Large-Scale Network Connectivity and Cognitive Function Changes After Exercise Training in Older Adults with Intact Cognition and Mild Cognitive Impairment
Abstract
Background:
Despite growing evidence regarding the association between exercise training (ET) and functional brain network connectivity, little is known about the effects of ET on large-scale within- and between-network functional connectivity (FC) of core brain networks.
Objective:
We investigated the effects of ET on within- and between-network functional connectivity of the default mode network (DMN), frontoparietal network (FPN), and salience network (SAL) in older adults with intact cognition (CN) and older adults diagnosed with mild cognitive impairment (MCI). The association between ET-induced changes in FC and cognitive performance was examined.
Methods:
33 older adults (78.0±7.0 years; 16 MCI and 17 CN) participated in this study. Before and after a 12-week walking ET intervention, participants underwent a graded exercise test, Controlled Oral Word Association Test (COWAT), Rey Auditory Verbal Learning Test (RAVLT), a narrative memory test (logical memory; LM), and a resting-state fMRI scan. We examined the within (W) and between (B) network connectivity of the DMN, FPN, and SAL. We used linear regression to examine associations between ET-related changes in network connectivity and cognitive function.
Results:
There were significant improvements in cardiorespiratory fitness, COWAT, RAVLT, and LM after ET across participants. Significant increases in DMNW and SALW, and DMN-FPNB, DMN-SALB, and FPN-SALB were observed after ET. Greater SALW and FPN-SALB were associated with enhanced LM immediate recall performance after ET in both groups.
Conclusion:
Increased within- and between-network connectivity following ET may subserve improvements in memory performance in older individuals with intact cognition and with MCI due to Alzheimer’s disease.
INTRODUCTION
It has been well-documented that aging is associated with declines in cognition [1], brain volume, including cortical and subcortical (e.g., the hippocampus) atrophy [2, 3], cerebral blood flow [4], and altered brain tissue microstructure [5]. In addition to these measurements, functional connectivity (FC) analysis has been used to characterize the functional organization of the brain in response to aging. Functional brain network connectivity is typically analyzed using resting-state functional magnetic resonance imaging (fMRI) data collected during task-free conditions [6, 7]. Using resting-state fMRI data, major functional networks of the brain have been identified including the default mode network (DMN; primary anchor regions: the medial prefrontal gyrus and posterior cingulate), the frontoparietal network (FPN; primary anchor regions: the dorsolateral prefrontal cortex and lateral posterior parietal cortex), and the salience network (SAL; primary anchor regions: the dorsal anterior cingulate cortex and insular cortex; also referred to as the cingulo-opercular network) [8, 9]. It has been suggested that these key networks have unique roles and functions. For example, the DMN is responsible for introspection and self-generated thoughts [8, 10], the FPN is responsible for higher-order cognitive processes [11], and the SAL is responsible for detecting and integrating relevant sensory information and the facilitation of switching between DMN and FPN engagement [12]. Converging evidence reports that aging is associated with reduced FC within the DMN [13], FPN [14], and SAL [15], which are indices of age-related deterioration in brain functional network organization. Clinically, age-related declines in cognition and brain network organization are also associated with increased risk for the diagnosis of mild cognitive impairment (MCI) and Alzheimer’s disease [16].
Mounting evidence suggests that exercise training (ET) mitigates age-related decline in functional network integrity. Particularly, there is a line of evidence suggesting ET-elicited neuroprotective effects are reflected by changes in brain FC [17]. For example, one study found increases in the DMN and lateral prefrontal aspects of a fronto-insular network (and overlap with the SAL network) in response to a 12-month walking ET intervention, and the ET-related increase in DMN connectivity was associated with improved executive function performance in older adults (67.3 years) [18]. In another study, 4 months of aerobic ET consisting of treadmill and cycling exercise led to an increase in FC between the right parahippocampal gyrus and left superior temporal gyrus in middle-aged adults (45.1 years) [19]. We previously reported that a 12-week walking ET was also associated with an increase in cerebellar FC with inferior parietal lobule and precuneus regions, which were associated with improvement in phonemic fluency performance in healthy older adults (78.0 years) [20]. In addition to the evidence in older adults with intact cognition, there has been great interest in the impact of ET on brain network connectivity in older adults diagnosed with MCI, a prodromal stage of dementia most often characterized by episodic memory decline with intact activities of independent daily living [21]. For example, a 12-week walking ET intervention was associated with increased DMN FC [22] and hippocampal FC [23] in older adults diagnosed with MCI. Importantly, the ET-related increase in hippocampal FC corresponded to ET-related improvements in memory performance in older adults diagnosed with MCI [23], suggesting that regular participation in exercise may be able to stimulate neural plasticity in memory networks among older individuals who have experienced cognitive decline.
Many of the studies in the exercise neuroscience literature have utilized a ‘seed-based’ approach to investigate the effects of exercise on the functional networks of the aging brain [17]. A seed-based analysis of resting-state fMRI data requires a hypothesis-driven determination of the seed regions to assess the FC between the seed region and rest of the brain [24]. Since the analysis is centered on the seed region, it is relatively simple to compute and interpretation of the results is straightforward [24]. Nonetheless, the results from seed-based analyses depend highly on the selection of the seed region(s), which could make it susceptible to bias [25]. Furthermore, the seed-based analysis is locally constrained to the FC between the seed region and brain regions that are functionally connected to the seed region, which limits our understanding about the global scale functional organization of the brain (e.g., within-network and between-network connectivity) [24].
Dorsman and colleagues (2020) investigated the longitudinal relationship between physical activity and inter- and intra-network connectivity in older adults (73.3 years). They found that longitudinal increases in self-reported participation in physical activity were associated with increased network FC including the FPN-subcortical network and intra-subcortical network [26]. Yet, physical activity was assessed by self-report and only cognitively healthy older adults were included. Hence, a prospective intervention trial is necessary to gain insights into the effects of ET on network FC in older adults, particularly those diagnosed with MCI. To address this knowledge gap, we investigated the effects of ET on within- and between-network connectivity in older adults with intact cognition and those diagnosed with MCI. We also tested if the ET-related changes in within- and between-brain network connectivity were associated with changes in cognitive performance. For the cognitive tasks, Controlled Oral Word Association Test (COWAT) [27], the Rey Auditory Verbal Learning test (RAVLT) [28], and the Wechsler Memory Scale Logical Memory subtest (LM) [29, 30] were used based on our previous findings that showed improved performance of these tasks after walking ET [20, 23, 31].
To test our research questions, the triple network model was used to examine the effects of ET on FC within the DMN, FPN, and SAL, as well as FC between each of these networks [32]. The model posits that the interconnectivity of three major brain networks (i.e., DMN, FPN, and SAL) underlies complex cognitive processes that when disrupted presumably leads to cognitive dysfunction [32]. Previous evidence suggests that aging is associated with decreased within-network FC and increased between-network FC [33]. Given the beneficial effects of ET on functional network connectivity in older adults, both with intact cognition and MCI [17, 18], we hypothesized that there would be increased within-network FC and decreased between-network FC in response to ET in both older adults with intact cognition and those diagnosed with MCI. We also hypothesized that greater ET-related increases in within-network FC and decrease in between-network FC would be associated with greater ET-related improvements in the COWAT, RALVT, and LM performances in both groups.
