011) ) Marked accumulation of 27-hydroxycholesterol in the brains of Alzheimer’s patients with the Swedish APP 670/671 mutation. J Lipid Res 52: , 1004–1010. |
[43] | Loera-Valencia R , Goikolea J , Parrado-Fernandez C , Merino-Serrais P , Maioli S ((2019) ) Alterations in cholesterol metabolism as a risk factor for developing Alzheimer’s disease: Potential novel targets for treatment. J Steroid Biochem Mol Biol 190: , 104–114. |
[44] | Tontonoz P , Mangelsdorf DJ ((2003) ) Liver X receptor signaling pathways in cardiovascular disease. Mol Endocrinol 17: , 985–993. |
[45] | Li X , Zhang S , Blander G , Jeanette GT , Krieger M , Guarente L ((2007) ) SIRT1 deacetylates and positively regulates the nuclear receptor LXR. Mol Cell 28: , 91–106. |
[46] | Kedenko L , Lamina C , Kedenko I , Kollerits B , Kiesslich T , Iglseder B , Kronenberg F , Paulweber B ((2014) ) Genetic polymorphisms at SIRT1 and FOXO1 are associated with carotid atherosclerosis in the SAPHIR cohort. BMC Med Genet 15: , 112. |
[47] | Cruz M , Valladares-Salgado A , Garcia-Mena J , Ross K , Edwards M , Angeles-Martinez J , Ortega-Camarillo C , Escobedo De La Peña J , Burguete-Garcia A , Wacher-Rodarte N ((2010) ) Candidate gene association study conditioning on individual ancestry in patients with type 2 diabetes and metabolic syndrome from Mexico City. Diabetes Metab Res Rev 26: , 261–270. |
[48] | Weyrich P , Machicao F , Reinhardt J , Machann J , Schick F , Tschritter O , Stefan N , Fritsche A , Häring H-U ((2008) ) SIRT1 genetic variants associate with the metabolic response of Caucasians to a controlled lifestyle intervention–the TULIP Study. BMC Med Genet 9: , 100. |
[49] | Aisen PS ((1997) ) Inflammation and Alzheimer’s disease: Mechanisms and therapeutic strategies. Gerontology 43: , 143–149. |
[50] | Akiyama H , Barger S , Barnum S , Bradt B , Bauer J , Cole GM , Cooper NR , Eikelenboom P , Emmerling M , Fiebich BL ((2000) ) Inflammation and Alzheimer’s disease. Neurobiol Aging 21: , 383–421. |
[51] | Guo J-t , Yu J , Grass D , de Beer FC , Kindy MS ((2002) ) Inflammation-dependent cerebral deposition of serum amyloid a protein in a mouse model of amyloidosis. J Neurosci 22: , 5900–5909. |
[52] | Rogers JT , Leiter LM , McPhee J , Cahill CM , Zhan S-S , Potter H , Nilsson LN ((1999) ) Translation of the Alzheimer amyloid precursor protein mRNA is up-regulated by interleukin-1 through 5′-untranslated region sequences. J Biol Chem 274: , 6421–6431. |
[53] | Etminan M , Gill S , Samii A ((2003) ) Effect of non-steroidal anti-inflammatory drugs on risk of Alzheimer’s disease: Systematic review and meta-analysis of observational studies. BMJ 327: , 128. |
[54] | Xie J , Zhang X , Zhang L ((2013) ) Negative regulation of inflammation by SIRT1. Pharmacol Res 67: , 60–67. |
[55] | Vachharajani VT , Liu T , Wang X , Hoth JJ , Yoza BK , McCall CE ((2016) ) Sirtuins link inflammation and metabolism. J Immunol Res 2016: , 8167273. |
[56] | Goh FG , Midwood KS ((2012) ) Intrinsic danger: Activation of Toll-like receptors in rheumatoid arthritis. Rheumatology 51: , 7–23. |
[57] | Viatour P , Merville M-P , Bours V , Chariot A ((2005) ) Phosphorylation of NF-κB and IκB proteins: Implications in cancer and inflammation. Trends Biochem Sci 30: , 43–52. |
[58] | Snow WM , Albensi BC ((2016) ) Neuronal gene targets of NF-κB and their dysregulation in Alzheimer’s disease. Front Mol Neurosci 9: , 118. |
[59] | Chen Lf , Mu Y , Greene WC ((2002) ) Acetylation of RelA at discrete sites regulates distinct nuclear functions of NF-κB. EMBO J 21: , 6539–6548. |
[60] | Chen J , Zhou Y , Mueller-Steiner S , Chen L-F , Kwon H , Yi S , Mucke L , Gan L ((2005) ) SIRT1 protects against microglia-dependent amyloid-β toxicity through inhibiting NF-κB signaling. J Biol Chem 280: , 40364–40374. |
[61] | Yan J , Luo A , Gao J , Tang X , Zhao Y , Zhou B , Zhou Z , Li S ((2019) ) The role of SIRT1 in neuroinflammation and cognitive dysfunction in aged rats after anesthesia and surgery. Am J Transl Res 11: , 1555. |
[62] | Chauhan R , Chen K-F , Kent BA , Crowther DC ((2017) ) Central and peripheral circadian clocks and their role in Alzheimer’s disease. Dis Model Mech 10: , 1187–1199. |
[63] | Vogt NM , Kerby RL , Dill-McFarland KA , Harding SJ , Merluzzi AP , Johnson SC , Carlsson CM , Asthana S , Zetterberg H , Blennow K ((2017) ) Gut microbiome alterations in Alzheimer’s disease. Sci Rep 7: , 13537. |
[64] | Doi M , Hirayama J , Sassone-Corsi P , ((2006) ) Circadian regulator CLOCK is a histone acetyltransferase. Cell 125: , 497–508. |
