Maintaining a Dynamic Brain: A Review of Empirical Findings Describing the Roles of Exercise, Learning, and Environmental Enrichment in Neuroplasticity from 2017-2023
Abstract
Brain plasticity, also termed neuroplasticity, refers to the brain’s life-long ability to reorganize itself in response to various changes in the environment, experiences, and learning. The brain is a dynamic organ capable of responding to stimulating or depriving environments, activities, and circumstances from changes in gene expression, release of neurotransmitters and neurotrophic factors, to cellular reorganization and reprogrammed functional connectivity. The rate of neuroplastic alteration varies across the lifespan, creating further challenges for understanding and manipulating these processes to benefit motor control, learning, memory, and neural remodeling after injury. Neuroplasticity-related research spans several decades, and hundreds of reviews have been written and published since its inception. Here we present an overview of the empirical papers published between 2017 and 2023 that address the unique effects of exercise, plasticity-stimulating activities, and the depriving effect of social isolation on brain plasticity and behavior.
INTRODUCTION
Brain plasticity can be defined as the brain’s capacity for structural and functional reorganization that depends on experience and circuit use. This capacity is heavily governed by the principle “use it or lose it” [1–3] that spans from the intense “use” during development (experience-expectant plasticity) [4] to experience-dependent plasticity in adolescence and adulthood. The capacity for neuroplasticity may be “lost” when circumstances (isolation) or physiological capacities (aging/disease) become unfavorable [5–7].
The field of neuroplasticity emerged from the early findings of neuronal and non-neuronal tissue reorganization after exposure to environmental complexity (also known as environmental enrichment; 8,9). Initially, researchers contended that experience-dependent changes were possible in neural tissues, but that such changes predominantly affected synapse number and maturity. Over the years, our understanding of how the brain matures and adapts to new environments or stimuli has evolved as it is apparent that all components of brain tissue are subject to plasticity, including neuronal proliferation and adaptation of their principal components (synapses, dendrites), glial cells (astrocytes, microglia and myelinating oligoglia) and cerebrovasculature.
Environmental complexity and exercise are arguably the two most powerful plasticity-evoking experiences that are used to study the phenomenon of brain plasticity. However, other experimental paradigms are used to reshape the brain’s structure and function, including complex motor learning (highly skilled movements, acrobat learning), combined intervention and isolation. The goal of this review is to summarize findings from recently published (2017-2023) empirical studies aimed to further understand the mechanisms of brain plasticity and to evaluate their potential as therapeutic interventions.
MECHANISMS OF NEUROPLASTICITY
The molecular and cellular mechanisms underlying neuroplasticity have been topics of study for at least four decades. However, our understanding of these mechanisms is continuously refined with the advancement of technologies to examine changes to the brain’s structure and function in vivo. As reviewed previously [10–12], aerobic activity has been extensively studied as a strong neuroplastic modulator. From these studies, it is evidenced that aerobic activity increases the level of circulating neurotrophins including brain-derived neurotrophic factor (BDNF), nerve growth factor (NGF), vascular endothelial growth factor (VEGF), insulin-like growth factor 1 (IGF-1) and these factors contribute to several cellular alterations in the brain [reviewed in 13]. First, production of VEGF, BDNF, and IGF-1 are increased in response to elevated blood lactate and receptor concentration in epithelial cells of brain microvasculature [14, 15], as well as increased synaptic function and blood flow. Collectively, elevated neurotrophin release and circuit function leads to spinogenesis, further dendritic complexity, and ultimately synaptogenesis. Sustained production of neurotrophins promotes neuro-, glio-, and angiogenesis [reviewed in 12]. Neurogenesis occurs in two specific niches of the adult brain, while gliogenesis is ubiquitous. Together, these cellular changes contribute to larger gray and white matter volumes (Fig. 1). The most recent advances in understanding how different plasticity-promoting behaviors are related to these mechanisms are reviewed below.
Fig. 1
Overview of the many neuroplastic mechanisms that are known contributors to neural remodeling. Created with BioRender.com.
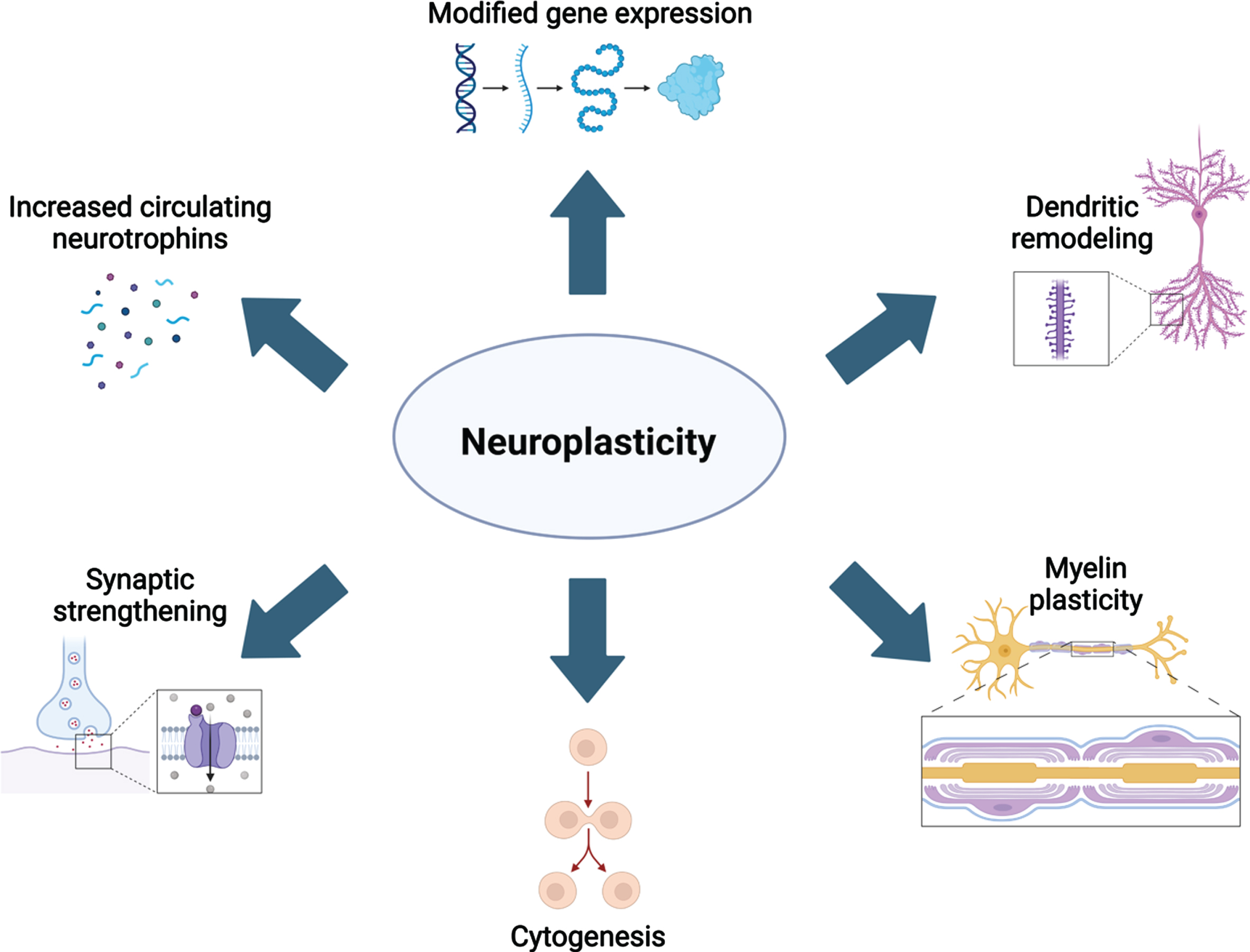
RATES OF NEUROPLASTICITY FROM ADOLESCENCE TO THE AGING BRAIN
Neuroplasticity occurs across the lifespan and is required for the maintenance and adaptation of neural connections. The developing brain is the most dynamic and the rate of brain plasticity is at its height during development; this is critical for connectome creation and optimization to support adult cognition. In support of this, neural connections remain lightly myelinated until late childhood when axonal pruning occurs. Following pruning, the remaining connections are heavily insulated during a second adolescent peak in myelination to stabilize connectome reorganization [16].
A series of experiments conducted in the second half of 20th century were pivotal in uncovering early periods of enhanced brain plasticity driven by experience-expectant and experience-dependent processes that are foundational in shaping brain structure and function [4, 17–19]. Hubel and Wiesel famously demonstrated that deprivation of visual input during an early experience-expectant critical period in childhood results in chronic visual impairment [17]. Indeed, mice exposed to an enriched environment from birth, housed with at least 6 mice and toys that are interchanged frequently for novelty have distinct retinotopic maps compared to mice reared in standard cages. The neuroanatomical alterations were strikingly different: mice exposed to the enriched environment had larger primary visual cortices, exhibited larger visual fields, and a unique organization of the cortical representation of the visual field coverage [20]. However, by the time that axonal pruning and secondary myelination occurs in late childhood and adolescence, the nature of neuroplasticity changes such that sensitive periods occur during which experience-dependent processes reversibly shape brain organization and function. Importantly, pathogenic onset prenatally, during infancy, or in adolescence has the potential to alter the developmental trajectory of the brain, ultimately changing the onset and length of critical and sensitive periods. We encourage the readers to study the reviews by Ho et al. [21] and Bandeira et al. [22] which describe adolescent mechanisms of neuroplasticity in greater detail.
Since 2017, several new discoveries have highlighted paradoxical changes to brain organization and behavior following specific environmental stimuli based on timing of exposure (adolescence vs. adulthood). Li and colleagues discovered that administration of low dose corticosterone to adolescent rats increased the expression of BDNF and AMPA receptor subunits in the hippocampus, and these changes were associated with enhanced inhibition and spatial learning capacities [23]. The opposite was found if rats were exposed to the same low level of corticosterone in adulthood; this exposure increased anxiety-related behaviors [23]. Similarly, it is well-established that social isolation, a plasticity-reducing stimulus, increases anxiety-like behaviors in adult rats [19]. Within the last five years, several groups have uncovered that isolation exposure in adolescence through adulthood led to reduced anxiety-like behaviors, suggesting a potential resiliency [24, 25].