MATERIALS AND METHODS
Participants
A previous paper provides more detail on our participant recruitment procedures [34], which included advertisements in newspapers, talks at community and residential sites, and referrals by physicians. Telephone screening was used for health and magnetic resonance imaging (MRI) exclusions to determine preliminary eligibility, followed by a neurological and neuropsychological assessment to determine final eligibility (see Neurocognitive Testing section). Written informed consent and physician approval for participating in moderate-intensity ET were obtained from all participants. This study was approved in accordance with the Declaration at Helsinki. Testing sessions were administered within 3–5 days prior to and following the intervention.
Inclusion and exclusion criteria
As previously reported [34], we included only older individuals who, during phone screening, reported engaging in moderate-intensity physical activity on fewer than three days/week for the six months preceding the study. Other exclusionary criteria included history of or current: 1) brain injury, cardiovascular disease, or cerebral ischemia; 2) contraindications for MRI; 3) cerebrovascular or neurological disorder or disease; 4) untreated psychiatric symptoms meeting DSM-IV criteria, including but not limited to severe depression and substance use disorder; 5) current score of >15 on the 30-item Geriatric Depression Scale (GDS) [35] or evidence of impaired activities of daily living [36]; and 6) left-handedness (i.e., <50 of laterality quotient [37]).
Neurocognitive testing
As previously reported [34], a team of neuropsychologists evaluated cognitive scores to determine MCI diagnosis using National Institute of Aging-Alzheimer’s Association criteria [38], including: 1) subjective cognitive complaints; 2) cognitive impairment in at least one domain; 3) intact activities of daily living; 4) not demented. The neuropsychological test battery, administered between 0700 and 1100 hours included the COWAT [27], RAVLT [28], and LM subtest of the Wechsler Memory Scale-III [29, 30] before and following a 12-week ET intervention. The average difference between the last day of exercise and post-intervention testing across all participants was 5.3±3.9 days. The COWAT examines phonemic fluency (i.e., words starting with a specific letter, e.g., F, A, S); participants were instructed to produce as many words as possible within 60 s [27]. The total number of words produced during the tests were used as the index of the test performance in the present study. Alternate forms were used for before and after ET intervention across participants. The RAVLT (episodic memory) involves individually presenting 15 unrelated words that participants are instructed to attempt to recall after the presentation; five consecutive trials are performed [28]. After presentation and recall of a different (interference) list, immediate recall of the original words is tested, followed by delayed recall 20-min later. The primary interpretive index of the RAVLT performance was the number of words recalled at Trial 1 (T1), sum of Trial 1 to Trial 5 (T1-5), immediate recall, and delayed recall. Alternate forms of the RAVLT were counterbalanced at the before and after ET sessions to minimize possible learning effects across participants. During the LM test, the examiner reads two short stories after which participants immediately recall the stories as exactly as possible [29, 30]. A delayed recall test follows 30-min later and a yes/no recognition test of story details thereafter. The number of correct responses during the immediate recall, delayed recall, and recognition were used for the present study.
Cardiorespiratory fitness test
All participants underwent a modified submaximal exercise test to assess cardiorespiratory fitness before and after the 12-week exercise intervention, as described in our previous paper [34]. We utilized a modified Balke-Ware protocol in which exercise speed was constant at 3.2 km/h and grade increased 1°/min (start at 0° grade) motorized treadmill. Metabolic data, including rate of oxygen (
Exercise intervention
The study participants completed a 12-week treadmill walking intervention. A certified personal trainer or exercise physiologist supervised all exercise sessions in local recreation centers. Each session lasted 30 min and occurred four days per week. A HR monitor (Polar Electro, Kempele, Finland) and the RPE scale [40] were used during each exercise sessions to monitor the intensity of the exercise and to maintain a moderate intensity. The exercise session intensity, duration, and weekly frequency were increased gradually across the first month until participants were walking 30 min per session (4 days per week). During the remaining (5–12) weeks, the exercise intensity was targeted at 50–60% of HRR and exercise duration was 30 min (a total of 44 sessions for 12 weeks), plus a 10-min warm-up and 10-min cool-down (50 min total). The treadmill speed and grade were modified each session based on each participant’s progress and exercise capacity. The proportion of sessions attended was used to assess compliance to the intervention protocol.
MRI acquisition
MRI data (3.0 Tesla (GE, Waukesha, WI) were acquired at high-resolution with T1-weighted anatomical images for co-registration using the following parameters: 3D Spoiled Gradient Recalled at steady state protocol (SPGR), slice thickness = 1 mm, voxel size = 0.94×0.94×1.00 mm, number of excitations (NEX) = 1, repetition time (TR) = 9.6 ms, field of view = 240 mm, echo time (TE) = 3.9 ms, inversion recovery preparation time = 450 ms, flip angle = 12°, resolution = 256×224, and sequence duration = 6 min. Resting state fMRI data were collected with eyes open (with a fixation cross) using the following standard parameters: gradient echo planar images (6 min acquisition time, 36 slices, axial plane), 4.0 mm isotropic voxels, field of view = 240 mm, slice thickness = 1.0 mm, TR/TE = 2000/25 ms, NEX = 1 mm, resolution = 64×64, flip angle = 77°.
Structural and functional MRI data processing
First, FreeSurfer’s (version 5.3.0) automated processing stream (recon-all) was used to process the T1-weighted anatomical volumes, which generate cortical and subcortical reconstructions [41]. Second, to minimize magnetization disequilibrium, we used Analysis of Functional NeuroImages [42] (AFNI, v.17.2.10) 3dTcat function to manually remove the first three volumes of the functional image time-series. Third, Slice-Oriented Motion Correction, which corrects misalignment between consecutive slices, was used to realign the truncated functional images [43]. Next, the motion-corrected functional volumes and FreeSurfer-rendered anatomical images were coregistered using AFNI’s align_epi_anat function. We visually inspected for proper alignment and no further correction was administered. AFNI’s single-subject preprocessing stream (proc.py) was used to further process the coregistered functional volumes that include: 1) censoring volumes with outlier fraction threshold (>10%), 2) despiking (3dDespike) the remaining time-series to reduce high-intensity transients within the blood oxygen level dependent (BOLD) signal, 3) time-shifting to the beginning of the TR (3dTshift), 4) bandpass filtering (0.01 to 0.1 Hz), 5) censoring image volumes if their frame-wise displacement was greater than 0.2 mm, and 6) removing signals from ventricles and white matter to further reduce physiological noise. The proc.py script resulted in the transformation matrix with a grid spacing of 2 mm3. Since there was no difference in the percentage of censored TRs between the before (0.86±0.27%) and after ET scans (0.94±0.34%) (p = 0.09; paired t-test), we did not use head movement as a covariate in the subsequent analyses.