[65] | Wang G , Yao J , Li Z , Zu G , Feng D , Shan W , Li Y , Hu Y , Zhao Y , Tian X ((2016) ) miR-34a-5p inhibition alleviates intestinal ischemia/reperfusion-induced reactive oxygen species accumulation and apoptosis via activation of SIRT1 signaling. Antioxid Redox Signal 24: , 961–973. |
[66] | Hut RA , Kronfeld-Schor N , van der Vinne V , De la Iglesia H ((2012) ) In search of a temporal niche: Environmental factors. Prog Brain Res 199: , 281–304. |
[67] | Colwell CS ((2011) ) Linking neural activity and molecular oscillations in the SCN. Nat Rev Neurosci 12: , 553–569. |
[68] | Salgado-Delgado R , Tapia Osorio A , Saderi N , Escobar C ((2011) ) Disruption of circadian rhythms: A crucial factor in the etiology of depression. Depress Res Treat 2011: , 839743. |
[69] | Mohawk JA , Green CB , Takahashi JS ((2012) ) Central and peripheral circadian clocks in mammals. Ann Rev Neurosci 35: , 445–462. |
[70] | Antunes L , Levandovski R , Dantas G , Caumo W , Hidalgo M ((2010) ) Obesity and shift work: Chronobiological aspects. Nutr Res Rev 23: , 155–168. |
[71] | Braidy N , Guillemin GJ , Mansour H , Chan-Ling T , Poljak A , Grant R ((2011) ) Age related changes in NAD+ metabolism oxidative stress and Sirt1 activity in Wistar rats. PloS One 6: , e19194. |
[72] | Chang H-C , Guarente L ((2013) ) SIRT1 mediates central circadian control in the SCN by a mechanism that decays with aging. Cell 153: , 1448–1460. |
[73] | Hill JM , Clement C , Pogue AI , Bhattacharjee S , Zhao Y , Lukiw WJ ((2014) ) Pathogenic microbes, the microbiome, and Alzheimer’s disease (AD). Front Aging Neurosci 6: , 127. |
[74] | O’Hara AM , Shanahan F ((2006) ) The gut flora as a forgotten organ. EMBO Rep 7: , 688–693. |
[75] | Nagpal R , Neth BJ , Wang S , Craft S , Yadav H ((2019) ) Modified Mediterranean-ketogenic diet modulates gut microbiome and short-chain fatty acids in association with Alzheimer’s disease markers in subjects with mild cognitive impairment. EBioMedicine 47: , 529–542. |
[76] | O’Toole PW , Claesson MJ ((2010) ) Gut microbiota: Changes throughout the lifespan from infancy to elderly. Int Dairy J 20: , 281–291. |
[77] | Askarova S , Umbayev B , Masoud A-R , Kaiyrlykyzy A , Safarova Y , Tsoy A , Olzhayev F , Kushugulova A ((2020) ) The links between the gut microbiome, aging, modern lifestyle and Alzheimer’s disease. Front Cell Infect Microbiol 10: , 104. |
[78] | Goodrich JK , Davenport ER , Beaumont M , Jackson MA , Knight R , Ober C , Spector TD , Bell JT , Clark AG , Ley RE ((2016) ) Genetic determinants of the gut microbiome in UK twins. Cell Host Microbe 19: , 731–743. |
[79] | Xie H , Guo R , Zhong H , Feng Q , Lan Z , Qin B , Ward KJ , Jackson MA , Xia Y , Chen X ((2016) ) Shotgun metagenomics of 250 adult twins reveals genetic and environmental impacts on the gut microbiome. Cell Syst 3: , 572–584. e573. |
[80] | He F , Ouwehand AC , Isolauri E , Hosoda M , Benno Y , Salminen S ((2001) ) Differences in composition and mucosal adhesion of bifidobacteria isolated from healthy adults and healthy seniors. Curr Microbiol 43: , 351–354. |
[81] | DuPont AW , DuPont HL ((2011) ) The intestinal microbiota and chronic disorders of the gut. Nat Rev Gastroenterol Hepatol 8: , 523–531. |
[82] | Cryan JF , Dinan TG ((2012) ) Mind-altering microorganisms: The impact of the gut microbiota on brain and behaviour. Nat Rev Neurosci 13: , 701–712. |
[83] | Adewuyi EO , O’Brien EK , Nyholt DR , Porter T , Laws SM ((2022) ) A large-scale genome-wide cross-trait analysis reveals shared genetic architecture between Alzheimer’s disease and gastrointestinal tract disorders. Commun Biol 5: , 691. |
[84] | Ferreiro AL , Choi J , Ryou J , Newcomer EP , Thompson R , Bollinger RM , Hall-Moore C , Ndao IM , Sax L , Benzinger TLS , Stark SL , Holtzman DM , Fagan AM , Schindler SE , Cruchaga C , Butt OH , Morris JC , Tarr PI , Ances BM , Dantas G ((2023) ) Gut microbiome composition may be an indicator of preclinical Alzheimer’s disease. Sci Transl Med 15: , eabo2984. |
[85] | Sasso GL , Ryu D , Mouchiroud L , Fernando SC , Anderson CL , Katsyuba E , Piersigilli A , Hottiger MO , Schoonjans K , Auwerx J ((2014) ) Loss of Sirt1 function improves intestinal anti-bacterial defense and protects from colitis-induced colorectal cancer. PloS One 9: , e102495. |
[86] | Wang Y , Shi X , Qi J , Li X , Uray K , Guan X ((2012) ) SIRT1 inhibits the mouse intestinal motility and epithelial proliferation. Am J Physiol Gastrointest Liver Physiol 302: , G207–G217. |