In addition to these findings, a review of the neuroplasticity literature over the last five years emphasizes novel methods for supporting or stimulating neuroplastic remodeling in the aging brain to prevent cognitive and motor decline. It is well-established that the potential for neuroplastic alteration is limited in the aging brain due to reductions in glutamatergic and cholinergic signaling, production of neurotrophic factors [26], competing upregulation of cytokine production, epigenetic remodeling that downregulates cell mitosis and favors mature cell phenotypes [27], cellular senescence, in addition to disrupted bioenergetics [reviewed in [28]] and deterioration of the blood brain barrier that restricts nutrient supplementation to the brain. An individual’s lifestyle is one of the most influential factors contributing to the onset and rate of brain aging, ultimately mediating severity of neurodegeneration. Tarumi and colleagues showed that white matter integrity in the aged human brain was comparable to that in the young adult brain if participants reported exercising throughout their life [29]. Indeed, sustained cognitive training across life has also been suggested to stave off the onset of aging-related dementia [reviewed in 30]. Novel findings on the impact of key plasticity-evoking and reducing stimulations across the lifespan are reviewed in greater detail in the following sections.
TYPES OF PLASTICITY-EVOKING STIMULATIONS
Exercise
Exercise has long been established as a positive modulator of brain health; it supports neuroplastic alterations that allow the brain to adapt to changes in the environment, cognitive demand, age or in response to disease/trauma. Searching keywords [aerobic exercise AND neuroplasticity] in PubMed on 5-22-23 yielded 5,627 papers published between 1950-2023 and about one half of these articles have been published between 2017-2023, demonstrating an ever-increasing interest in this topic. The earliest empirical studies uncovered that voluntary aerobic exercise increases VEGF and lactate in peripheral muscles which in turn upregulates vasculogenesis, neurogenesis, the release of neurotrophic factors, cerebral blood flow and waste clearance in the healthy brain [31–37]. Importantly, voluntary wheel running stimulates neurotrophin production, serotonin release [38], reduces reward sensitivity of basal striatal dopaminergic neurons [39], and yields the most motor recovery following intracerebral stroke [40] compared to forced training on a treadmill. Forced training elevates anxiety and stress-related behaviors in trained rodents, due to the onset of an inflammatory cascade and limited serotonergic activity [38], and this is damaging to brain health. These findings have been replicated in animals and humans. Additionally, Lai and colleagues (2021) showed that aerobic activity attenuated increased neuroinflammation and decreased gut microbiota diversity in aged mice that received intracranial surgery, indicating that exercise may mediate post-surgical recovery in the aged brain via the gut-brain axis [41]. Within the last 5 years, researchers have focused on identifying exercise-induced changes to white matter development and maintenance of adult structures known as myelin plasticity (Fig. 2). A PubMed search including the keywords [aerobic exercise AND myelin] revealed 425 articles published between 1967-2023 discussing this topic; only 165 articles were published between 2017-2023. Overall, it is suggested that voluntary aerobic exercise may promote adaptive myelination in the adult brain via the production of oligodendrocyte precursor cells (OPCs) stimulated by increased circulation of VEGF and BDNF in the corpus callosum and prefrontal cortex [42–47]. However, Bloom et al. aptly point out that proliferation of precursor glia without a parallel increase in the number of myelinating oligodendrocytes, suggests that myelin remodeling is incomplete or not maintained in the exercised adult brain [44]. Importantly, Tomlinson et al. showed that aerobic activity stimulated OPC production and oligodendrocyte maturation in the prefrontal cortex of adult rodents, but not in juvenile rodents following increased voluntary aerobic activity likely due to Wnt-mediated proliferation and differentiation of OPCs in gray matter [45, 48]. In both age groups, OPC production and maturation was supported in the corpus callosum following aerobic activity, suggesting that myelin plasticity is age- and region-specific in the mammalian brain. Convergent with these preclinical findings, Routsalainen et al. have demonstrated that aerobic fitness in teenagers aged 12–16 years was positively correlated with maturation (higher Fractional Anisotropy and lower Radial Diffusivity) of the corpus callosum, suggesting that the thickness of the myelin sheath was increased in exercising youth [49]. Similar benefits of aerobic activity on white matter maturation have been identified in other white matter tracts and subregions of corpus callosum [50–52].
Fig. 2
Molecular and cellular alterations resulting from aerobic and resistance training in the prefrontal cortex (purple) and corpus callosum (blue) in the healthy, aging, and pathological brain with citations. Associated alterations to behavior are summarized. Created with BioRender.com.
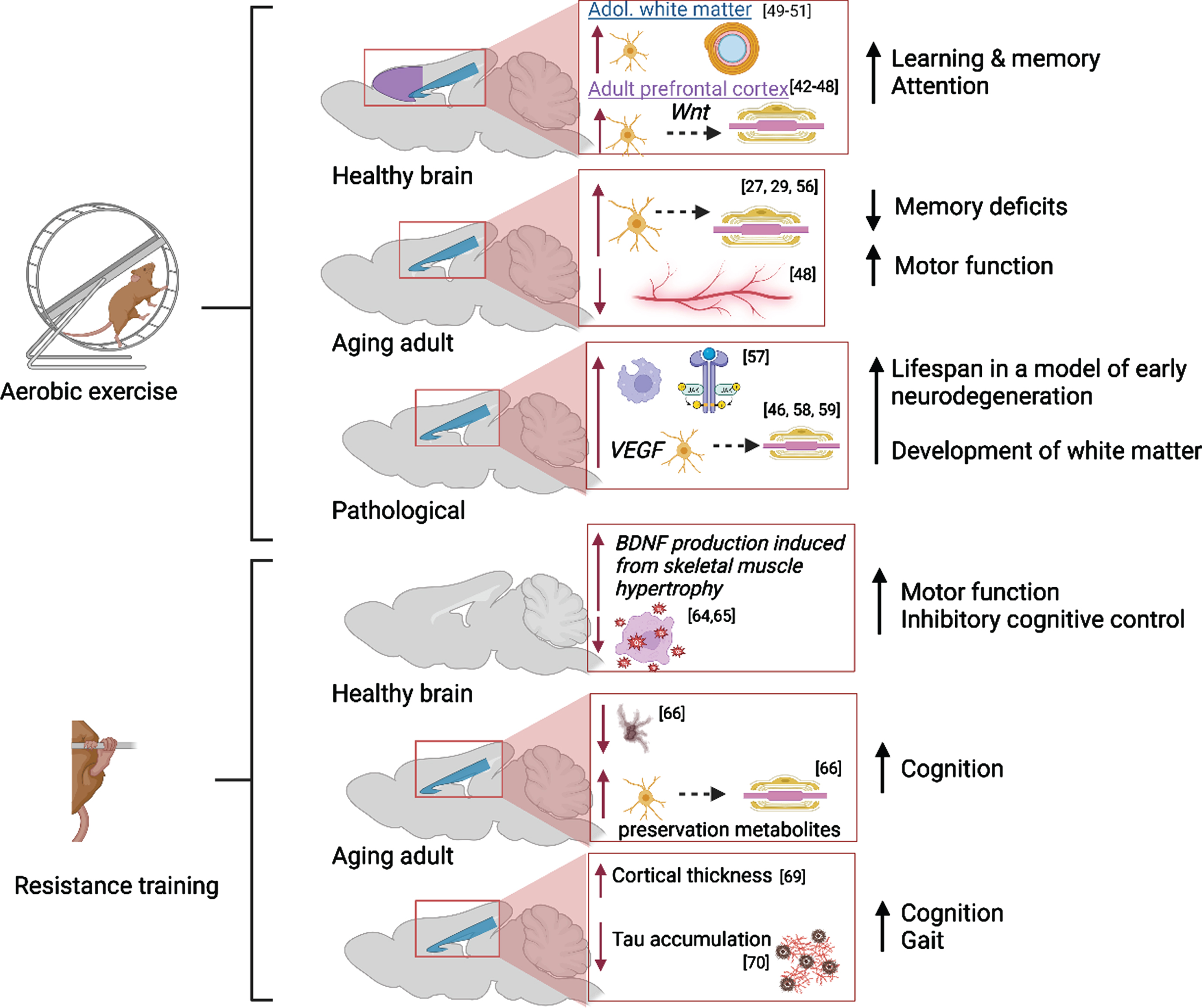
In addition, aerobic activity supports white matter integrity in the aged brain via myelin remodeling [44, 53]. Lower physical activity in adulthood is associated with higher neurodegeneration during aging due to slowed production of protective factors (i.e., decreased neurotrophin production leading to white matter degradation) and poor cardiovascular and neurovascular health [54]. Tarumi and colleagues reported that the integrity of global white matter in aging adults who regularly participated in aerobic exercise was comparable to that in young adults [29]. The impact of exercise intervention in aged adults is less clear: Clark and colleagues did not uncover any immediate benefits of a six-month aerobic exercise regimen on white matter integrity in adults aged 57 to 86 years [55]. However, there is evidence from preclinical studies that a longer intervention period (14 months) was successful in preventing age-related declines in white matter and intra-white matter vascular atrophy in middle-aged (18 months) and aged (28 months) rats [56]. Stimulation of precursor cell differentiation by exercise directly combats slowed oligoglia maturation in the aging brain [27].
Further, myelin remodeling in neuropathology has been associated with increased production of myelin basic protein, density of remyelinated axons and thickening of myelin sheaths in white matter regions [reviewed in 44]. A study using a mouse model of Multiple Sclerosis found that pro-growth mechanisms were triggered early in remyelination and followed by the upregulation of anti-inflammatory processes and phagocytic activity [57]. In a mouse model of progressive early neurodegeneration, exercise increased oligodendrogenesis via elevated VEGF production and ultimately lengthened the mouse lifespan from 25 days to 1 year [46]. In a rat model of Fetal Alcohol Spectrum Disorders, it has been discovered that increasing aerobic activity in adolescence restores the trajectory of late corpus callosum development via promotion of oligoglia maturation in the alcohol-exposed brain [58, 59].
There are distinct differences between the effects of aerobic, strength and combination training on neuroplasticity. Resistance activity aimed at increasing muscle strength, refines corticospinal tract connectivity and maximizes efferent activation of motor neurons. Aagaard et al. and Herold et al. comprehensively reviewed the major findings from several paramount preclinical and clinical studies measuring neuroplastic changes resulting from strength training [60, 61]. Notably, while aerobic activity supports the genesis of capillaries, neurons, glia, waste clearance, and myelin plasticity, strength training appears to enhance existing neuronal connections. The benefits of combined aerobic and strength training are reviewed in a following subsection.