Construction of functional connectivity networks
We used the brain atlas developed by Power et al. [44] to define functional network nodes, which were created based on meta-analytic and FC mapping techniques. The Power et al. atlas contains a total of 264 regions of interest widely distributed across cortical, subcortical, and cerebellar brain regions. These regions of interest were modeled as spherical nodes representing individual elements of large-scale brain organization. Among all brain networks, we specifically selected three core large-scale networks, the DMN, FPN, and SAL, based on the triple network model [32]. We first created spherical regions of interest positioned on each of the coordinates provided by the atlas developed by Power and colleagues (2011) [44] in MNI space (10 mm in diameter) (Fig. 1). We then extracted the average residual BOLD signal within each network node to calculate network edges for each participant and each experimental time point (e.g., before and after ET). Edge weights of subject-specific networks were defined as the Fisher’s r-to-z transformed Pearson product-moment cross-correlations of the average BOLD time series calculated between network nodes.
Within-network and between-network functional connectivity
Fig. 1
Location of the nodes for each network defined by Power (2011) atlas [44]. Red: DMN (59 nodes); Yellow: FPN (25 nodes); and Black: SAL (18 nodes). Detailed coordinates for each node are presented in Supplementary Table 1.
![Location of the nodes for each network defined by Power (2011) atlas [44]. Red: DMN (59 nodes); Yellow: FPN (25 nodes); and Black: SAL (18 nodes). Detailed coordinates for each node are presented in Supplementary Table 1.](https://content.iospress.com:443/media/adr/2023/7-1/adr-7-1-adr220062/adr-7-adr220062-g001.jpg)
Average FC was calculated within and between the DMN, FPN, and SAL. Within-network FC was calculated for each participant as the average of all edges (nij) within the DMN (DMNW), FPN (FPNW), and SAL (SALW). Between-network FC was calculated as the average of all edges between nodes of the DMN and FPN (DMN-FPNB), between nodes of the DMN and SAL (DMN-SALB), and between nodes of the FPN and SAL (FPN-SALB). We retained negative edges in adjacency matrices for the present study; thus an undirected, signed, and weighted matrix was created for each participant for each time point (i.e., before and after ET). Although a statistical threshold is commonly applied to correlation matrices in FC analyses in order to retain the most reliable positive connections, the present study did not use a threshold for the adjacency matrices due to the potential neurophysiological relevance of weak and negatively correlated FC in the whole-brain network topology [45, 46]. The group-averaged adjacency matrices representing the DMN, FPN, and SAL for each time point (i.e., before- and after-ET) are presented in Fig. 2.
Statistical analysis
Fig. 2
Group-averaged (CN and MCI) adjacency matrix representing functional connectivity of DMN, FPN, and SAL defined using Power (2011) atlas [44]. A) Before exercise training. B) After exercise training. C) After minus Before exercise training.
![Group-averaged (CN and MCI) adjacency matrix representing functional connectivity of DMN, FPN, and SAL defined using Power (2011) atlas [44]. A) Before exercise training. B) After exercise training. C) After minus Before exercise training.](https://content.iospress.com:443/media/adr/2023/7-1/adr-7-1-adr220062/adr-7-adr220062-g002.jpg)
The baseline demographic and cognitive test performance differences between groups (MCI versus CN) were analyzed using independent sample t-tests (or Wilcoxon signed-rank tests for non-parametric data) or Fisher’s exact test for discrete data [e.g., number of female participants or apolipoprotein E epsilon-4 allele (APOE ɛ4) carrier] after determining normality using the Shapiro-Wilk test. Since no alternate forms were used for the LM test, we computed residualized change scores for the LM test to minimize practice effects and regression to the mean [47, 48]. Repeated measures ANOVAs were used to compute the main effects of Time (i.e., before versus after ET), Group (i.e., MCI versus CN), and the Group×Time interaction on the functional network connectivity metrics and cognitive test performance outcomes. The associations between ET-related changes in cognitive performance (Δcognitive performance) and within and between network connectivity FC (Δwithin-network FC and Δbetween-network FC, respectively) were then examined using partial correlation analysis. We conducted bivariate correlation tests to evaluate relationships between age and variables of interest including FC and cognitive performance prior to the partial correlation analysis. We found no significant correlations between age and variables of interest (p≥0.209). We also did not find significant correlations between sex and variables of interest (p≥0.146). Therefore, the correlation analyses were unadjusted for age and sex [49, 50]. Statistical significance was determined at alpha = 0.05. All statistical tests were conducted using SPSS (v. 26.0, IBM, Armonk, NY).
RESULTS
Demographic characteristics
Data from the sample used in the study have been previously published examining different outcomes and sub-samples [34]. Briefly, advertisements for recruitment yielded 407 respondents; 92 were consented and underwent neurological examination, 68 were scheduled for pre-test assessments, 39 began exercise intervention, and 35 completed all study procedures. Of the 35 participants who completed the entire study protocol, 2 individuals (1 MCI and 1 CN) were excluded due to missing fMRI data. The remaining 33 participants were included in the present analyses (16 MCI and 17 CN; mean age = 78 years). Of the 16 MCI participants, six were classified as amnestic MCI (i.e., impairment specifically in the memory domain; detailed information about recruitment and enrollment is described in our previous work [34]). 31 participants were Caucasians and two were African Americans. Formal education averaged 16 years and 72% were women. 11 participants were APOE ɛ4 carriers. The CN group demonstrated a significantly greater Mattis Dementia Rating Scale-2 score compared to MCI (p = 0.001), as expected. In contrast, there were no significant differences between the MCI and CN groups in the baseline demographic characteristics including age, sex, education, number of APOE ɛ4 carriers,
Table 1
Demographic information for study participants
Total Sample | MCI | CN | Group Differences | |
(n = 33) | (n = 16) | (n = 17) | ||
Mean±SD | Mean±SD | Mean±SD | p | |
Demographics | ||||
Age (y) | 78.0±7.0 | 80.5±5.7 | 75.8±7.4 | 0.223 |
Female (n, %) | 24 (72.7%) | 10 (62.5%) | 14 (82.3%) | 0.201F |
Education (y) | 16.0±2.5 | 15.6±3.2 | 16.5±1.9 | 0.332 |
APOE ɛ4 Carriers (n, %) | 11 (33.3%) | 5 (31.2%) | 6 (35.2%) | 0.622F |
Cardiorespiratory Fitness | ||||
Baseline | 19.4±4.5 | 18.6±3.7 | 20.1±5.1 | 0.258 |
Depression | ||||
Baseline GDS | 4.6±3.3 | 6.0±3.7 | 3.8±2.7 | 0.074 |
Cognitive Function | ||||
Baseline Mattis Dementia Rating Scale-2 Total | 134.3±11.1 | 128.0±13.3 | 140.3±2.5 | 0.001 |
Activities of Daily Living | ||||
Baseline Lawton Activities of Daily Living | 4.6±0.5 | 4.6±0.5 | 4.6±0.6 | 0.910 |
MCI, mild cognitive impairment; CN, normal cognition control; F, Fisher’s Exact Test; APOE ɛ4, apolipoprotein E epsilon 4 allele;
Exercise intervention
The mean number of exercise sessions completed was 42.3±2.2 out of 44 total sessions, and the adherence rate was 96.1±5.0%. The mean intensity of the exercise session during the first four weeks was 46.9±7.1% HRR and during the weeks 5–12 was 54.7±11.0% HRR and the mean rating of perceived exertion (RPE) was 10.6±1.8 and 10.8±2.0 (light exertion), respectively [34].