[87] | Lo Sasso G , Ryu D , Mouchiroud L , Fernando SC , Anderson CL , Katsyuba E , Piersigilli A , Hottiger MO , Schoonjans K , Auwerx J ((2014) ) Loss of Sirt1 function improves intestinal anti-bacterial defense and protects from colitis-induced colorectal cancer. PloS One 9: , e102495. |
[88] | Ma Y-h , Xu C , Wang W-s , Sun L , Yang S , Lu D , Liu Y , Yang H ((2014) ) Role of SIRT1 in the protection of intestinal epithelial barrier under hypoxia and its mechanism. Zhonghua Wei Chang Wai Ke Za Zhi 17: , 602–606. |
[89] | Kushner RF , Sorensen KW ((2013) ) Lifestyle medicine: The future of chronic disease management. Curr Opin Endocrinol Diabetes Obes 20: , 389–395. |
[90] | Livingston G , Huntley J , Sommerlad A , Ames D , Ballard C , Banerjee S , Brayne C , Burns A , Cohen-Mansfield J , Cooper C , Costafreda SG , Dias A , Fox N , Gitlin LN , Howard R , Kales HC , Kivimaki M , Larson EB , Ogunniyi A , Orgeta V , Ritchie K , Rockwood K , Sampson EL , Samus Q , Schneider LS , Selbaek G , Teri L , Mukadam N ((2020) ) Dementia prevention, intervention, and care: 2020 report of the Lancet Commission. Lancet 396: , 413–446. |
[91] | Mehramiz M , Porter T , Laws SM , Rainey-Smith SR ((2022) ) Sleep, Sirtuin 1 and Alzheimer’s disease: A review. Aging Brain 2: , 100050. |
[92] | Liang Y , Liu C , Lu M , Dong Q , Wang Z , Wang Z , Xiong W , Zhang N , Zhou J , Liu Q ((2018) ) Calorie restriction is the most reasonable anti-ageing intervention: A meta-analysis of survival curves. Sci Rep 8: , 5779. |
[93] | Mattison JA , Roth GS , Beasley TM , Tilmont EM , Handy AM , Herbert RL , Longo DL , Allison DB , Young JE , Bryant M ((2012) ) Impact of caloric restriction on health and survival in rhesus monkeys from the NIA study. Nature 489: , 318–321. |
[94] | Fontana L , Partridge L , Longo VD ((2010) ) Extending healthy life span—from yeast to humans. Science 328: , 321–326. |
[95] | Patel NV , Gordon MN , Connor KE , Good RA , Engelman RW , Mason J , Morgan DG , Morgan TE , Finch CE ((2005) ) Caloric restriction attenuates Aβ-deposition in Alzheimer transgenic models. Neurobiol Aging 26: , 995–1000. |
[96] | Mouton PR , Chachich ME , Quigley C , Spangler E , Ingram DK ((2009) ) Caloric restriction attenuates amyloid deposition in middle-aged dtg APP/PS1 mice. Neurosci Lett 464: , 184–187. |
[97] | Wu P , Shen Q , Dong S , Xu Z , Tsien JZ , Hu Y ((2008) ) Calorierestriction ameliorates neurodegenerative phenotypes inforebrain-specific presenilin-1 and presenilin-2 double knockoutmice. Neurobiol Aging 29: , 1502–1511. |
[98] | Luchsinger JA , Tang M-X , Shea S , Mayeux R ((2002) ) Caloric intake and the risk of Alzheimer disease. Arch Neurol 59: , 1258–1263. |
[99] | Geda YE , Ragossnig M , Roberts LA , Roberts RO , Pankratz VS , Christianson TJ , Mielke MM , Levine JA , Boeve BF , Sochor O ((2013) ) Caloric intake, aging, and mild cognitive impairment: A population-based study. J Alzheimers Dis 34: , 501–507. |
[100] | Bordone L , Cohen D , Robinson A , Motta MC , Van Veen E , Czopik A , Steele AD , Crowe H , Marmor S , Luo J ((2007) ) SIRT1 transgenic mice show phenotypes resembling calorie restriction. Aging Cell 6: , 759–767. |
[101] | Van Cauwenberghe C , Vandendriessche C , Libert C , Vandenbroucke RE ((2016) ) Caloric restriction: Beneficial effects on brain aging and Alzheimer’s disease. Mamm Genome 27: , 300–319. |
[102] | Cantó C , Auwerx J ((2009) ) PGC-1alpha, SIRT1 and AMPK, an energy sensing network that controls energy expenditure. Curr Opin Lipidol 20: , 98. |
[103] | Tang BL ((2016) ) Sirt1 and the mitochondria. Mol Cells 39: , 87. |
[104] | Civitarese AE , Carling S , Heilbronn LK , Hulver MH , Ukropcova B , Deutsch WA , Smith SR , Ravussin E ((2007) ) Calorie restriction increases muscle mitochondrial biogenesis in healthy humans. PLoS Med 4: , e76. |
[105] | Imai S-I ((2009) ) SIRT1 and caloric restriction: An insight into possible trade-offs between robustness and frailty. Curr Opin Clin Nutr Metab Care 12: , 350. |
[106] | Martínez-González MA , Salas-Salvadó J , Estruch R , Corella D , Fitó M , Ros E , Investigators P ((2015) ) Benefits of theMediterranean diet: Insights from the PREDIMED study. ProgCardiovasc Dis 58: , 50–60. |
[107] | Scoditti E , Capurso C , Capurso A , Massaro M ((2014) ) Vascular effects of the Mediterranean diet—Part II: Role of omega-3 fatty acids and olive oil polyphenols. Vasc Pharmacol 63: , 127–134. |
[108] | Kopel E , Sidi Y , Kivity S ((2013) ) Mediterranean diet for primary prevention of cardiovascular disease. N Engl J Med 369: , 672. |
[109] | Scarmeas N , Stern Y , Tang MX , Mayeux R , Luchsinger JA ((2006) ) Mediterranean diet and risk for Alzheimer’s disease. Ann Neurol 59: , 912–921. |