The impact of strength training on neuroplastic alterations is similarly dependent on age and overall health of neural tissues. Searching keywords [strength or resistance training AND neuroplasticity] in PubMed on 7-18-23 yielded 143 papers published between 1990-2023 and 87 these articles have been published between 2017-2023, highlighting that the paucity in research on this topic is slowly being addressed. However, the effects of resistance training on mechanisms of neuroplasticity are controversial. Pinho et al. reviewed the divergent theories by which resistance training impacts cerebral BDNF production, neurogenesis, oxidative stress, and boosts cognitive inhibition [62]. There is very little known about the effects of strength training on adolescent neuroplasticity. In 2008, the Canadian Society for Exercise Physiology released a paper recommending modified resistance training paradigms for children [63]. In addition to the direct effect of resistance training on neural remodeling, Palmer et al. and Hendy et al. review seminal evidence that distinct neural mechanisms are responsible for the cross-education phenomenon: increasing the torque of maximum voluntary isometric contraction in contralateral untrained limbs following resistance training in one limb [64, 65]. More work is needed to further delineate how these mechanisms contribute to overall brain health in the developing and adult brain; yet it is likely that resistance training upregulates peripheral and cerebral BDNF production and acts as a cerebral antioxidant, creating a favorable environment for neuroplastic remodeling. In comparison to the effects of aerobic activity on white matter integrity in the aging brain, strength training also supports white matter integrity in the aging brain. In the aged brain, progressive neurodegeneration categorized by white matter lesioning was delayed in older women (aged 65-75) who engaged in resistance training twice weekly for 1 year [66]. However, in a meta-analysis assessing the behavioral outcome of strength training, the researchers discovered that increasing the intensity of aerobic or resistance training was positively correlated with improved motor function in young adults. However, a similar correlation was less clear in aged adults [67]. Interestingly, in another study moderate and high intensity resistance training for twenty-four weeks was sufficient to improve cognitive function in elderly adults [68].
In pathological conditions, resistance training has been shown to mitigate disease-related lesioning of white matter. Twenty-four weeks of progressive resistance training increased cortical thickness in adults with Multiple Sclerosis, a disorder characterized by white matter degeneration [69]. Convergent with these findings, Amiri et al. discovered that the accumulation of Tau protein (a sign of axonal deterioration) in women with Multiple Sclerosis was significantly reduced in the brain following a 3x/week resistance training session that lasted a total of 8 weeks [70]. However, the meta-analysis conducted by Hortobagyi et al. emphasizes an increasing need for more research concerning this topic as this group did not find any correlation between the intensity of resistance training and motor function in neurological patients with Multiple Sclerosis, Parkinson’s disease, and stroke [67]. While few studies have investigated the impact of resistance training in the developing brain, strength training is a common intervention for children and adolescents with cerebral palsy. Mockford et al. provide a comprehensive review of the improvement of gait in children and adolescents with cerebral palsy [71] which may be attributed to strengthening of neural circuits involved in motor coordination.
Lastly, cessation of aerobic activity in physically fit individuals can negatively affect brain health. Alfini et al. have shown that master athletes that cease aerobic activity for 10 days have marked reductions to cerebral blood flow in the hippocampus, a prominent neural structure for learning and memory [54]. Moreover, Nishijima and colleagues discovered that exercise cessation in rats with voluntary access to running wheels transiently reduced neurogenesis below control levels for a few months, in a negative rebound nature [72]. To date, there are no studies that have investigated the impact of exercise cessation on myelin plasticity.
Complex motor learning and acrobat training
Complex motor learning via the manipulation of forelimbs or distal digits and acrobat training require significant structural and functional changes in the circuitry of the primary motor cortex (M1) and cerebellar cortex, respectively [reviewed in 12, 73–76]. The primary method involved in acrobat training includes teaching rodents how to navigate a challenging circuit with many obstacles that requires physical and cognitive exertion including ropes, ladders, grids, ramps, and seesaws [77–79]. These changes require altered gene expression and protein synthesis to facilitate changes in neuronal dendrites and synaptic connectivity. In addition to basic motor learning and acrobat training, many other movement-based behaviors lead to experience-dependent plasticity changes including (but not limited to) locomotion, vocalization or any other behavior where learning of a new controlled motor pattern is required.
M1 is considered a central brain region for initiating and encoding skilled voluntary movements. Studies published before 2017 showed enlargement of cortical map representation of trained digits and forearm after motor learning [80, 81] which were paralleled by cortical synaptogenesis in Layer V of these regions [82] and increased basilar dendrite length in Layer V pyramidal neurons [83]. Recent developments in rodent in vivo imaging have allowed for the combination of longitudinal structural MRI with immunohistochemistry following motor skill learning (skilled, single-pellet forelimb reach task) and demonstrate that learning resulted in bilateral nonlinear decreases in gray matter volume juxtaposed with nonlinear increases in white matter volume in primary and secondary motor areas and in somatosensory cortex [84] due to an increase in myelin content and the number of newly differentiated oligodendrocytes [85] in the somatosensory cortex of the trained forelimb. These findings are summarized in Fig. 3.
Fig. 3
Molecular and cellular alterations resulting from motor learning in the motor and somatosensory cortices (purple) with citations. Associated alterations to behavior are summarized. Created with BioRender.com.
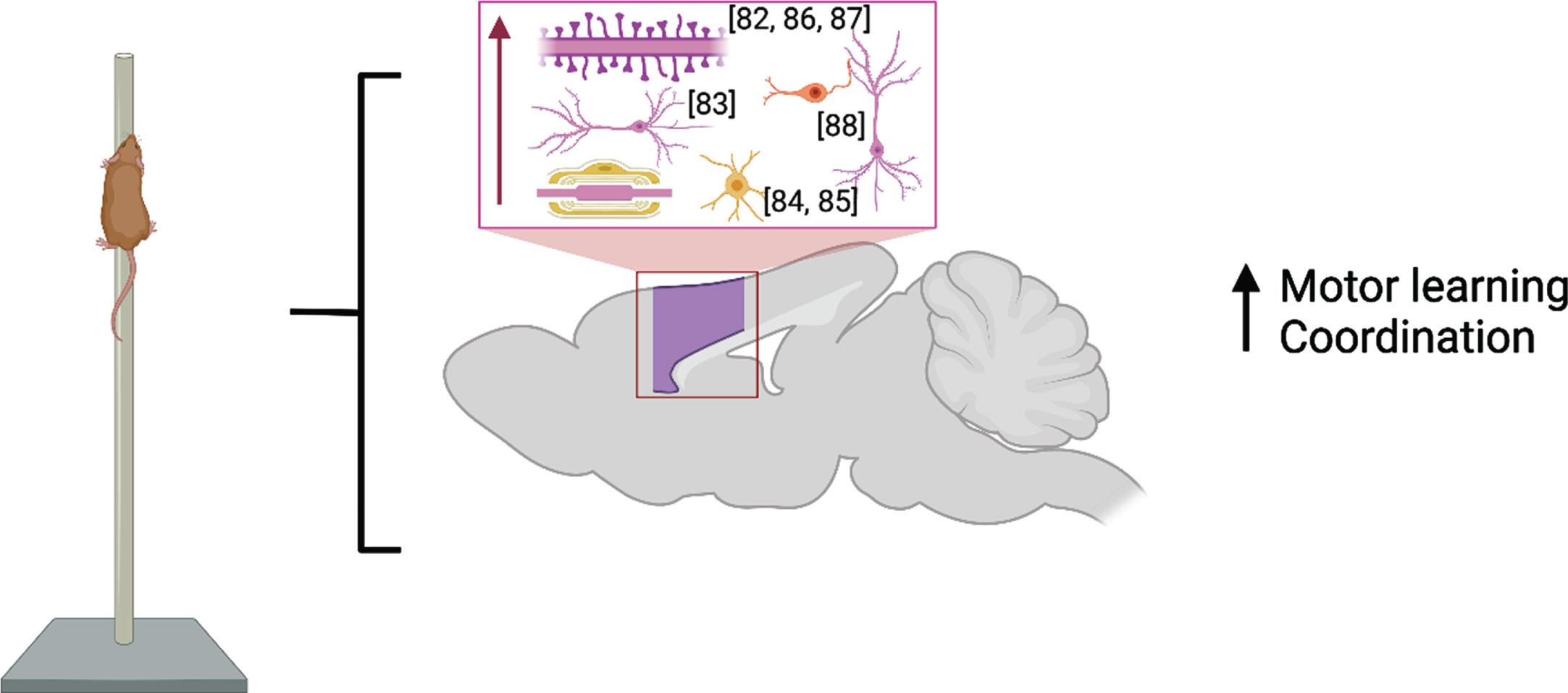
It has been demonstrated many times that skilled motor learning enhances brain plasticity in the form of increased dendritic length and complexity, and in synaptogenesis en masse. The genetic targeted recombination in active populations (TRAP) approach used in Hwang et al. was used to label M1 neurons specifically involved in circuitry underlying learning a forelimb reaching task in rodents [86]. In vivo imaging revealed that this population of behavior-related neurons in M1 represented an engram that was reactivated during the recall of the learned task. Motor learning induced a transient increase in dendritic spine density and stabilization of newly formed spines specifically on engram neurons but not on neighboring non-TRAPed neurons.
While many studies evaluate changes in plasticity during the process of learning (longitudinal studies) or immediately after completion of a learning paradigm, few have addressed the time-course of learning-induced changes in brain plasticity. Streffling et al. assessed progressive motor-learning-induced dendritic plasticity and spine density in M1 by studying dendritic morphology of layer II/III pyramidal neurons at day 0, day 30 and day 60 following acquisition of a skilled reaching task in rats [87]. As Layer II/III neurons are known to receive and process cortical and thalamic input, their involvement in circuitry underlying skill-dependent learning is indisputable. There was a transient increment in complexity of the distal apical dendrite 30 days after training, while length and complexity continuously decreased in basal dendrites of superficial and deep layer II/III pyramidal cells. Spine density increased over time in apical and basal dendrites of both superficial and deep layer II/III neurons accompanied with a morphological change towards stubby- and mushroom-like spines, indicating increased maturity. Thus, profound but delayed changes occurred within the dendritic compartment of layer II/III pyramidal cells following motor training, highlighting the lasting benefits of motor learning on cortical-thalamic circuit connectivity.