Cardiorespiratory fitness and cognitive task performance
There was a significant increase in
No significant Group×Time interactions were found for cognitive performance, including LM immediate recall [F(1,28) = 0.194, p = 0.663,
Table 2
Cognitive task outcome data for study participants
Total Sample (n = 33) | MCI (n = 16) | CN (n = 17) | Time | Group | Group×Time | ||||
Before | After | Before | After | Before | After | ||||
Mean±SD | Mean±SD | Mean±SD | Mean±SD | Mean±SD | Mean±SD | p (
| p (
| p (
| |
Cardiorespiratory Fitness | |||||||||
| 19.38±4.50 | 21.10±3.70 | 18.59±3.68 | 21.20±3.24 | 20.07±5.14 | 21.02±4.16 | 0.005 (0.248) | 0.647 (0.008) | 0.166 (0.067) |
Cognitive Function | |||||||||
LM Immediate Recall | 36.27±13.39 | 39.23±13.51 | 30.60±14.23 | 34.07±15.89 | 41.93±9.99 | 44.40±8.26 | 0.014 (0.196) | 0.021 (0.177) | 0.663 (0.007) |
LM Delayed Recall | 21.57±10.14 | 23.63±10.63 | 17.80±10.18 | 19.47±10.80 | 25.33±8.89 | 27.80±8.94 | 0.039 (0.143) | 0.028 (0.161) | 0.679 (0.006) |
LM Recognition | 24.23±3.40 | 25.53±3.47 | 22.47±3.06 | 24.20±3.29 | 26.00±2.80 | 26.87±3.20 | 0.006 (0.242) | 0.006 (0.239) | 0.327 (0.034) |
RAVLT Trial 1 | 4.67±2.08 | 5.63±1.93 | 4.31±1.88 | 5.60±2.09 | 5.00±2.26 | 5.67±1.83 | 0.035 (0.149) | 0.478 (0.018) | 0.278 (0.042) |
RAVLT Trial 1–5 | 43.23±13.77 | 44.87±15.04 | 37.80±13.44 | 40.47±15.62 | 48.67±12.21 | 49.27±13.53 | 0.282 (0.041) | 0.050 (0.130) | 0.494 (0.017) |
RAVLT Immediate Recall | 8.60±4.46 | 8.93±4.35 | 6.80±4.09 | 7.60±4.73 | 10.40±4.20 | 10.27±3.61 | 0.386 (0.027) | 0.043 (0.138) | 0.227 (0.052) |
RAVLT Delayed Recall | 8.30±4.69 | 8.60±4.66 | 6.60±4.43 | 7.00±4.76 | 10.00±4.45 | 10.20±4.10 | 0.584 (0.011) | 0.040 (0.142) | 0.855 (0.001) |
COWAT | 36.60±12.47 | 39.40±14.34 | 33.07±13.64 | 38.47±16.84 | 40.13±10.46 | 40.33±12.47 | 0.041 (0.141) | 0.056 (0.124) | 0.352 (0.031) |
MCI, mild cognitive impairment; CN, normal cognition control;
Table 3
Within- and between-network data for study participants
Total Sample (n = 33) | MCI (n = 16) | CN (n = 17) | Time | Group | Group×Time | ||||
Before | After | Before | After | Before | After | ||||
Mean±SD | Mean±SD | Mean±SD | Mean±SD | Mean±SD | Mean±SD | p (
| p (
| p (
| |
Within-Network Connectivity | |||||||||
DMNW | 0.079±0.027 | 0.103±0.037 | 0.082±0.024 | 0.092±0.035 | 0.077±0.031 | 0.113±0.037 | 0.008 (0.204) | 0.323 (0.031) | 0.125 (0.074) |
FPNW | 0.112±0.054 | 0.128±0.044 | 0.118±0.053 | 0.127±0.053 | 0.105±0.056 | 0.130±0.036 | 0.143 (0.068) | 0.714 (0.004) | 0.466 (0.017) |
SALW | 0.099±0.036 | 0.145±0.062 | 0.097±0.040 | 0.115±0.062 | 0.100±0.033 | 0.145±0.062 | 0.013 (0.183) | 0.221 (0.048) | 0.275 (0.038) |
Between-Network Connectivity | |||||||||
DMN-FPNB | 0.004±0.008 | 0.019±0.266 | 0.005±0.006 | 0.018±0.027 | 0.002±0.010 | 0.019±0.026 | 0.006 (0.219) | 0.861 (0.001) | 0.588 (0.010) |
DMN-SALB | 0.009±0.013 | 0.026±0.030 | 0.012±0.014 | 0.024±.034 | 0.006±0.012 | 0.028±0.027 | 0.004 (0.237) | 0.846 (0.001) | 0.376 (0.025) |
FPN-SALB | 0.018±0.022 | 0.040±0.040 | 0.022±0.021 | 0.041±0.045 | 0.014±0.023 | 0.039±0.035 | 0.013 (0.184) | 0.572 (0.010) | 0.694 (0.005) |
MCI, mild cognitive impairment; CN, normal cognition control; p-values and effect size (
Within- and between-network connectivity
In response to ET, significantly increased within-network connectivity occurred from before to after ET for the DMN (DMNW) [F(1,31) = 7.963, p = 0.008,
Associations between ET-related changes in network connectivity and cognitive performance
Across participants, ET-related changes in the SAL within-network connectivity (ΔSALW) explained 22.1% of the variance in ΔLM immediate recall residualized change score [R = 0.471, R2 = 0.221, p = 0.009] (Fig. 3A). No significant relationships were observed between the within-network connectivity measures and other cognitive measures including the RAVLT T1, COWAT, LM delayed recall, or LM recognition performance (p≥0.063). For the between network connectivity measures, the ΔFPN-SALB explained 30.1% of the variance in ΔLM immediate recall residualized change score [R = 0.548, R2 = 0.301, p = 0.001] (Fig. 3B). There were no other significant associations between between-network connectivity measures and other cognitive assessments (p≥0.056).