[110] | Luciano M , Corley J , Cox SR , Hernández MCV , Craig LC , Dickie DA , Karama S , McNeill GM , Bastin ME , Wardlaw JM ((2017) ) Mediterranean-type diet and brain structural change from 73 to 76years in a Scottish cohort. Neurology 88: , 449–455. |
[111] | Staubo SC , Aakre JA , Vemuri P , Syrjanen JA , Mielke MM , Geda YE , Kremers WK , Machulda MM , Knopman DS , Petersen RC ((2017) ) Mediterranean diet, micronutrients and macronutrients, and MRI measures of cortical thickness. Alzheimers Dement 13: , 168–177. |
[112] | Rainey-Smith SR , Gu Y , Gardener SL , Doecke JD , Villemagne VL , Brown BM , Taddei K , Laws SM , Sohrabi HR , Weinborn M ((2018) ) Mediterranean diet adherence and rate of cerebral Aβ-amyloid accumulation: Data from the Australian Imaging, Biomarkers and Lifestyle Study of Ageing. Transl Psychiatry 8: , 238. |
[113] | Silva P , Sureda A , Tur JA , Andreoletti P , Cherkaoui-Malki M , Latruffe N ((2019) ) How efficient is resveratrol as an antioxidant of the Mediterranean diet, towards alterations during the aging process? Free Radic Res 53: (Sup1), 1101–1112. |
[114] | Navarro-Cruz A , Ramírez y Ayala R , Ochoa-Velasco C , Brambila E , Avila-Sosa R , Pérez-Fernández S , Morales-Medina J , Aguilar-Alonso P ((2017) ) Effect of chronic administration ofresveratrol on cognitive performance during aging process in rats. Oxid Med Cell Longev 2017: , 8510761. |
[115] | Baur JA , Pearson KJ , Price NL , Jamieson HA , Lerin C , Kalra A , Prabhu VV , Allard JS , Lopez-Lluch G , Lewis K ((2006) ) Resveratrol improves health and survival of mice on a high-calorie diet. Nature 444: , 337–342. |
[116] | Luchsinger JA , Mayeux R ((2004) ) Dietary factors and Alzheimer’s disease. Lancet Neurol 3: , 579–587. |
[117] | Jacka FN , Cherbuin N , Anstey KJ , Sachdev P , Butterworth P ((2015) ) Western diet is associated with a smaller hippocampus: A longitudinal investigation. BMC Med 13: , 215. |
[118] | Dodge HH , Buracchio TJ , Fisher GG , Kiyohara Y , Meguro K , Tanizaki Y , Kaye JA ((2012) ) Trends in the prevalence of dementia in Japan. Int J Alzheimers Dis 2012: , 956354. |
[119] | Hanson AJ , Craft S (2015) The impact of high saturated fat and high glycemic index foods on cognitive function and Alzheimer’s disease biomarkers: Does a typical Western diet cause Alzheimer’s disease? In Diet and Nutrition in Dementia and Cognitive Decline,Martin CR, Preedy VR, eds. Academic Press, pp. 733-742. |
[120] | Molteni R , Wu A , Vaynman S , Ying Z , Barnard R , Gomez-Pinilla F ((2004) ) Exercise reverses the harmful effects of consumption of a high-fat diet on synaptic and behavioral plasticity associated to the action of brain-derived neurotrophic factor. Neuroscience 123: , 429–440. |
[121] | Gerhart-Hines Z , Rodgers JT , Bare O , Lerin C , Kim SH , Mostoslavsky R , Alt FW , Wu Z , Puigserver P ((2007) ) Metabolic control of muscle mitochondrial function and fatty acid oxidation through SIRT1/PGC-1α. EMBO J 26: , 1913–1923. |
[122] | Hou X , Xu S , Maitland-Toolan KA , Sato K , Jiang B , Ido Y , Lan F , Walsh K , Wierzbicki M , Verbeuren TJ ((2008) ) SIRT1 regulates hepatocyte lipid metabolism through activating AMP-activated protein kinase. J Biol Chem 283: , 20015–20026. |
[123] | Ponugoti B , Kim D-H , Xiao Z , Smith Z , Miao J , Zang M , Wu S-Y , Chiang C-M , Veenstra TD , Kemper JK ((2010) ) SIRT1 deacetylates and inhibits SREBP-1C activity in regulation of hepatic lipid metabolism. J Biol Chem 285: , 33959–33970. |
[124] | Ghosh HS , McBurney M , Robbins PD ((2010) ) SIRT1 negatively regulates the mammalian target of rapamycin. PLoS One 5: , e9199. |
[125] | Kitada M , Koya D ((2013) ) SIRT1 in type 2 diabetes: Mechanisms and therapeutic potential. Diabetes Metab J 37: , 315–325. |
[126] | Owen N , Sparling PB , Healy GN , Dunstan DW , Matthews CE ((2010) ) Sedentary behavior: Emerging evidence for a new health risk. Mayo Clin Proc 85: , 1138–1141. |
[127] | Wheeler MJ , Dempsey PC , Grace MS , Ellis KA , Gardiner PA , Green DJ , Dunstan DW ((2017) ) Sedentary behavior as a risk factor for cognitive decline? A focus on the influence of glycemic control in brain health. Alzheimers Dement (N Y) 3: , 291–300. |
[128] | Fenesi B , Fang H , Kovacevic A , Oremus M , Raina P , Heisz JJ ((2017) ) Physical exercise moderates the relationship of apolipoprotein E (APOE) genotype and dementia risk: A population-based study. J Alzheimers Dis 56: , 297–303. |