Synaptic plasticity in cortical circuitry required for motor skill learning occurs both in excitatory and inhibitory components of the modified circuits. Investigation of learning-related spine plasticity in dendritic compartments of neurons in the upper layers of M1 demonstrated that plasticity occurred in layer I distal dendritic branches but not in perisomatic regions of layer II/III excitatory neurons [88]. Studying the role of two genetically identified inhibitory interneuron subtypes, Chen and colleagues revealed distinct subtype-specific plasticity of inhibitory circuits, in which distally-targeting somatostatin interneurons decreased their synapses in layer I and perisomatically-targeting parvalbumin neurons increased their synapses [88]. These results shed light on a mechanism by which subtype-specific inhibitory circuit plasticity regulates specificity of learning-related structural plasticity of excitatory synapses involved in acquisition of motor skills.
Complex motor skill “acrobat” training is a powerful plasticity-promoting approach known to evoke significant plastic changes in various but specific brain areas (e.g., motor and cerebellar cortex but not deep cerebellar nuclei) and to result in improved motor task performance, increase in synaptogenesis and expression of neurotrophins and synaptophysin [80, 89, 90]. Plasticity mechanisms that facilitate retention of such skills were addressed in Sampaio et al. where it was demonstrated that acrobat training promoted significant plastic changes in the expression of proteins in motor and cerebellar cortices [91]. Unfortunately, the studies identifying known mechanisms of acrobat-related plasticity are scarce, especially recently.
Environmental Complexity/Enrichment
Environmental complexity (EC) or enrichment (EE) is a fundamental plasticity-driving intervention that has been integral for elucidating the interactions between experience-dependent mechanisms in the brain. EC or EE broadly consists of three major components: physical activity, cognitive stimulation, and social interaction. Traditionally, EE consists of housing at least 6 same-sex rodents in one cage and providing toys, ramps, and, in some cases, running wheels that are exchanged for novelty every few days [92]. One of the primary examples by which EC/EE changes the brain is by adult neurogenesis and synaptogenesis in the hippocampus. Unlike in exercise paradigms, EC/EE-stimulates synaptogenesis which is imperative for the integration of newly proliferated cells into existing neural networks. Network remodeling is required for sustaining brain health long-term. A second distinguishing factor between exercise and EE paradigms is that the increased challenge presented during EE results in greater reductions to anxiety-like behaviors [93]. Third, EC/EE-related neurogenesis is supported by heightened activity of the fibroblast growth factor receptor [94], elevated expression of chondroitin sulfate proteoglycan, a regulator of embryonic neurogenesis [95], and finally through CD8 + T cell-regulated neurotrophic factor expression [96] instead of via upregulated VEGF and BDNF production as previously described. Finally, there is emerging evidence to suggest that EE/EC plays a greater role in preventing cognitive decline in aging rodents compared to increased physical activity [97]. A recent study by Ramirez-Rodriguez et al (2022) explored the anxiolytic and neurogenic effects of EC variations (continuous EC, increasing complexity of EC, decreasing complexity) versus exercise. They demonstrated decreased anxiety in mice exposed to EC variations, but not after continuous EC or after exercise in the running wheels. The number of immature DCX+ neurons was increased at a higher level by all EC conditions than by running, which in turn produced more immature neurons than in control brains. Since its beginning, much research has been conducted that has identified numerous plasticity-related mechanisms such as modified gene and protein expression [reviewed in 98].
Despite this model’s extensive utilization and study throughout the past 80 years, EC/EE remains a topic of interest in the field of brain plasticity as well as intervention research. Using the keywords [((complexity) OR (enrichment)) AND (environment) AND (plasticity) AND (brain)] yielded 1,655 papers published between 1975–2023 (per PubMed on 05/06/2023). Narrowing the search to papers published from 2017–2023 produced 603 results, while further narrowing by excluding reviews produced 473, which demonstrates that this plasticity-inducing paradigm is still of great interest to the scientific and medical communities. The focus of many of the empirical papers published in the last five years is to elucidate brain mechanisms responsible for experience-dependent plasticity that occurs in the rodent brain when exposed to a complex environment.
EC/EE not only promotes neuro- and synaptogenesis, but modulates activity of inhibitory and excitatory hippocampal neurons, facilitates myelin remodeling, and alters lipid metabolism. Loisy et al. showed that 3 weeks of EC/EE housing limited the plasticity of inhibitory activity from CA3 to CA2 hippocampal subregions in adult mice [99]. The authors used a variety of electrophysiological techniques to demonstrate that GABA transmission was reduced overall in CA2 and that this effect could be reversed if mice were socially isolated. In another seminal study, Dahlmanns and colleagues showed that increasing the duration of EE (4 hours vs. 12 hours) effectively enhanced activin A production, CA1 pyramidal neuron excitability and increased sensitivity of the hippocampal theta generator to cholinergic stimulation optimizing performance of hippocampal circuits in response to higher demand (behavioral stimulation) [100]. Together, these studies demonstrate how excitation of hippocampal CA1 is induced following EE. Additionally, Fletcher et al. demonstrated that 6 weeks of EE led to significant myelin remodeling: a global increase in axonal diameter, myelin sheath thickness, and paranode length in adult mice. The authors revealed an increase in the differentiation of premature oligoglia in corpus callosum and somatosensory cortex, which suggests that EE is a powerful modulator of myelin-dependent plasticity in young adulthood [101]. Alterations to myelin ensheathment fine tune synaptic communication between brain regions. At the molecular level, Borgmeyer et al. identified several lipids and proteins that are upregulated in the mouse and rat brain following 6 weeks of EE [102]. This multiomics study was the first to create a lipidomics library of EE-induced changes in the rodent brain. Most notably, the authors uncovered that EE reduced endocannabinoid signaling in dendritic spines, and this upregulated the expression of AMPA receptors, ultimately tuning the function of synaptic junctions [102]. These recent discoveries in the cellular and molecular mechanisms by which EE alters neurotransmission have significantly contributed to our understanding of how the environment shapes brain function.
While the unique effects of physical activity and cognitive stimulation have been extensively studied [103–105], studies that compare the contributions of the components of EC or EE in addition to social interaction have been lacking until recent years (Fig. 4). In 2019, Moreno-Jimenez and colleagues investigated the pro-neurogenic effects of prolonged social interaction compared to regular EC and control housing on both behavioral and neuroanatomical measures in rodents [106]. Adult mice were pair or triplicate-housed in standard home cages (control condition), in EE which consisted of a large cage housing 12 same-sex mice with running wheels and toys that were replaced every other day for novelty, or in social enrichment cages which included 12 mice without the added wheels and toys. Overall, the authors discovered that mice exposed to social interaction only displayed similar increases in DCX+ cells in the hippocampus as those exposed to the full EC paradigm. These morphological changes were associated with comparable increases in exploratory and pro-social behaviors. This suggests that social interaction alone may sufficiently stimulate neurogenesis. However, it is likely that comprehensive EC exposure may induce more robust neurogenesis or be accompanied by additional morphometric changes not assessed in the Moreno-Jimenez study. In a similar manner, four weeks of full EE or exercise alone comparably restricted corticosterone and ΔFosB expression in the basolateral amygdala in adult female prairie voles after isolation stress [107]. This could suggest that exercise may be the primary change-inducing stimulus for these physiological measures. However, it should be noted that this study lacked an intervention group that did not undergo isolation stress, which has been shown to induce pronounced and lasting changes to the brain (reviewed below). Additionally, a recent study by Brenes at al. compared EE or exercise alone with fluoxetine treatment after social isolation in rats [108]. EE produced the widest and largest anti-depressive effect, followed by exercise, then fluoxetine administration. Interestingly, while fluoxetine treatment resulted in the most robust increase in serotonin concentration, EE outperformed fluoxetine in normalizing 5-HIAA and 5-HT turnover, which suggests that the process by which serotonergic modifiers exert anti-depressive effects may be more complex and are mediated by unique experience-dependent mechanisms [108]. Finally, Mansk and colleagues (2023) recently illustrated that adult neurogenesis and new neuron survival was increased by housing in EE in both inbred Swiss and outbred C67BL/6 mice strain; however, the authors demonstrated that advanced social recognition memory following EE was maintained for longer in C57BL/6 mice compared to Swiss mice. This effect was abolished in C57BL/6 mice if they were isolate housed for an extended period [109].
Fig. 4
Molecular and cellular alterations resulting from social interaction and environmental complexity in the hippocampus (green) and prefrontal cortex (purple) with citations. Associated alterations to behavior are summarized. Created with BioRender.com.
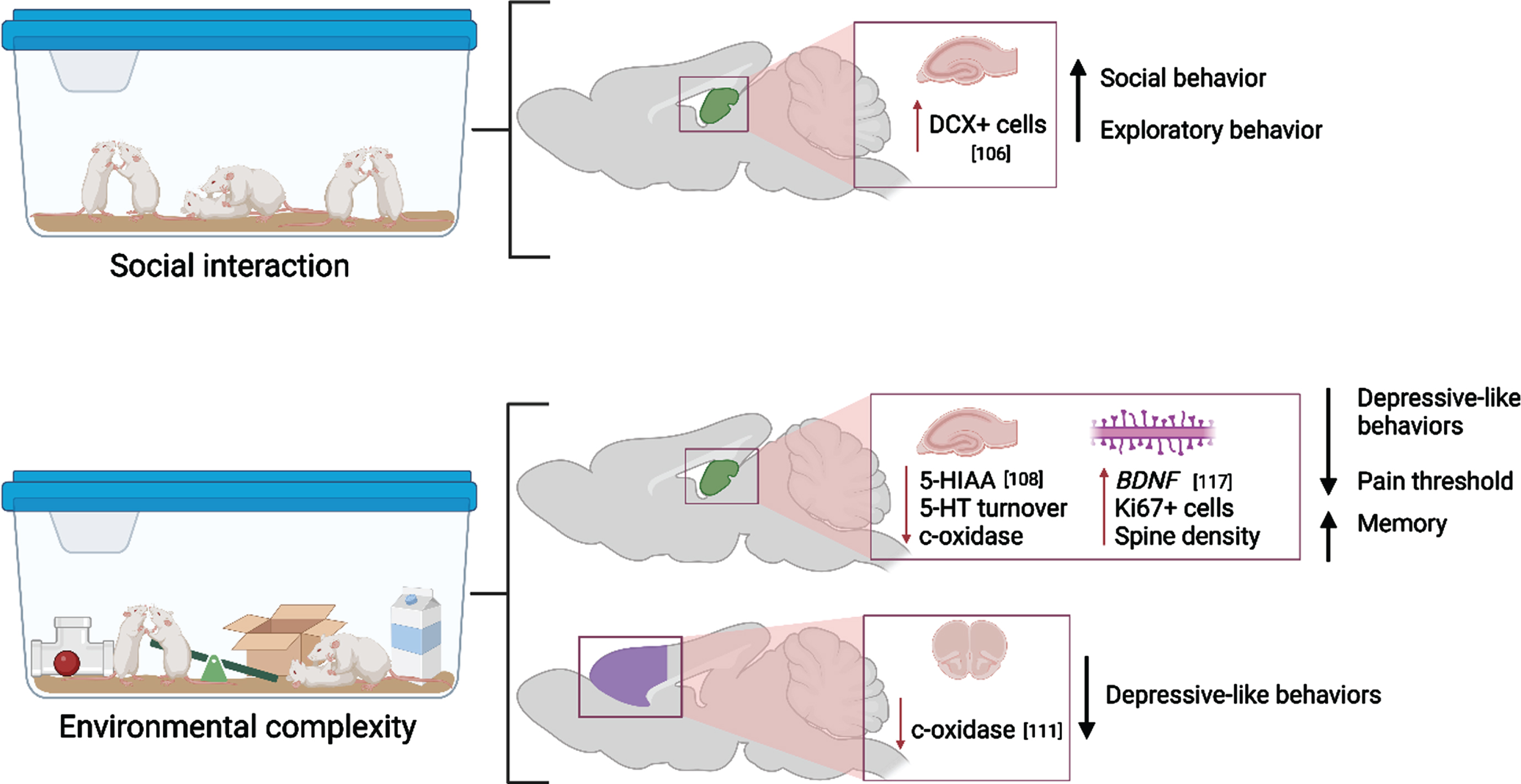
The full paradigm of EC/EE has been shown to have long-lasting effects on both memory and memory-related neuroanatomical substrates. Adult mice that underwent 40 days of EC exhibited extended recovery of object-recognition memory 20 days after learning occurred [110]. Similar effects have been seen to last long after EE ceases, as shown by Gonzalez-Pardo et al. by exposing rats to either EE or non-enriched environment for 45 days followed by 44 days of standard housing [111]. EE reduced anxiety- and depression-like behaviors as well as reduced metabolic capacity as measured by cytochrome oxidase histochemistry despite EE cessation, which may indicate better metabolic efficiency while performing behavioral tasks long-term [111].