Fig. 3
A) Positive association of change in the salience network within-network connectivity and changes in logical memory immediate recall performance across participants (both MCI and CN). B) Positive associations of FPN-SAL between-network connectivity and logical memory immediate recall performance across participants (both MCI and CN). Dotted curves indicate 95% confidence interval around the mean.
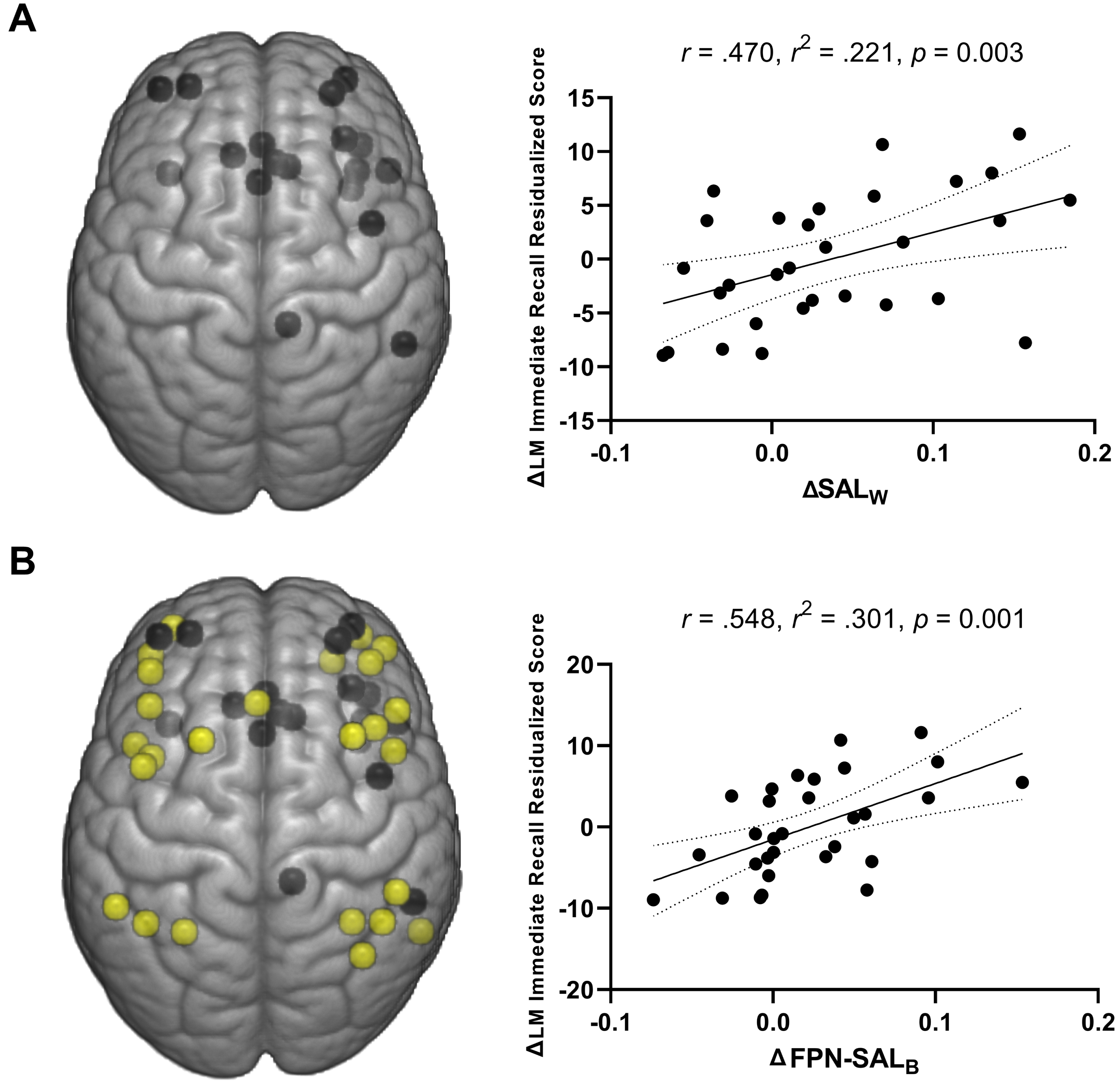
DISCUSSION
The present study examined the effects of a 12-week walking ET intervention on the within- and between-network FC of the major brain functional networks (i.e., DMN, FPN, and SAL) and their associations with cognitive performance in cognitively normal older adults and those diagnosed with MCI. Our results indicate that participation in moderate-intensity walking exercise 4 days per week for 12 weeks resulted in a 10.5% enhancement in cardiorespiratory fitness (
In line with our hypothesis, our finding suggests that a 12-week ET is associated with increased DMN within-network FC. This result is consistent with previous cross-sectional studies suggesting an association between higher cardiorespiratory fitness and greater DMNW [51, 52]. Moreover, the present result is consistent with our previous work using the same cohort that demonstrated an increased DMN FC in response to ET (seed region: posterior cingulate cortex) [22]. Similarly, the ET-induced increase in SALW in this study is in agreement with a previous study suggesting increased anterior insula FC (i.e., a key node of the SAL) in older adults after a 6-month aerobic ET intervention that was paired with regular intake of a nutritional supplement [53]. Importantly, we found that the ET-related increase in SALW was associated with enhanced LM immediate recall performance. The association between the ET-related increase in within-network FC and LM immediate recall performance suggests that strengthened FC within the network may serve as one underlying neurophysiological mechanism of ET-related cognitive improvements. These findings support the hypothesis that ET may enhance the within-network functional connections, which may subserve improved cognitive function in older adults with normal cognition and older adults diagnosed with MCI. On the other hand, we did not find significant changes in FPNW in response to ET, which is consistent with Voss et al. (2010) that showed no overall effects after a 12-month walking exercise [18]. Nevertheless, although the effect size was smaller, the direction of the ET-related effects on FPNW was consistent with DMNW and SALW in this study; thus, the effects of ET on FPN should be further investigated in the future with larger sample.
In contrast to our hypothesis, ET led to increased FC between the DMN, FPN, and SAL. Our results are in partial contrast to the findings reported by Voss and colleagues (2016) in which CRF was negatively associated with the strength of between-network FC [51]. One possible reason for the conflicting results is the difference in the selection of functional networks; the present study selected the nodes based on the work by Power et al. [44], while Voss et al. (2016) selected the nodes based on previous findings from their group [18, 52]. Other possible factors for the disagreement include the differences in the study design (intervention versus cross-sectional), sample characteristic (cognitively impaired and intact older adults versus healthy younger and older adults), and fMRI preprocessing approach (nuisance regression versus global signal regression (minimizing between network FC than nuisance regression)).