[129] | Marton O , Koltai E , Nyakas C , Bakonyi T , Zenteno-Savin T , Kumagai S , Goto S , Radak Z ((2010) ) Aging and exercise affect the level of protein acetylation and SIRT1 activity in cerebellum of male rats. Biogerontology 11: , 679–686. |
[130] | Voss MW , Soto C , Yoo S , Sodoma M , Vivar C , van Praag H ((2019) ) Exercise and hippocampal memory systems. Trends Cogn Sci 23: , 318–333. |
[131] | Radák Z , Kaneko T , Tahara S , Nakamoto H , Pucsok J , Sasvári M , Nyakas C , Goto S ((2001) ) Regular exercise improves cognitivefunction and decreases oxidative damage in rat brain. NeurochemInt 38: , 17–23. |
[132] | Radak Z , Taylor AW , Ohno H , Goto S ((2001) ) Adaptation to exercise-induced oxidative stress: From muscle to brain. Exerc Immunol Rev 7: , 90–107. |
[133] | Marosi K , Felszeghy K , Mehra RD , Radák Z , Nyakas C ((2012) ) Arethe neuroprotective effects of estradiol and physical exercisecomparable during ageing in female rats? Biogerontology 13: , 413–427. |
[134] | White AT , Schenk S ((2012) ) NAD+/NADH and skeletal muscle mitochondrial adaptations to exercise. Am J Physiol Endocrinol Metab 303: , E308–E321. |
[135] | Gurd BJ , Perry CG , Heigenhauser GJ , Spriet LL , Bonen A ((2010) ) High-intensity interval training increases SIRT1 activity in human skeletal muscle. Appl Physiol Nutr Metab 35: , 350–357. |
[136] | Guerra B , Guadalupe-Grau A , Fuentes T , Ponce-González JG , Morales-Alamo D , Olmedillas H , Guillén-Salgado J , Santana A , Calbet JA ((2010) ) SIRT1, AMP-activated protein kinase phosphorylationand downstream kinases in response to a single bout of sprintexercise: Influence of glucose ingestion. Eur J Appl Physiol 109: , 731–743. |
[137] | Radak Z , Bori Z , Koltai E , Fatouros IG , Jamurtas AZ , Douroudos II , Terzis G , Nikolaidis MG , Chatzinikolaou A , Sovatzidis A ((2011) ) Age-dependent changes in 8-oxoguanine-DNA glycosylase activity are modulated by adaptive responses to physical exercise in human skeletal muscle. Free Radic Biol Med 51: , 417–423. |
[138] | El Hayek L , Khalifeh M , Zibara V , Abi Assaad R , Emmanuel N , Karnib N , El-Ghandour R , Nasrallah P , Bilen M , Ibrahim P ((2019) ) Lactate mediates the effects of exercise on learning and memory through SIRT1-dependent activation of hippocampal brain-derived neurotrophic factor (BDNF). J Neurosci 39: , 2369–2382. |
[139] | Pedersen BK ((2019) ) Physical activity and muscle–brain crosstalk. Nat Rev Endocrinol 15: , 383. |
[140] | Pedersen BK , Pedersen M , Krabbe KS , Bruunsgaard H , Matthews VB , Febbraio MA ((2009) ) Role of exercise-induced brain-derived neurotrophic factor production in the regulation of energy homeostasis in mammals. Exp Physiol 94: , 1153–1160. |
[141] | Jeong H , Cohen DE , Cui L , Supinski A , Savas JN , Mazzulli JR , Yates JR , Bordone L , Guarente L , Krainc D ((2012) ) Sirt1 mediates neuroprotection from mutant huntingtin by activation of the TORC1 and CREB transcriptional pathway. Nat Med 18: , 159–165. |
[142] | Ng T , Teo SM , Yeo HL , Shwe M , Gan YX , Cheung YT , Foo KM , Cham MT , Lee JA , Tan YP ((2015) ) Brain-derived neurotrophic factor genetic polymorphism (rs6265) is protective against chemotherapy-associated cognitive impairment in patients with early-stage breast cancer. Neurooncology 18: , 244–251. |
[143] | Podracka L , Vehanen K , Tuulos T , Ronkko S , Kaarniranta K , Uusitalo H , Kalesnykas G ((2011) ) BDNF-deficiency downregulates SIRT1 and upregulates SIRT2 expression in aging mouse retina. Invest Ophthalmol Vis Sci 52: , 2697–2697. |
[144] | DeMarini DM ((2004) ) Genotoxicity of tobacco smoke and tobacco smoke condensate: A review. Mutat Res 567: , 447–474. |
[145] | Durazzo TC , Meyerhoff DJ , Nixon SJ ((2010) ) Chronic cigarette smoking: Implications for neurocognition and brain neurobiology. Int J Environ Res Public Health 7: , 3760–3791. |
[146] | Swan GE , Lessov-Schlaggar CN ((2007) ) The effects of tobacco smoke and nicotine on cognition and the brain. Neuropsychol Rev 17: , 259–273. |
[147] | Almeida OP , Garrido GJ , Alfonso H , Hulse G , Lautenschlager NT , Hankey GJ , Flicker L ((2011) ) 24-month effect of smoking cessation on cognitive function and brain structure in later life. Neuroimage 55: , 1480–1489. |
[148] | Cataldo JK , Prochaska JJ , Glantz SA ((2010) ) Cigarette smoking is a risk factor for Alzheimer’s disease: An analysis controlling for tobacco industry affiliation. J Alzheimers Dis 19: , 465–480. |
[149] | Rusanen M , Rovio S , Ngandu T , Nissinen A , Tuomilehto J , Soininen H , Kivipelto M ((2010) ) Midlife smoking, apolipoprotein E and risk of dementia and Alzheimer’s disease: A population-based cardiovascular risk factors, aging and dementia study. Dement Geriatr Cogn Disord 30: , 277–284. |