Due to the many known effects of EC/EE on brain plasticity, much recent work has been committed to utilizing it as an intervention in preclinical models of disease. Across several studies utilizing rodent models of neurodevelopmental disease, researchers have demonstrated that EE is important for the development of spatial working memory. For instance, perinatal hypoxia has been shown to disrupt oligodendrocyte maturation and myelination and this is rescued by continuous rearing in EE between PD15-45 [112]. Likewise, EE during and immediately following pregnancy resulted in better performance on neurodevelopmental and spatial memory tasks of rat pups that underwent hypoxic ischemia on PD 3 [113]. Hypoxic pups that underwent EE during perinatal development also exhibited a lack of hippocampal tissue loss and increased GFAP expression otherwise seen in their non-EE counterparts. When mice were exposed to EE for five weeks immediately preceding ischemic injury, EE resulted in reduced infarct volume as well as prevented increased expression of inflammatory cytokine IL1-β [114]. One potential mechanism by which EE protects or repairs the brain is through the upregulation of neurotrophins such as BDNF. In another “two-hit” model of perinatal neuroimmune activation, two weeks of EE in late adolescence was shown to increase BDNF expression, TrkB expression, upregulate the expression of several excitatory-related receptors (NMDA, GluNR1, 2A and 2B) in “two-hit” mice compared to controls. Moreover, this was correlated with improved spatial working memory performance [115]. Additionally, Zajac et al. found that two weeks of voluntary exercise and EE in late adolescence had unique effects on serotonergic receptor expression in a transgenic mouse model of Huntington’s Disease. EE increased Htr2a gene expression in male mice, and this was associated with improvement on the head-twitch test. Findings from this study highlighted the impact of a short intervention period early in life to rectify behavioral impairments associated with Huntington’s Disease [116]. Early intervention with EE significantly enhances neurodevelopment and spatial working memory capacity in multiple models of neurodevelopmental disorders.
The potential for EC/EE to rectify maladaptive behaviors in adult rodent models of neuropsychiatric disease continues to be explored. Four weeks of EE in adulthood following chronic constriction injury of the sciatic nerve ameliorated reductions to pain threshold and BDNF protein expression, long-term memory deficits, LTP impairment, and synaptic plasticity impairments measured by hippocampal spine density [117]. However, the trkB antagonist ANA-12 blocked the effects of EE on long-term memory and synaptic plasticity, but not BDNF expression [117]. In another study, eight days of EC was found to increase DCX-expressing cells in the hippocampi of 11-week-old mice but this was not correlated with an increase in BDNF [118]. Taken together, BDNF signaling may have downstream effects on plasticity without modifying BDNF expression itself. In another study, researchers uncovered that EE enhanced the effect of antidepressants on regulating the hypothalamic-pituitary-adrenal axis in a corticosterone-induced mouse model of depression. Mice treated with the antidepressant venlafaxine during EE showed signs of recovery 4 weeks before mice treated in standard housing, and this was reflected in the brain as combined pharmaceutical-EE intervention reduced the number of hippocampal perineuronal nets and this was not identified in brain tissue extracted from treatment-only mice [119]. EE exposure at any age is beneficial for rectifying certain neuroanatomical and behavioral impairments resulting from various brain disorders and conditions.
Combined interventions
The synergistic impact of combined behavioral interventions on the stimulation of neuroplastic changes is noteworthy. In addition to the direct effects of single interventions, several groups have demonstrated that intervention combinations may be key for maintaining the neuroplastic changes described. As mentioned above, some single plasticity-evoking interventions contain multiple yet necessary components (e.g., environmental complexity being the additive effects of physical activity, cognitive stimulation, and social interaction). However, many distinct interventions have historically been used simultaneously or consecutively and show that neuroplastic alterations are sustained via implementation of these more complex intervention strategies. In several historic studies, preceding EC exposure with voluntary aerobic activity (“super-intervention”) not only stimulated neurogenesis, but helped to integrate newly-differentiated neurons into existing circuits in the typically-developing and diseased rat brain [120–122]. For further review of combined intervention success compared to individual stimulation paradigms, we encourage you to read [123]. In the last five years, combined intervention studies have aimed to identify novel therapeutic strategies and mechanisms associated with ameliorating behaviors in several disease states. Norouzi-Gheidari and colleagues showed that combining robotics, virtual reality activities, and neuromuscular electrical stimulation showed the most promise in ameliorating deficits in upper extremity movement resulting from chronic stroke [124].
More research is needed to evaluate the impact of combined intervention on neural remodeling in the aged brain. One such study investigated the impact of a cognitive-exercise dual-task intervention on cognitive decline in aged rats. Li et al. exposed 18-month-old rats to a cognitive operant conditioning task, passive exercise, or both five times a week for 12 weeks [125]. Rats in the dual-task group demonstrated improved performance on a novel object recognition test, as well as increased plasticity markers such as synaptophysin, BDNF, and glutathione peroxidase compared to rats in just one of the intervention conditions. Marinus et al. discovered that the concentration of peripheral BDNF of aging adults is immediately upregulated after strength and combination aerobic-strength training but not after aerobic exercise alone [26]. Indeed, levels of circulating brain-derived neurotrophic factor reflect levels of this factor in brain tissue across species [126]. It is evident that combined interventions are best for stimulating neuroplasticity in the aged brain.
Increasing intervention “intensity” accelerates neuroplasticity. Functional alterations to neural networks are particularly sensitive to varying levels of intervention exposure. Andrews et al. showed that cortical network function was enhanced in an intensity-dependent manner in young adults exposed to interval training [127]. BDNF polymorphism was fundamental in mediating this relationship; specific epigenomic alterations were associated with the varying degrees of exercise intensity. Similarly, tiered intervention approaches have proven to be beneficial in mitigating neural remodeling in disease states. Zhan and colleagues demonstrated that a three-phase enriched environment intervention supported post-stroke recovery in adult rats by increasing the levels of socialization, environmental novelty, and potential for voluntary physical activity over 1-month during the stroke recovery period [128]. Noninvasive magnetic resonance imaging revealed that striatal and cortical volumes were preserved in ischemic rats following intervention exposure. Moreover, cerebral blood flow in these regions and angiogenesis in peri-infarct areas was improved in intervention-exposed ischemic rats compared to ischemic rats without intervention. Improved structural remodeling was positively correlated with improved spatial learning capacity.
PLASTICITY-REDUCING FACTORS
Isolation/Individual housing
Environmental complexity is widely and incontestably accepted as a powerful brain/neuronal plasticity-enhancing environment. The counts of empirical papers demonstrating this phenomenon using the keywords [enriched AND environment AND brain] exceed 4,800 (per PubMed on 04/18/2023): narrowing the search to [enriched AND environment AND brain AND plasticity] resulted in 886 papers published between 1978 and 2023, with 385 papers in 2017–2023. The effect of social isolation is significantly less studied: search for [social AND isolation AND environment AND brain AND plasticity] produced only 124 results in the period between 1978 and 2023, with 53 papers published in 2017-2023. These numbers clearly indicate that our knowledge of the plasticity-reducing effect of isolation is far from complete. The importance of understanding the immediate and long-lasting effects of social isolation on mental and psychosomatic health and their mechanisms has been emphasized by a recent global event: the COVID-19 pandemic. Here we summarize the major findings from studies examining the effects of environmental and social deprivation (Fig. 5).
Fig. 5
Molecular and cellular alterations resulting from isolation during different life stages in the prefrontal cortex (purple) and hippocampus (green) with citations. Associated alterations to behavior are summarized. Created with BioRender.com.