Nevertheless, the literature consistently points to increasing between-network FC in the aging brain [54]. It has been hypothesized that the age-related increase in network FC reflects recruitment of broader neural resources to compensate for adverse age-related changes in the brain. While the compensatory mechanism may be indicative of distressed networks, the adaptation to increase communication across networks may enable brain function and behavior to be maintained in the face of age- and pathology-related neurodegeneration [55]. Indeed, a greater compensatory response in the aging brain has been hypothesized to promote preserved cognitive function for a longer period of time [56, 57]. Given that we found an ET-related increase in between-network FC and corresponding improvements in cognitive performance, the ET-related increases in between-network FC may reflect a compensatory reorganization of functional networks involving broader network interactions in the aging brain. Another potential mechanism underpinning the current findings is that ET-related improvements in vascular brain function including enhanced blood flow, greater blood volume, better neuro-vascular coupling may have influenced the BOLD response, which in turn would affect measures of FC [58–60]. In support of these hypotheses, our previous work using the same cohort also consistently showed an ET-related increase in FC [20, 22, 23, 61] and corresponding improvements in cognitive function [20, 23]. Of note, the ET-related increase in the between-network FC was observed in all the core networks (i.e., DMN-FPNB, DMN-SALB, and FPN-SALB) in both CN and MCI participants, suggesting broader effects elicited by ET on compensatory responses between major brain networks.
Indeed, we observed no significant Group (CN versus MCI) by Time (before-ET versus after-ET) interaction effects on the within- and between-network connectivity changes. This contrasts with our previous report using the same cohort in which we found (using a seed-based analysis) a significant Group by Time interaction effect on DMN FC. Specifically, our previous study demonstrated an ET-related increase in FC between the posterior cingulate cortex and right inferior parietal lobule in older adults with MCI, while the CN group demonstrated an ET-related decrease in the FC between the posterior cingulate cortex and right inferior parietal lobule [22]. In the present study, however, the Group by Time interaction for the DMNW was not significant (p = 0.125), which presumably attributes to differences in the analytic approach (i.e., connectivity within the network versus connectivity between seed and rest of the brain). Further, due to the relatively small sample size (N = 33; CN = 16; MCI = 17), the present study should be replicated in the future with a larger sample size to conclusively test whether or not cognitive status is an important moderator on the effects of ET on large-scale brain network FC.
Lastly, we found that ET-related changes in the within- and between-network connectivity were consistently associated with LM memory performance. Specifically, within- and between-network connectivity of the SAL has been associated with LM immediate recall, which reflects episodic memory performance. Although there is relatively little evidence regarding the direct association between the SAL and LM performance, the implication of SAL on working memory has been established [62]. Specifically, it has been suggested that the SAL plays a role in transforming salience signals to engage in a task-relevant neurophysiological response, while disengaging from responses that are not relevant to the task [62]. With this, it has been speculated that the SAL engages in maintenance and retrieval of the information, an essential aspect of effective working memory performance [63]. Notably, successful performance of the LM immediate recall task requires encoding, maintenance, and retrieval of the information since participants had to recall short stories immediately after listening to them, which is associated with working memory processes [64]. Therefore, engagement of the SAL in maintenance and retrieval of information may corroborate the association between the ET-related alterations in the within- and between-network changes in the SAL and improvements in the LM immediate recall performance after ET. Indeed, it has been previously suggested that greater network FC between the FPN and DMN is associated with better memory performance [65] and the present study adds to the prior finding with evidence that the greater interconnectivity between the FPN and SAL are associated with improved memory performance.
Strengths and limitations
One major strength of the present study is using a large network-level analysis. Beyond a seed-based approach, the within- and between-network analysis helps to broaden our understanding about the potential role of ET in delaying the onset of age-related cognitive decline and Alzheimer’s disease. In addition, our participants demonstrated a high compliance rate (96%) to the walking exercise intervention. We hypothesize that the high compliance rate played an important role in significantly enhancing cardiorespiratory fitness (10.5% increase in
Conclusion
The current findings elucidate the salutary effects of walking exercise on communication within and between large brain functional networks, beyond our well-established understanding on the effects of ET on regional or task-specific brain networks. Notably, the ET-related changes in network FC and memory performance were observed in both groups (CN and MCI), indicating that ET-induced effects on brain network FC may occur in older individuals who have experienced clinically relevant cognitive decline. These findings suggest regular participation in simple aerobic exercise like moderate intensity walking may induce neuroplastic effects even in the face of Alzheimer’s disease-related neurodegenerative processes that have resulted in a diagnosis of MCI.
ACKNOWLEDGMENTS
We thank the participants for their dedication while participating in this study, Drs. Nathan Hantke and Alissa Butts for their assistance with neuropsychological assessment, and Dr. Piero Antuono for his role in diagnosis of MCI.
FUNDING
This study was supported by the University of Wisconsin-Milwaukee Graduate School Research Growth Initiative; and the National Center for Advancing Translational Sciences, NIH grant numbers 8UL1TR000055, 8KL2TR000056. The content is solely the responsibility of the authors and does not necessarily represent the official views of the NIH.
CONFLICT OF INTEREST
The authors have no conflict of interest to report.
DATA AVAILABILITY
The data will be made available in csv format upon request.
SUPPLEMENTARY MATERIAL
[1] The supplementary material is available in the electronic version of this article: https://dx.doi.org/10.3233/ADR-220062.