[150] | Kovacic P ((2005) ) Unifying mechanism for addiction and toxicity of abused drugs with application to dopamine and glutamate mediators: Electron transfer and reactive oxygen species. Med Hypotheses 65: , 90–96. |
[151] | Anbarasi K , Vani G , Balakrishna K , Devi CS ((2006) ) Effect of bacoside A on brain antioxidant status in cigarette smoke exposed rats. Life Sci 78: , 1378–1384. |
[152] | Iskandar AR , Liu C , Smith DE , Hu K-Q , Choi S-W , Ausman LM , Wang X-D ((2013) ) β-Cryptoxanthin restores nicotine-reduced lung SIRT1 to normal levels and inhibits nicotine-promoted lung tumorigenesis and emphysema in A/J mice. Cancer Prev Res 6: , 309–320. |
[153] | Cantó C , Sauve AA , Bai P ((2013) ) Crosstalk between poly (ADP-ribose) polymerase and sirtuin enzymes. Mol Aspects Med 34: , 1168–1201. |
[154] | Kwon H-S , Ott M ((2008) ) The ups and downs of SIRT1. Trends Biochem Sci 33: , 517–525. |
[155] | Yao H , Sundar IK , Ahmad T , Lerner C , Gerloff J , Friedman AE , Phipps RP , Sime PJ , McBurney MW , Guarente L ((2014) ) SIRT1 protects against cigarette smoke-induced lung oxidative stress via a FOXO3-dependent mechanism. Am J Physiol Lung Cell Mol Physiol 306: , L816–L828. |
[156] | Di Vincenzo S , Heijink IH , Noordhoek JA , Cipollina C , Siena L , Bruno A , Ferraro M , Postma DS , Gjomarkaj M , Pace E ((2018) ) SIRT 1/FoxO3 axis alteration leads to aberrant immune responses in bronchial epithelial cells. J Cell Mol Med 22: , 2272–2282. |
[157] | Csiszar A , Labinskyy N , Podlutsky A , Kaminski PM , Wolin MS , Zhang C , Mukhopadhyay P , Pacher P , Hu F , De Cabo R ((2008) ) Vasoprotective effects of resveratrol and SIRT1: Attenuation of cigarette smoke-induced oxidative stress and proinflammatory phenotypic alterations. Am J Physiol Heart Circ Physiol 294: , H2721–H2735. |
[158] | Arunachalam G , Yao H , Sundar IK , Caito S , Rahman I ((2010) ) SIRT1 regulates oxidant-and cigarette smoke-induced eNOS acetylation in endothelial cells: Role of resveratrol. Biochem Biophys Res Commun 393: , 66–72. |
[159] | World Health Organization ((1992) ) The ICD-10 classification of mental and behavioural disorders: Clinical descriptions and diagnostic guidelines. Weekly Epidemiol Record 67: , 227–227. |
[160] | Zahodne LB , Stern Y , Manly JJ ((2014) ) Depressive symptoms precede memory decline, but not vice versa, in non-demented older adults. J Am Geriatr Soc 62: , 130–134. |
[161] | Shelton DJ , Kirwan CB ((2013) ) A possible negative influence of depression on the ability to overcome memory interference. Behav Brain Res 256: , 20–26. |
[162] | Liu L , Zhang Q , Cai Y , Sun D , He X , Wang L , Yu D , Li X , Xiong X , Xu H ((2016) ) Resveratrol counteracts lipopolysaccharide-induced depressive-like behaviors via enhanced hippocampal neurogenesis. Oncotarget 7: , 56045. |
[163] | Kodali M , Parihar VK , Hattiangady B , Mishra V , Shuai B , Shetty AK ((2015) ) Resveratrol prevents age-related memory and mood dysfunction with increased hippocampal neurogenesis and microvasculature, and reduced glial activation. Sci Rep 5: , 8075. |
[164] | Yirmiya R , Rimmerman N , Reshef R ((2015) ) Depression as a microglial disease. Trends Neurosci 38: , 637–658. |
[165] | Abe-Higuchi N , Uchida S , Yamagata H , Higuchi F , Hobara T , Hara K , Kobayashi A , Watanabe Y ((2016) ) Hippocampal sirtuin 1 signaling mediates depression-like behavior. Biol Psychiatry 80: , 815–826. |
[166] | Nakahata Y , Sahar S , Astarita G , Kaluzova M , Sassone-Corsi P ((2009) ) Circadian control of the NAD+ salvage pathway by CLOCK-SIRT1. Science 324: , 654–657. |
[167] | Ramsey KM , Yoshino J , Brace CS , Abrassart D , Kobayashi Y , Marcheva B , Hong H-K , Chong JL , Buhr ED , Lee C ((2009) ) Circadian clock feedback cycle through NAMPT-mediated NAD+biosynthesis. Science 324: , 651–654. |
[168] | Lv Y , Lin S , Peng F ((2017) ) SIRT1 gene polymorphisms and risk of lung cancer. Cancer Manag Res 9: , 381. |
[169] | Kovanen L , Donner K , Partonen T ((2015) ) SIRT1 polymorphisms associate with seasonal weight variation, depressive disorders, and diastolic blood pressure in the general population. PLoS One 10: , e0141001. |
[170] | Figarska SM , Vonk JM , Boezen HM ((2013) ) SIRT1 polymorphism, long-term survival and glucose tolerance in the general population. PLoS One 8: , e58636. |