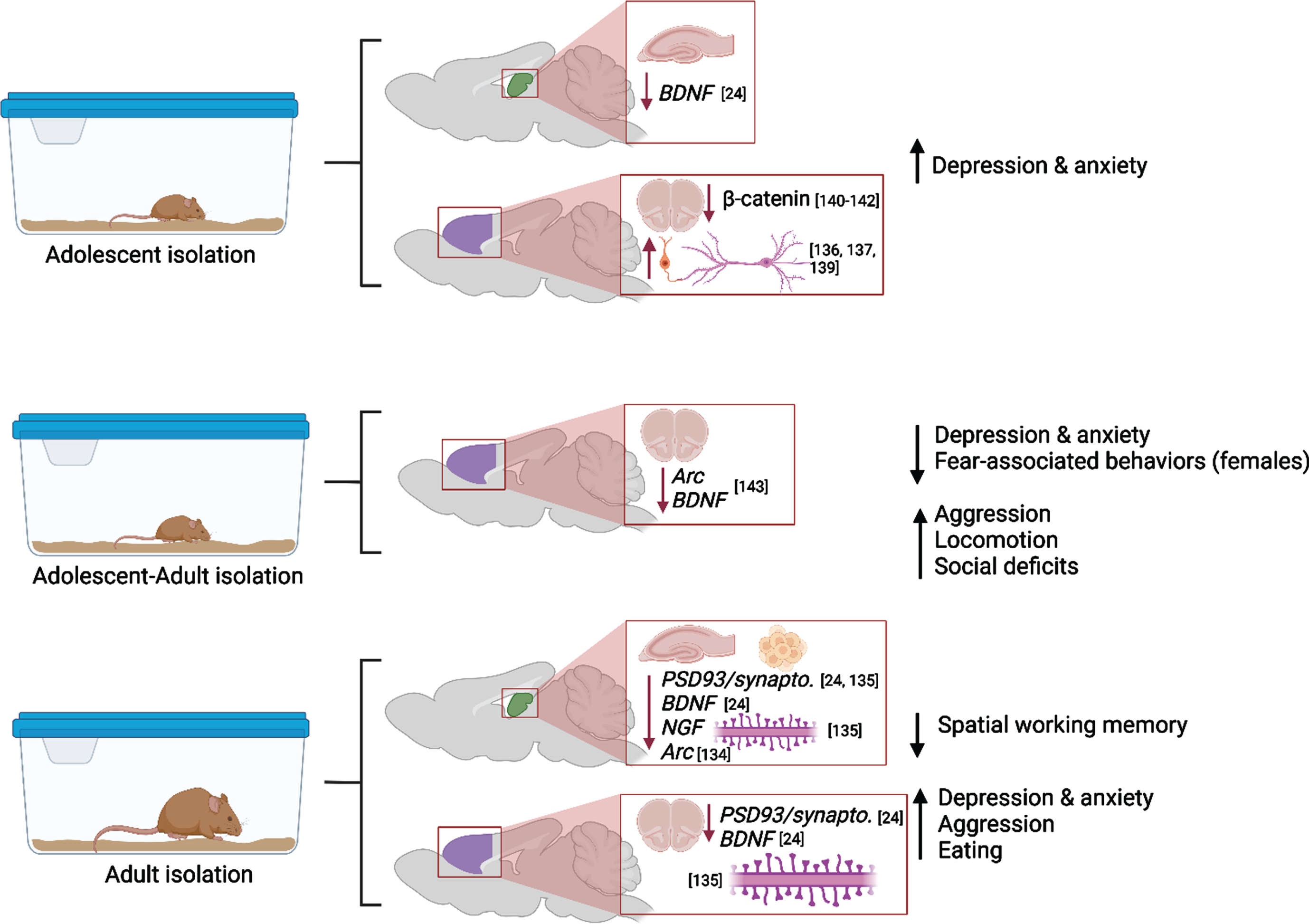
Social isolation (SI) induces lasting negative effects on the brain, and these effects could be more powerful or distinctly different when SI occurs during vulnerable periods, such as adolescence. Social isolation could result in lasting behavioral abnormalities and neuropsychiatric disorders, including aggression, substance addiction, obsessive-compulsive disorder, and eating disorders [129–131]. Adolescents, especially girls, are more likely to suffer from anxiety and depression during and after SI [132]. Preclinical studies have demonstrated that in post-weaning rats the impact of continuous SI manifested in depressive-like behaviors, however SI extended from postweaning into adulthood paradoxically decreased anxiety [24]. Fear-related behaviors were decreased in postweaning and adult SI animals. Decreased anxiety-related behavior was confirmed in adult female (but not male) rats after SI in adolescence and testing in adulthood [25]. Moreover, rats reared in SI from weaning until early adulthood showed abnormal forms of aggression and social deficits that were temporarily ameliorated by re-socialization, but aggression was again escalated in a novel environment [133]. SI resulted in hyperactivity in rats exposed to isolation on PDs 21-101 [134], and these results have been mirrored in clinical studies which have proven that early-life adversity (i.e., neglect) has been associated with hyperlocomotion in youth and adults.
These behavioral alterations were paralleled by decreased expression of notable plasticity-related proteins in hippocampus and prefrontal cortex (BDNF in adolescent and adult hippocampus, pERK and pCREB in adult hippocampus, PSD-95 in adult prefrontal cortex) and by increased expression of these factors in adult amygdala [24]. Four weeks of SI in adulthood caused cognitive dysfunction (novel object recognition test, Morris water maze), decreased synaptic proteins (synaptophysin or PSD93) and BDNF expression and dendritic spine numbers in the prefrontal cortex (PFC), dorsal hippocampus, and ventral hippocampus [135]. Begni et al. reported a strong reduction in the expression of Arc and BDNF mRNA in PFC after SI on PD21-101 [134].
In the PFC, SI after weaning reduced the number of excitatory synaptic inputs, and enhanced the quantity of inhibitory synaptic inputs on pyramidal neurons that densely innervate the dorsomedial striatum (DMS), compromising the intrinsic excitability of these neurons and shifting the PFC to a less excited state overall [136, 137]. A study by Shan et al. revealed that adolescent SI suppresses excitatory synaptic neurotransmission, both presynaptically and postsynaptically, specifically onto dorsal medial septal D1 receptor medium spiny neurons, but not onto other neuronal types (e.g., dorsal medial septal D2 receptor medium spiny neurons) [138]. As the dorsal striatum plays a central role in encoding goal-directed action and habitual response, the suppression effect in the excitatory neurotransmission onto the dorsal medial striatal D1 receptor medium spiny neurons is responsible for the SI-induced shift in the balance of decision-making strategy from goal-directed action to habitual behavioral approach [138].
It should be noted that effects of adolescent SI could be more severe if such exposure occurs as a second “hit” after early adversity (e.g., after early maternal separation or neglect). Gildawie et al. demonstrated sex-specific impact of early life adversity combined with SI in adolescence on adult locomotion and risk-assessment behavior, where females are more sensitive to adversity-induced deviations in performance [25]. Parvalbumin-positive interneurons’ counts and perineuronal nets integrity in medial PFC were also affected in sex-specific manner, suggesting that females could be at higher risk of long-term neural and extracellular consequences following multiple hits of adversity. These findings are in line with recent reports that development of PV interneurons in PFC is affected by SI during a juvenile critical window, resulting in reduced number and activation of these interneurons, and subsequent social behavior deficits [137, 139].
The powerful detrimental effect of SI in adolescence is further confirmed by the study of Cuesta et al. where five days of isolation during adolescence led to molecular changes in the Wnt/ß- catenin pathway in PFC and to affect anxiety-related behaviors during adulthood [140]. In neurons, ß -catenin regulates synapse formation and function as well as axon growth and branching [141, 142] thus stress-induced reduction of ß -catenin during adolescence could affect the protracted maturation of the PFC circuitry and lead to long-term changes in PFC function.
Effects of SI in adulthood parallel the effects of isolation in adolescence. Biggio et al. highlight molecular and structural impairments in the hippocampus of socially isolated rats: the reduction in the amount of BDNF, NGF and Arc elicited by SI were associated with a significant reduction in dentate gyrus neurogenesis, spine density and dendritic arborization [143]. Popa et al. discovered that social isolation of young adult and aged mice reduced the sociability of the mice, and this was related to the expression of specific micro-RNAs in the prefrontal cortex [144]. Moreover, Sukegawa and colleagues found that the removal of rodents from EE/EC elevated aggressive behaviors and disrupted sleeping patterns. The maladaptive behaviors were observed up to 2 weeks following enrichment removal [145].
A comprehensive review of the effects of SI on human and animal behavior and brain, covering two decades of research in detail, was published in 2022 [146].
DISCUSSION AND CLINICAL RELEVANCE
The medical and scientific communities continue to study the mechanisms of brain plasticity to develop interventions, predict outcomes of adverse experiences, and to prevent or ameliorate neuropathology. As summarized in the preceding sections, recent advances have uncovered that the timing and length of plasticity-evoking or -depriving stimuli are paramount features that contribute to neuroplastic alteration and modified behaviors. In summary, the studies published in the recent years confirm that the brain is most sensitive to neuroplastic alteration during development and into young adulthood. However, events occurring prenatally or in early life may alter the capacity for neuroplastic alteration later in life. Moreover, recent advances have uncovered that neuroplastic remodeling is possible in the aging and diseased brain (i.e., hypoxia, Multiple Sclerosis, Parkinson’s Disease, stroke, and Fetal Alcohol Spectrum Disorders).
The clinical relevance of combining multiple interventions can be exemplified by several studies conducted in recent years. Fraser et al. utilized an intervention consisting of 12 weeks of aerobic exercise coupled with either cognitive training or computer training to demonstrate that combined intervention resulted in benefits to balance and gait in older adults compared to just a single intervention alone [147]. Similarly, there has been much recent work dedicated to utilizing motor-based interventions to enhance the structural neuroplasticity of patients with various brain injuries such as stroke [reviewed in 148]. Even EC, which may be confounded by socioeconomic status, has been implicated in neuroplastic changes to functional brain network development when applied to adults [149]. Importantly, EE has been shown to improve cognitive outcomes in children irrespective of socioeconomic status, which parallels findings in rodent studies [150, 151].
The ability of the brain to reorganize its structure and function via neuroplastic mechanisms makes these stimuli invaluable tools for ameliorating deficits that may not respond to other treatment, such as stroke or spinal cord injury, Alzheimer’s disease, or treatment-resistant neuropsychiatric disorders [reviewed in 152]. There is also great potential for plasticity-evoking behaviors to be used synergistically with cognitive behavioral therapy as a more robust treatment for depression [reviewed in 153].
Taken together, the findings summarized above demonstrate that there is still much to be elucidated regarding the effects of neuroplasticity-evoking behaviors on the brain, despite the abundance of past research. Each stimulus provokes unique mechanistic and functional outcomes on brain structure, and the combination of stimuli may lead to synergistic promotion of neuroplastic remodeling.
ACKNOWLEDGMENTS
The authors have no acknowledgments to report.
FUNDING
This research was supported by the National Institute on Alcohol Abuse and Alcoholism of the National Institutes of Health (R01AA27269) to AK. The content was solely the responsibility of the authors and does not necessarily represent the official views of the National Institutes of Health.
CONFLICT OF INTEREST
The authors have no conflict of interest to report.