REFERENCES
[1] | Murman DL The impact of age on cognition. Semin Hear ((2015) ) 36: , 111–121. |
[2] | Fjell AM , Walhovd KB , Fennema-Notestine C , McEvoy LK , Hagler DJ , Holland D , Brewer JB , Dale AM One-year brain atrophy evident in healthy aging. J Neurosci ((2009) ) 29: , 15223–15231. |
[3] | Jack CR , Petersen RC , Xu Y , O’brien PC , Smith GE , Ivnik RJ , Boeve BF , Tangalos EG , Kokmen E Rates of hippocampal atrophy correlate with change in clinical status in aging and AD. Neurology ((2000) ) 55: , 484–490. |
[4] | Tarumi T , Zhang R Cerebral blood flow in normal aging adults: Cardiovascular determinants, clinical implications, and aerobic fitness. J Neurochem ((2018) ) 144: , 595–608. |
[5] | Callaghan MF , Freund P , Draganski B , Anderson E , Cappelletti M , Chowdhury R , Diedrichsen J , FitzGerald TH , Smittenaar P , Helms G Widespread age-related differences in the human brain microstructure revealed by quantitative magnetic resonance imaging. Neurobiol Aging ((2014) ) 35: , 1862–1872. |
[6] | Fox MD , Snyder AZ , Vincent JL , Corbetta M , Van Essen DC , Raichle ME The human brain is intrinsically organized into dynamic, anticorrelated functional networks. Proc Natl Acad Sci U S A ((2005) ) 102: , 9673–9678. |
[7] | Damoiseaux JS , Rombouts S , Barkhof F , Scheltens P , Stam CJ , Smith SM , Beckmann CF Consistent resting-state networks across healthy subjects. Proc Natl Acad Sci U S A ((2006) ) 103: , 13848–13853. |
[8] | Buckner RL , Andrews-Hanna JR , Schacter DL ((2008) ) The brain’s default network: Anatomy, function, and relevance to disease. Ann N Y Acad Sci 1124: , 1–38. |
[9] | Yeo BT , Krienen FM , Sepulcre J , Sabuncu MR , Lashkari D , Hollinshead M , Roffman JL , Smoller JW , Zöllei L , Polimeni JR ((2011) ) The organization of the human cerebral cortex estimated by intrinsic functional connectivity. J Neurophysiol 106: , 1125–1165. |
[10] | Andrews-Hanna JR , Smallwood J , Spreng RN ((2014) ) The default network and self-generated thought: Component processes, dynamic control, and clinical relevance. Ann N Y Acad Sci 1316: , 29–52. |
[11] | Seeley WW , Menon V , Schatzberg AF , Keller J , Glover GH , Kenna H , Reiss AL , Greicius MD ((2007) ) Dissociable intrinsic connectivity networks for salience processing and executive control. J Neurosci 27: , 2349–2356. |
[12] | Goulden N , Khusnulina A , Davis NJ , Bracewell RM , Bokde AL , McNulty JP , Mullins PG ((2014) ) The salience network is responsible for switching between the default mode network and the central executive network: Replication from DCM. Neuroimage 99: , 180–190. |
[13] | Mevel K , Chételat G , Eustache F , Desgranges B ((2011) ) The default mode network in healthy aging andAlzheimer’s disease. Int J Alzheimers Dis 2011: , 535816. |
[14] | Campbell KL , Grady CL , Ng C , Hasher L ((2012) ) Age differences in the frontoparietal cognitive control network: Implications for distractibility. Neuropsychologia 50: , 2212–2223. |
[15] | Lee T-H , Kim SH , Katz B , Mather M ((2020) ) The decline in intrinsic connectivity between the salience network and locus coeruleus in older adults: Implications for distractibility. Front Aging Neurosci 12: , 2. |
[16] | Ibrahim B , Suppiah S , Ibrahim N , Mohamad M , Hassan HA , Nasser NS , Saripan MI ((2021) ) Diagnostic power of resting-state fMRI for detection of network connectivity in Alzheimer’s disease and mild cognitive impairment: A systematic review. Hum Brain Mapp 42: , 2941–2968. |
[17] | Won J , Callow DD , Pena GS , Gogniat MA , Kommula Y , Arnold-Nedimala NA , Jordan LS , Smith JC ((2021) ) Evidence for exercise-related plasticity in functional and structural neural network connectivity. Neurosci Biobehav Rev 131: , 923–940. |
[18] | Voss MW , Prakash RS , Erickson KI , Basak C , Chaddock L , Kim JS , Alves H , Heo S , Szabo A , White SM ((2010) ) Plasticity of brain networks in a randomized intervention trial of exercise training in older adults. Front Aging Neurosci 2: , 32. |
[19] | Tozzi L , Carballedo A , Lavelle G , Doolin K , Doyle M , Amico F , McCarthy H , Gormley J , Lord A , O’Keane V ((2016) ) Longitudinal functional connectivity changes correlate with mood improvement after regular exercise in a dose-dependent fashion. Eur J Neurosci 43: , 1089–1096. |
[20] | Won J , Faroqi-Shah Y , Callow DD , Williams A , Awoyemi A , Nielson KA , Smith JC ((2021) ) Association between greater cerebellar network connectivity and improved phonemic fluency performance after exercise training in older adults. Cerebellum 20: , 542–555. |
[21] | Petersen RC ((2000) ) Mild cognitive impairment: Transition between aging and Alzheimer’s disease. Neurologia 15: , 93–101. |
[22] | Chirles TJ , Reiter K , Weiss LR , Alfini AJ , Nielson KA , Smith JC ((2017) ) Exercise training and functional connectivity changes in mild cognitive impairment and healthy elders. J Alzheimers Dis 57: , 845–856. |
[23] | Won J , Callow DD , S Pena G , Jordan LS , Arnold-Nedimala NA , Nielson KA , Smith JC ((2021) ) Hippocampal functional connectivity and memory performance after exercise intervention in older adults with mild cognitive impairment. J Alzheimers Dis 82: , 1015–1031. |
[24] | Cole DM , Smith SM , Beckmann CF ((2010) ) Advances and pitfalls in the analysis and interpretation of resting-state FMRI data. Front Syst Neurosci 4: , 8. |
[25] | Lv H , Wang Z , Tong E , Williams LM , Zaharchuk G , Zeineh M , Goldstein-Piekarski AN , Ball TM , Liao C , Wintermark M ((2018) ) Resting-state functional MRI: Everything that nonexperts have always wanted to know. Am J Neuroradiol 39: , 1390–1399. |
[26] | Dorsman KA , Weiner-Light S , Staffaroni AM , Brown JA , Wolf A , Cobigo Y , Walters S , Kramer JH , Casaletto KB ((2020) ) Get moving! Increases in physical activity are associated with increasing functional connectivity trajectories in typically aging adults. Front Aging Neurosci 12: , 104. |
[27] | Ruff RM , Light RH , Parker SB , Levin HS ((1996) ) Benton controlled oral word association test: Reliability and updated norms. Arch Clin Neuropsychol 11: , 329–338. |
[28] | Rey A ((1958) ) L’examen clinique en psychologie. Presses Universitaries De France. |
[29] | Sullivan K ((2005) ) Alternate forms of prose passages for the assessment of auditory–verbal memory. Arch Clin Neuropsychol 20: , 745–753. |
[30] | Wechsler D ((1945) ) Wechsler Memory Scale. Psychological Corporation. |
[31] | Callow DD , Won J , Pena GS , Jordan LS , Arnold-Nedimala NA , Kommula Y , Nielson KA , Smith JC ((2021) ) Exercisetraining-related changes in cortical gray matter diffusivity and cognitive function in mild cognitive impairmentand healthy older adults. Front Aging Neurosci 13: , 645258. |
[32] | Menon V ((2011) ) Large-scale brain networks and psychopathology: A unifying triple network model. Trends Cogn Sci 15: , 483–506. |