[171] | Lagouge M , Argmann C , Gerhart-Hines Z , Meziane H , Lerin C , Daussin F , Messadeq N , Milne J , Lambert P , Elliott P ((2006) ) Resveratrol improves mitochondrial function and protects against metabolic disease by activating SIRT1 and PGC-1α. Cell 127: , 1109–1122. |
[172] | Mohtavinejad N , Nakhaee A , Harati H , Poodineh J , Afzali M ((2015) ) SIRT1 gene is associated with cardiovascular disease in the Iranian population. Egypt J Med Hum Genet 16: , 117–122. |
[173] | Razi S , Cogger VC , Kennerson M , Benson VL , McMahon AC , Blyth FM , Handelsman DJ , Seibel MJ , Hirani V , Naganathan V ((2017) ) SIRT1polymorphisms and serum-induced SIRT1 protein expression in agingand frailty: The CHAMP study. J Gerontol A Biol Sci Med Sci 72: , 870–876. |
[174] | Zillikens MC , van Meurs JB , Sijbrands EJ , Rivadeneira F , Dehghan A , van Leeuwen JP , Hofman A , van Duijn CM , Witteman JC , Uitterlinden AG ((2009) ) SIRT1 genetic variation and mortality in type 2 diabetes: Interaction with smoking and dietary niacin. Free Radic Biol Med 46: , 836–841. |
[175] | Satoh A , Brace CS , Rensing N , Cliften P , Wozniak DF , Herzog ED , Yamada KA , Imai S-I ((2013) ) Sirt1 extends life span and delays aging in mice through the regulation of Nk2 homeobox 1 in the DMH and LH. Cell Metab 18: , 416–430. |
[176] | Huang J , Sun L , Liu M , Zhou L , Lv Z , Hu C , Huang Z , Zheng C , Zhou L , Yang Z ((2013) ) Association between SIRT1 gene polymorphisms and longevity of populations from Yongfu region of Guangxi. Zhonghua Yi Xue Yi Chuan Xue Za Zhi 30: , 55–59. |
[177] | Kim S , Bi X , Czarny-Ratajczak M , Dai J , Welsh DA , Myers L , Welsch MA , Cherry KE , Arnold J , Poon LW ((2012) ) Telomere maintenance genes SIRT1 and XRCC6 impact age-related decline in telomere length but only SIRT1 is associated with human longevity. Biogerontology 13: , 119–131. |
[178] | Lin R , Yan D , Zhang Y , Liao X , Gong G , Hu J , Fu Y , Cai W ((2016) ) Common variants in SIRT1 and human longevity in a Chinese population. BMC Med Genet 17: , 1–7. |
[179] | Collins FS , Varmus H ((2015) ) A new initiative on precision medicine. N Engl J Med 372: , 793–795. |
[180] | Sasaki T , Kim H-J , Kobayashi M , Kitamura Y-I , Yokota-Hashimoto H , Shiuchi T , Minokoshi Y , Kitamura T ((2010) ) Induction of hypothalamic Sirt1 leads to cessation of feeding via agouti-related peptide. Endocrinology 151: , 2556–2566. |
[181] | Ramadori G , Fujikawa T , Fukuda M , Anderson J , Morgan DA , Mostoslavsky R , Stuart RC , Perello M , Vianna CR , Nillni EA ((2010) ) SIRT1 deacetylase in POMC neurons is required for homeostatic defenses against diet-induced obesity. Cell Metab 12: , 78–87. |
[182] | Stumpferl SW , Brand SE , Jiang JC , Korona B , Tiwari A , Dai J , Seo J-G , Jazwinski SM ((2012) ) Natural genetic variation in yeast longevity. Genome Res 22: , 1963–1973. |
[183] | Hallows WC , Yu W , Denu JM ((2012) ) Regulation of glycolytic enzyme phosphoglycerate mutase-1 by Sirt1 protein-mediated deacetylation. J Biol Chem 287: , 3850–3858. |
[184] | Walker AK , Yang F , Jiang K , Ji J-Y , Watts JL , Purushotham A , Boss O , Hirsch ML , Ribich S , Smith JJ ((2010) ) Conserved role of SIRT1 orthologs in fasting-dependent inhibition of the lipid/cholesterol regulator SREBP. Genes Dev 24: , 1403–1417. |
[185] | Feng T , Liu P , Wang X , Luo J , Zuo X , Jiang X , Liu C , Li Y , Li N , Chen M ((2018) ) SIRT1 activator E1231 protects from experimental atherosclerosis and lowers plasma cholesterol and triglycerides by enhancing ABCA1 expression. Atherosclerosis 274: , 172–181. |
[186] | Luo G , Xiao L , Wang D , Wang N , Luo C , Yang X , Hao L ((2020) ) Resveratrol protects against ethanol-induced impairment of insulin secretion in INS-1 cells through SIRT1-UCP2 axis. Toxicol In Vitro 65: , 104808. |
[187] | Song Y , Wu Z , Zhao P ((2021) ) The protective effects of activating Sirt1/NF-κB pathway for neurological disorders. Rev Neurosci 33: , 427–438. |
[188] | Qiang L , Wang L , Kon N , Zhao W , Lee S , Zhang Y , Rosenbaum M , Zhao Y , Gu W , Farmer SR ((2012) ) Brown remodeling of white adipose tissue by SirT1-dependent deacetylation of Pparγ. Cell 150: , 620–632. |
[189] | Planavila A , Iglesias R , Giralt M , Villarroya F ((2011) ) Sirt1 acts in association with PPARα to protect the heart from hypertrophy, metabolic dysregulation, and inflammation. Cardiovasc Res 90: , 276–284. |
[190] | Mattagajasingh I , Kim C-S , Naqvi A , Yamamori T , Hoffman TA , Jung S-B , DeRicco J , Kasuno K , Irani K ((2007) ) SIRT1 promotes endothelium-dependent vascular relaxation by activating endothelial nitric oxide synthase. Proc Natl Acad Sci U S A 104: , 14855–14860. |