REFERENCES
[1] | Swaab DF Brain aging and Alzheimer’s disease, “Wear and tear” versus “Use it or lose it, ” Neurobiol Aging (1991) ;12: (4):317–24. |
[2] | Shors TJ , Anderson ML , Curlik DM , Nokia MS Use it or lose it: How neurogenesis keeps the brain fit for learning, Behav Brain Res (2012) ;227: (2):450–8. |
[3] | Allred RP , Kim SY , Jones TA Use it and/or lose it-experience effects on brain remodeling across time after stroke, Front Hum Neurosci (2014) ;8: (JUNE):1–8. |
[4] | Greenough WT , Black JE , Wallace CS Experience and brain development, Child Dev (1987) ;58: (3):539–59. |
[5] | Pascual-Leone A , Amedi A , Fregni F , Merabet LB THE PLASTIC HUMAN BRAIN CORTEX. https://doi.org/101146/annurev.neuro27070203144216 [Internet] 2005 Jul 15 [cited 2023 Oct 6];28: 377–401. Available from: https://www.annualreviews.org/doi/abs/10.1146/annurev.neuro.27.070203.144216 |
[6] | Balietti M , Conti F Environmental enrichment and the aging brain: is it time for standardization? Neurosci Biobehav Rev. (2022) ;139: :104728. |
[7] | Burke SN , Barnes CA Neural plasticity in the ageing brain, Nat Rev Neurosci [Internet]. Jan [cited 2023 Oct 6]; 7: (1):30–40. Available from: https://pubmed.ncbi.nlm.nih.gov/16371948/ |
[8] | Diamond MC , Ingham CA , Johnson RE , Bennett EL , Rosenzweig MR Effects of environment on morphology of rat cerebral cortex and hippocampus. J Neurobiol [Internet] 1976 [cited 2023 Oct 6];7: (1):75–85. Available from: https://pubmed.ncbi.nlm.nih.gov/1249575/ |
[9] | Turner AM , Greenough WT Differential rearing effects on rat visual cortex synapses. I. Synaptic and neuronal density and synapses per neuron. Brain Res [Internet]. 1985 Mar 11 [cited 2023 Oct 6];329: (1-2):195-203. Available from: https://pubmed.ncbi.nlm.nih.gov/3978441/ |
[10] | Stillman CM , Cohen J , Lehman ME , Erickson KI Mediators of Physical Activity on Neurocognitive Function: A Review at Multiple Levels of Analysis. Front Hum Neurosci [Internet]. 2016 Dec 8 [cited 2023 Oct 6];10(DEC2016). Available from: https://pubmed.ncbi.nlm.nih.gov/28018195/ |
[11] | El-Sayes J , Harasym D , Turco C V , Locke MB , Nelson AJ Exercise-Induced Neuroplasticity: A Mechanistic Model and Prospects for Promoting Plasticity. Neuroscientist [Internet] 2019 Feb 1 [cited 2023 Oct 6];25: (1):65–85. Available from: https://journals.sagepub.com/doi/10.1177/1073858418771538 |
[12] | Gutierrez RMS , Ricci NA , Gomes QRS , Oliveira DL , Pires RS The effects of acrobatic exercise on brain plasticity: a systematic review of animal studies. Brain Struct Funct [Internet] 2018 Jun 1 [cited 2023 Oct 6];223: (5):2055–71. Available from: https://pubmed.ncbi.nlm.nih.gov/29480413/ |
[13] | Bettio L , Thacker JS , Hutton C , Christie BR Modulation of synaptic plasticity by exercise. Int Rev Neurobiol [Internet] 2019 Jan 1 [cited 2023 Oct 6];147: 295–322. Available2 from: https://pubmed.ncbi.nlm.nih.gov/31607359/ |
[14] | Fabel K , Fabel K , Tam B , Kaufer D , Baiker A , Simmons N , Kuo CJ , Palmer TD VEGF is necessary for exercise induced adult hippocampal neurogenesis. Eur J Neurosci [Internet] 2003 Nov [cited 2023 Oct 6];18: (10):2803–12. Available from: https://pubmed.ncbi.nlm.nih.gov/14656329/ |
[15] | Morland C , Andersson KA , Haugen ØP , Hadzic A , Kleppa L , Gille A , Rinholm JE , Palibrk V , Diget EH , Kennedy LH , Stølen T , Hennestad E , Moldestad O , Cai Y , Puchades M , Offermanns S , Vervaeke K , Bjørås M , Wisløff U , Storm-Mathisen J , Bergersen LH Exercise induces cerebral VEGF and angiogenesis via the lactate receptor HCAR1. Nat Commun [Internet]. 2017 May 23 [cited 2023 Oct 6];8. Available from: https://pubmed.ncbi.nlm.nih.gov/28534495/ |
[16] | Kwon D , Pfefferbaum A , Sullivan E V , Pohl KM Regional growth trajectories of cortical myelination in adolescents and young adults: longitudinal validation and functional correlates, Brain Imaging Behav (2020) ;14: (1):242–66. |
[17] | Hubel DH , Wiesel T Receptive fields, binocular interaction and functional architecture in the cat’s visual cortex. J Physiol. 1962;106-54. |
[18] | Nelson CA , Gabard-Durnam LJ Early Adversity and Critical Periods: Neurodevelopmental Consequences of Violating the Expectable Environment. Trends Neurosci [Internet] 2020;43: (3):133–43. Available from: https://www.sciencedirect.com/science/article/pii/S0166223620300035 |
[19] | Schmidt S , Gull S , Herrmann KH , Boehme M , Irintchev A , Urbach A , Reichenbach JR , Klingner CM , Gaser C , Witte OW Experience-dependent structural plasticity in the adult brain: Howthe learning brain grows. Neuroimage [Internet]. 2021 Jan 15 [cited 2023 Oct 6];225. Available from: https://pubmed.ncbi.nlm.nih.gov/33164876/ |
[20] | Bibollet-Bahena O , Tissier S , Ho-Tran S , Rojewski A , Casanova C Enriched environment exposure during development positively impacts the structure and function of the visual cortex in mice. Sci Reports 2023 131 [Internet] 2023 Apr 29 [cited 2023 Oct 5];13: (1):1–12. Available from: https://www.nature.com/articles/s41598-023-33951-0 |
[21] | Ho TC , King LS Mechanisms of neuroplasticity linking early adversity to depression: developmental considerations. Transl Psychiatry [Internet] 2021 Dec 1 [cited 2023 Oct 6];11: (1). Available from: https://pubmed.ncbi.nlm.nih.gov/34628465/ |
[22] | Bandeira ID , Lins-Silva DH , Barouh JL , Faria-Guimarães D , Dorea-Bandeira I , Souza LS , Alves GS , Brunoni AR , Nitsche M , Fregni F , Lucena R Neuroplasticity and non-invasive brain stimulation in the developing brain. Prog Brain Res [Internet] 2021 Jan 1 [cited 2023 Oct 6];264: 57–89. Available from: https://pubmed.ncbi.nlm.nih.gov/34167665/ |
[23] | Li J , Li Y , Sun Y , Wang H , Liu X , Zhao Y , Wang H , Su Y , Si T Chronic mild corticosterone exposure during adolescence enhances behaviors and upregulates neuroplasticity-related proteins in rat hippocampus. Prog Neuropsychopharmacol Biol Psychiatry [Internet] 2019 Mar 8 [cited 2023 Oct 6];89: 400–11. Available from: https://pubmed.ncbi.nlm.nih.gov/30392783/ |
[24] | Matsumoto H , Omata N , Kiyono Y , Mizuno T , Mita K , Kosaka H Paradoxical changes in mood-related behaviors on continuous social isolation after weaning. Exp brain Res [Internet] 2021 Aug 1 [cited 2023 Oct 6];239: (8):2537–50. Available from: https://pubmed.ncbi.nlm.nih.gov/34143240/ |
[25] | Gildawie KR , Ryll LM , Hexter JC , Peterzell S , Valentine AA , Brenhouse HC A two-hit adversity model in developing rats reveals sex-specific impacts on prefrontal cortex structure and behavior. Dev Cogn Neurosci [Internet]. 2021 Apr 1 [cited 2023 Oct 6];48. Available from: https://pubmed.ncbi.nlm.nih.gov/33515957/ |
[26] | Marinus N , Hansen D , Feys P , Meesen R , Timmermans A , Spildooren J The Impact of Different Types of Exercise Training on Peripheral Blood Brain-Derived Neurotrophic Factor Concentrations in Older Adults: A Meta-Analysis. Sport Med [Internet] 2019 Oct 1 [cited 2023 Apr 10];49: (10):1529–46. Available from: https://link.springer.com/article/10.1007/s40279-01901148-z |
[27] | Boda E , Di Maria S , Rosa P , Taylor V , Abbracchio MP , Buffo A Early phenotypic asymmetry of sister oligodendrocyte progenitor cells after mitosis and its modulation by aging and extrinsic factors. Glia [Internet] 2015 Feb 1 [cited 2023 Apr 10];63: (2):271–86. Available from: https://onlinelibrary.wiley.com/doi/full/10.1002/glia.22750 |
[28] | Grimm A , Eckert A Brain aging and neurodegeneration: from a mitochondrial point of view. J Neurochem [Internet] 2017 Nov 1 [cited 2023 Oct 6];143: (4):418–31. Available from: https://pubmed.ncbi.nlm.nih.gov/28397282/ |
[29] | Tarumi T , Tomoto T , Repshas J , Wang C , Hynan LS , Cullum CM , Zhu DC , Zhang R Midlife aerobic exercise and brain structural integrity: Associations with age and cardiorespiratory fitness, Neuroimage (2021) ;225: :117512. |
[30] | Park DC , Bischof GN The aging mind: Neuroplasticity in response to cognitive training, Dialogues Clin Neurosci (2013) ;15: (1):109–19. |
[31] | Kleim JA , Cooper NR , VandenBerg PM Exercise induces angiogenesis but does not alter movement representations within rat motor cortex, Brain Res (2002) ;934: (1):1–6. |
[32] | Van Praag H , Kempermann G , Gage FH Running increases cell proliferation and neurogenesis in the adult mouse dentate gyrus. Nat Neurosci [Internet] 1999 Mar [cited 2023 Oct 6];2: (3):266–70. Available from: https://pubmed.ncbi.nlm.nih.gov/10195220/ |