[33] | Varangis E , Habeck CG , Razlighi QR , Stern Y ((2019) ) The effect of aging on resting state connectivity of predefined networks in the brain. Front Aging Neurosci 11: , 234. |
[34] | Smith JC , Nielson KA , Antuono P , Lyons J-A , Hanson RJ , Butts AM , Hantke NC , Verber MD ((2013) ) Semantic memory functional MRI and cognitive function after exercise intervention in mild cognitive impairment. J Alzheimers Dis 37: , 197–215. |
[35] | Yesavage JA ((1988) ) Geriatric depression scale. Psychopharmacol Bull 24: , 709–711. |
[36] | Lawton MP , Brody EM ((1969) ) Assessment of older people: Self-maintaining and instrumental activities of daily living. Gerontologist 9: , 179–186. |
[37] | Oldfield RC ((1971) ) The assessment and analysis of handedness: The Edinburgh inventory. Neuropsychologia 9: , 97–113. |
[38] | Albert MS , DeKosky ST , Dickson D , Dubois B , Feldman HH , Fox NC , Gamst A , Holtzman DM , Jagust WJ , Petersen RC ((2011) ) The diagnosis of mild cognitive impairment due to Alzheimer’s disease: Recommendations from the National Institute on Aging-Alzheimer’s Association workgroups on diagnostic guidelines for Alzheimer’s disease. Alzheimers Dement 7: , 270–279. |
[39] | Pescatello LS , American College of Sports Medicine ((2013) ) ACSM’s Guidelines for Exercise Testing and Prescription, 9th edition. Lippincott Williams & Wilkins, Philadelphia. |
[40] | Borg G ((1998) ) Borg’s perceived exertion and pain scales. Human Kinetics. |
[41] | Fischl B ((2012) ) FreeSurfer. Neuroimage 62: , 774–781. |
[42] | Cox RW ((1996) ) AFNI: Software for analysis and visualization of functional magnetic resonance neuroimages. Comput Biomed Res 29: , 162–173. |
[43] | Beall EB , Lowe MJ ((2014) ) SimPACE: Generating simulated motion corrupted BOLD data with synthetic-navigated acquisition for the development and evaluation of SLOMOCO: A new, highly effective slicewise motion correction. Neuroimage 101: , 21–34. |
[44] | Power JD , Cohen AL , Nelson SM , Wig GS , Barnes KA , Church JA , Vogel AC , Laumann TO , Miezin FM , Schlaggar BL ((2011) ) Functional network organization of the human brain. Neuron 72: , 665–678. |
[45] | Chen T , Cai W , Ryali S , Supekar K , Menon V ((2016) ) Distinct global brain dynamics and spatiotemporal organization of the salience network. PLoS Biol 14: , e1002469. |
[46] | Sporns O , Betzel RF ((2016) ) Modular brain networks. Annu Rev Psychol 67: , 613–640. |
[47] | Llabre MM , Spitzer SB , Saab PG , Ironson GH , Schneiderman N ((1991) ) The reliability and specificity of delta versus residualized change as measures of cardiovascular reactivity to behavioral challenges. Psychophysiology 28: , 701–711. |
[48] | Temkin NR , Heaton RK , Grant I , Dikmen SS ((1999) ) Detecting significant change in neuropsychological test performance: A comon of four models. J Int Neuropsychol Soc 5: , 357–369 paris. |
[49] | Adams KM , Brown GG , Grant I ((1985) ) Analysis of covariance as a remedy for demographic mismatch of research subject groups: Some sobering simulations. J Clin Exp Neuropsychol 7: , 445–462. |
[50] | Miller GA , Chapman JP ((2001) ) Misunderstanding analysis of covariance. J Abnorm Psychol 110: , 40. |
[51] | Voss MW , Weng TB , Burzynska AZ , Wong CN , Cooke GE , Clark R , Fanning J , Awick E , Gothe NP , Olson EA ((2016) ) Fitness, but not physical activity, is related to functional integrity of brain networks associated with aging. Neuroimage 131: , 113–125. |
[52] | Voss MW , Erickson KI , Prakash RS , Chaddock L , Malkowski E , Alves H , Kim JS , Morris KS , White SM , Wójcicki TR ((2010) ) Functional connectivity: A source of variance in the association between cardiorespiratory fitness and cognition? Neuropsychologia 48: , 1394–1406. |
[53] | Voss MW , Sutterer M , Weng TB , Burzynska AZ , Fanning J , Salerno E , Gothe NP , Ehlers DK , McAuley E , Kramer AF ((2019) ) Nutritional supplementation boosts aerobic exercise effects on functional brain systems. J Appl Physiol 126: , 77–87. |
[54] | Iordan AD , Cooke KA , Moored KD , Katz B , Buschkuehl M , Jaeggi SM , Jonides J , Peltier SJ , Polk TA , Reuter-Lorenz PA ((2018) ) Aging and network properties: Stability over time and links with learning during working memory training. Front Aging Neurosci 9: , 419. |
[55] | Park DC , Reuter-Lorenz P Annu Rev Psychol ((2009) ) The adaptive brain:Aging and neurocognitive scaffolding. Annu Rev Psychol 60: , 173–196. |
[56] | Kayes MK , Hatfield BD ((2019) ) The influence of physical activity on brain aging and cognition: The role of cognitive reserve, thresholds for decline, genetic influence, and the investment hypothesis. In Lifestyle Medicine, Third Edition, Rippe JM, ed. CRC Press, Boca Raton, pp. 1251–1269. |
[57] | Rao SM , Bonner-Jackson A , Nielson KA , Seidenberg M , Smith JC , Woodard JL , Durgerian S ((2015) ) Genetic risk for Alzheimer’s disease alters the five-year trajectory of semantic memory activation in cognitively intact elders. Neuroimage 111: , 136–146. |
[58] | Bright MG , Whittaker JR , Driver ID , Murphy K ((2020) ) Vascular physiology drives functional brain networks. Neuroimage 217: , 116907. |
[59] | Köbe T , Binette AP , Vogel JW , Meyer P-F , Breitner JC , Poirier J , Villeneuve S ((2021) ) Vascular risk factors are associated with a decline in resting-state functional connectivity in cognitively unimpaired individuals at risk for Alzheimer’s disease: Vascular risk factors and functional connectivity changes. Neuroimage 231: , 117832. |
[60] | Chong JSX , Jang H , Kim HJ , Ng KK , Na DL , Lee JH , Seo SW , Zhou J ((2019) ) Amyloid and cerebrovascular burden divergently influence brain functional network changes over time. Neurology 93: , e1514–e1525. |
[61] | Won J , Nielson KA , Smith JC ((2022) ) Subjective well-being and bilateral anterior insula functional connectivity after exercise intervention in older adults with mild cognitive impairment. Front Neurosci 16: , 834816. |
[62] | Sridharan D , Levitin DJ , Menon V ((2008) ) A critical role for the right fronto-insular cortex in switching between central-executive and default-mode networks. Proc Natl Acad Sci U S A 105: , 12569–12574. |
[63] | Santangelo V , Bordier C ((2019) ) Large-scale brain networks underlying successful and unsuccessful encoding, maintenance, and retrieval of everyday scenes in visuospatial working memory. Front Psychol 10: , 233. |
[64] | Johnstone E , Cunningham-Owens DG , Lawrie S ((2010) ) Companion to psychiatric studies e-book, Health Sciences. |
[65] | Grady C , Sarraf S , Saverino C , Campbell K ((2016) ) Age differences in the functional interactions among the default, frontoparietal control, and dorsal attention networks. Neurobiol Aging 41: , 159–172. |
[66] | Castro-Schilo L , Grimm KJ ((2018) ) Using residualized change versus difference scores for longitudinal research. J Soc Pers Relat 35: , 32–58. |