[191] | Lan F , Cacicedo JM , Ruderman N , Ido Y ((2008) ) SIRT1 modulation of the acetylation status, cytosolic localization, and activity of LKB1 possible role in AMP-activated protein kinase activation. J Biol Chem 283: , 27628–27635. |
[192] | Ramkaran P , Moodley D , Chuturgoon AA , Phulukdaree A , Khan S ((2016) ) Sirtuin 1 rs1467568 and rs7895833 in South African Indians with early-onset coronary artery disease. Cardiovasc J Afr 27: , 213. |
[193] | Zillikens MC , van Meurs JB , Rivadeneira F , Amin N , Hofman A , Oostra BA , Sijbrands EJ , Witteman JC , Pols HA , van Duijn CM ((2009) ) SIRT1 genetic variation is related to BMI and risk of obesity. Diabetes 58: , 2828–2834. |
[194] | Nan D , Yuqi C , Zonglin S , Chenglong D , Na L , Fang L , Cong Z , Xiufeng X ((2020) ) Association of a SIRT1 polymorphism with changes of gray matter volume in patients with first-episode medication-naïve major depression. Psychiatry Res Neuroimaging 301: , 111101. |
[195] | Han J , Atzmon G , Barzilai N , Suh Y ((2015) ) Genetic variation in SIRT1 is associated with lipid profiles but not with longevity in Ashkenazi Jews. Transl Res 165: , 480. |
[196] | Rai E , Sharma S , Kaul S , Jain K , Matharoo K , Bhanwer AS , Bamezai RN ((2012) ) The interactive effect of SIRT1 promoter region polymorphism on type 2 diabetes susceptibility in the North Indian population. PLoS One 7: , e48621. |
[197] | Libert S , Pointer K , Bell EL , Das A , Cohen DE , Asara JM , Kapur K , Bergmann S , Preisig M , Otowa T ((2011) ) SIRT1 activates MAO-A in the brain to mediate anxiety and exploratory drive. Cell 147: , 1459–1472. |
[198] | Maeda S , Koya D , Araki S-i , Babazono T , Umezono T , Toyoda M , Kawai K , Imanishi M , Uzu T , Suzuki D ((2011) ) Association between single nucleotide polymorphisms within genes encoding sirtuin families and diabetic nephropathy in Japanese subjects with type 2 diabetes. Clin Exp Nephrol 15: , 381–390. |
[199] | Kuningas M , Putters M , Westendorp RG , Slagboom PE , Van Heemst D ((2007) ) SIRT1 gene, age-related diseases, and mortality: The Leiden 85-plus study. J Gerontol A Biol Sci Med Sci 62: , 960–965. |
[200] | Hou Y , Su B , Chen P , Niu H , Zhao S , Wang R , Shen W ((2018) ) Association of SIRT1 gene polymorphism and its expression for the risk of alcoholic fatty liver disease in the Han population. Hepatol Int 12: , 56–66. |
[201] | İzmirli M , Göktekin Ö , Bacaksız A , Uysal Ö , Kılıc Ü ((2015) ) The effect of the SIRT1 2827 A>G polymorphism, resveratrol, exercise, age and occupation in Turkish population with cardiovascular disease. Anatol J Cardiol 15: , 103. |
[202] | Aftanas LI , Anisimenko MS , Berdyugina DA , Garanin AY , Maximov VN , Voevoda MI , Vyalova NM , Bokhan NA , Ivanova SA , Danilenko KV ((2019) ) SIRT1 allele frequencies in depressed patients of European descent in Russia. Front Genet 9: , 686. |
[203] | Zhao Y , Wei J , Hou X , Liu H , Guo F , Zhou Y , Zhang Y , Qu Y , Gu J , Zhou Y ((2017) ) SIRT1 rs10823108 and FOXO1 rs17446614 responsible for genetic susceptibility to diabetic nephropathy. Sci Rep 7: , 10285. |
[204] | Shimoyama Y , Mitsuda Y , Tsuruta Y , Suzuki K , Hamajima N , Niwa T ((2012) ) SIRTUIN 1 gene polymorphisms are associated with cholesterol metabolism and coronary artery calcification in Japanese hemodialysis patients. J Renal Nutr 22: , 114–119. |
[205] | van den Berg SW , Dolle ME , Imholz S , van‘t Slot R , Wijmenga C , Verschuren W , Strien C , Siezen C , Hoebee B , Feskens E ((2009) ) Genetic variations in regulatory pathways of fatty acid and glucose metabolism are associated with obesity phenotypes: A population-based cohort study. Int J Obesity 33: , 1143–1152. |
[206] | Han J , Wei M , Wang Q , Li X , Zhu C , Mao Y , Wei L , Sun Y , Jia W ((2015) ) Association of genetic variants of SIRT1 with type 2 diabetes mellitus. Gene Expr 16: , 177–185. |
[207] | Huang J , Sun L , Liu M , Zhou L , Lv Z , Hu C , Huang Z , Zheng C , Yang Z ((2013) ) Association between SIRT1 gene polymorphisms and longevity of populations from Yongfu region of Guangxi. Zhonghua Yi Xue Yi Chuan Xue Za Zhi 30: , 55–59. |
[208] | Zhang W-G , Bai X-J , Chen X-M ((2010) ) SIRT1 variants are associated with aging in a healthy Han Chinese population. Clin Chim Acta 411: , 1679–1683. |
[209] | Kishi T , Yoshimura R , Kitajima T , Okochi T , Okumura T , Tsunoka T , Yamanouchi Y , Kinoshita Y , Kawashima K , Fukuo Y ((2010) ) SIRT1 gene is associated with major depressive disorder in the Japanese population. J Affect Disord 126: , 167–173. |