[33] | Krityakiarana W , Espinosa-Jeffrey A , Ghiani CA , Zhao PM , Topaldjikian N , Gomez-Pinilla F , Yamaguchi M , Kotchabhakdi N , De Vellis J Voluntary exercise increases oligodendrogenesis in spinal cord. Int J Neurosci [Internet] 2010 Apr [cited 2023 Oct 6];120: (4):280–90. Available from: https://pubmed.ncbi.nlm.nih.gov/20374076/ |
[34] | He XF , Liu DX , Zhang Q , Liang FY , Dai GY , Zeng JS , Pei Z , Xu GQ , Lan Y Voluntary exercise promotes glymphatic clearance of amyloid beta and reduces the activation of astrocytes and microglia in aged mice, Front Mol Neurosci (2017) ;10: :259843. |
[35] | Van Praag H , Shubert T , Zhao C , Gage FH Exercise enhances learning and hippocampal neurogenesis in aged mice. J Neurosci [Internet] 2005 Sep 21 [cited 2023 Oct 6];25: (38):86805. Available from: https://pubmed.ncbi.nlm.nih.gov/16177036/ |
[36] | Pereira AC , Huddleston DE , Brickman AM , Sosunov AA , Hen R , McKhann GM , Sloan R , Gage FH , Brown TR , Small SA An in vivo correlate of exercise induced neurogenesis in the adult dentate gyrus. Proc Natl Acad Sci U S A [Internet] 2007 Mar 27 [cited 2023 Oct 6];104: (13):5638–43. Available from: https://pubmed.ncbi.nlm.nih.gov/17374720/ |
[37] | Müller P , Duderstadt Y , Lessmann V , Müller NG Lactate and BDNF: Key Mediators of Exercise Induced Neuro plasticity. J Clin Med 2020, Vol 9, Page 1136 [Internet] 2020 Apr 15 [cited 2023 Sep 18];9: (4)1136. Available from: https://www.mdpi.com/2077-0383/9/4/1136/htm |
[38] | Matsunaga D , Nakagawa H , Ishiwata T Difference in the brain serotonin and its metabolite level and anxiety-like behavior between forced and voluntary exercise conditions in rats, Neurosci Lett (2021) ;744: :135556. |
[39] | Katsidoni V , Tzatzarakis MN , Karzi V , Thermos K , Kastellakis A , Panagis G Differential effects of chronic voluntary wheel-running on morphine induced brain stimulation reward, motor activity and striatal dopaminergic activity, Behav Brain Res (2020) ;394: :112831. |
[40] | Sato C , Tanji K , Shimoyama S , Chiba M , Mikami M , Koeda S , Sumigawa K , Akahira K , Yamada J Effects of voluntary and forced exercises on motor function recovery in intracerebral hemorrhage rats. Neuroreport [Internet] 2020 Jan 27 [cited 2023 Oct 5];31: (2)189–96. Available from: https://journals.lww.com/neuroreport/fulltext/2020/01020/effects_of_voluntary_and_forced_exercises_on_motor.15.aspx |
[41] | Lai Z , Shan W , Li J , Min J , Zeng X , Zuo Z Appropriate exercise level attenuates gut dysbiosis and valeric acid increase to improve neuroplasticity and cognitive function after surgery in mice. Mol Psychiatry 2021 2612 [Internet] 2021 Oct 18 [cited 2023 Sep 18];26: (12):7167–87. Available from: https://www.nature.com/articles/s41380-021-01291-y |
[42] | McKenzie IA , Ohayon D , Li H , De Faria JP , Emery B , Tohyama K , Richardson WD Motor skill learning requires active central myelination, Science (80-) (2014) ;346: (6207):318–22. |
[43] | Xin W , Chan JR Myelin plasticity: sculpting circuits in learning and memory. Nat Rev Neurosci [Internet] 2020;21: (12):682–94. Available from: http://dx.doi.org/10.1038/s41583-02000379-8 |
[44] | Bloom MS , Orthmann-Murphy J , Grinspan JB Motor Learning and Physical Exercise in Adaptive Myelination and Remyelination. ASN Neuro [Internet]. 2022 [cited 2023 May 22];14. Available from: /pmc/articles/PMC9158406/ |
[45] | Tomlinson L , Huang PH , Colognato H Prefrontal cortex NG2 glia undergo a developmental switch in their responsiveness to exercise. Dev Neurobiol [Internet] 2018 Jul 1 [cited 2023 Apr 11];78: (7):687–700. Available from: https://onlinelibrary.wiley.com/doi/full/10.1002/dneu.22590 |
[46] | Alvarez-Saavedra M , De Repentigny Y , Yang D , O’Meara RW , Yan K , Hashem LE , Racacho L , Ioshikhes I , Bulman DE , Parks RJ , Kothary R , Picketts DJ Voluntary Running Triggers VGF-Mediated Oligodendrogenesis to Prolong the Lifespan of Snf2h-Null Ataxic Mice, Cell Rep (2016) ;17: (3):862–75. |
[47] | Hall JM , Vetreno RP , Savage LM Differential cortical neurotrophin and cytogenetic adaptation after voluntary exercise in normal and amnestic rats. Neuroscience [Internet]. 2014 Jan 31 [cited 2023 Oct 6];258: :131–46. Available from: https://pubmed.ncbi.nlm.nih.gov/24215977/ |
[48] | Zheng J , Sun X , Ma C , Li BM , Luo F Voluntary wheel running promotes myelination in the motor cortex through Wnt signaling in mice. Mol Brain [Internet] 2019 Oct 24 [cited 2023 Apr 17];12: (1):1–10. Available from: https://molecularbrain.biomedcentral.com/articles/10.1186/s13041-019-0506-8 |
[49] | Ruotsalainen I , Gorbach T , Perkola J , Renvall V , Syväoja HJ , Tammelin TH , Karvanen J , Parviainen T Physical activity, aerobic fitness, and brain white matter: Their role for executive functions in adolescence, Dev Cogn Neurosci (2020) ;42: :100765. |
[50] | Herting MM , Colby JB , Sowell ER , Nagel BJ White matter connectivity and aerobic fitness in male adolescents, Dev Cogn Neurosci (2014) ;7: :65–75. |
[51] | Chaddock-Heyman L , Erickson KI , Kienzler C , Drollette ES , Raine LB , Kao SC , Bensken J , Weisshappel R , Castelli DM , Hillman CH , Kramer AF Physical Activity Increases White Matter Microstructure in Children, Front Neurosci (2018) ;12: (December):1–11. |
[52] | Schaeffer DJ , Krafft CE , Schwarz NF , Chi L , Rodrigue AL , Pierce JE , Allison JD , Yanasak NE , Liu T , Davis CL , McDowell JE An 8-month exercise intervention alters frontotemporal white matter integrity in overweight children. Psychophysiology [Internet] 2014 [cited 2023 Oct 6];51: (8):728–33. Available from: https://pubmed.ncbi.nlm.nih.gov/24797659/ |
[53] | Guo J , Bertalan G , Meierhofer D , Klein C , Schreyer S , Steiner B , Wang S , Vieira da Silva R , Infante-Duarte C , Koch S , Boehm-Sturm P , Braun J , Sack I Brain maturation is associated with increasing tissue stiffness and decreasing tissue fluidity, Acta Biomater (2019) ;99: :433–42. |
[54] | Alfini AJ , Weiss LR , Leitner BP , Smith TJ , Hagberg JM , Smith JC Hippocampal and cerebral blood flow after exercise cessation in master athletes, Front Aging Neurosci (2016) ;8: (AUG):184. |
[55] | Clark CM , Guadagni V , Mazerolle EL , Hill M , Hogan DB , Pike GB , Poulin MJ Effect of aerobic exercise on white matter microstructure in the aging brain, Behav Brain Res (2019) ;373: :112042. |
[56] | Chen L , Chao FL , Lu W , Zhang L , Huang CX , Yang S , Qiu X , Yang H , Zhao YY , Wang SR , Li C , Tang Y Long-Term Running Exercise Delays Age-Related Changes in White Matter in Rats, Front Aging Neurosci (2020) ;12: :359. |
[57] | Jensen SK , Michaels NJ , Ilyntskyy S , Keough MB , Kovalchuk O , Yong VW Multimodal Enhancement of Remyelination by Exercise with a Pivotal Role for Oligodendroglial PGC1α. Cell Rep [Internet] 2018 Sep 18 [cited 2023 Oct 7];24: (12):3167–79. Available from: https://pubmed.ncbi.nlm.nih.gov/30232000/ |
[58] | Milbocker KA , Smith IF , Brengel EK , LeBlanc GL , Roth TL , Klintsova AY Exercise in Adolescence Enhances Callosal White Matter Refinement in the Female Brain in a Rat Model of Fetal Alcohol Spectrum Disorders, Cells (2023) ;12: (7). |
[59] | Milbocker KA , LeBlanc GL , Brengel EK , Hekmatyar KS , Kulkarni P , Ferris CF , Klintsova AY Reduced and delayed myelination and volume of corpus callosum in an animal model of Fetal Alcohol Spectrum Disorders partially benefit from voluntary exercise. Sci Rep [Internet] 2022;12: (1):1–17. Available from: https://doi.org/10.1038/s41598-022-14752-3 |
[60] | Aagaard P , Bojsen-Møller J , Lundbye-Jensen J Assessment of neuroplasticity with strength training. Exerc Sport Sci Rev [Internet] 2020 Oct 1 [cited 2023 Apr 11];48: (4):151–62. Available from: https://journals.lww.com/acsmessr/Fulltext/2020/10000/Assessment_of_Neur_oplasticity_With_Strength.2.aspx |
[61] | Herold F , Törpel A , Schega L , Müller NG Functional and/or structural brain changes in response to resistance exercises and resistance training lead to cognitive improvements - a systematic review. Eur Rev Aging Phys Act 2019 161 [Internet] 2019 Jul 10 1381 [cited 2023 May 22];16: (1):1–33. Available from: https://link.springer.com/articles/10.1186/s11556-019-0217-2 |
[62] | Pinho RA , Aguiar AS , Radák Z Effects of resistance exercise on cerebral redox regulation and cognition: An interplay between muscle and brain. Vol. 8, Antioxidants. MDPI; 2019. |
[63] | Behm DG , Faigenbaum AD , Falk B , Klentrou P Canadian Society for Exercise Physiology position paper: Resistance training in children and adolescents. Vol. 33, Applied Physiology, Nutrition and Metabolism. 2008. p. 547-61. |
[64] | Palmer HS , Håberg AK , Fimland MS , Solstad GM , Iversen VM , Hoff J , Helgerud J , Eikenes L Structural brain changes after 4 wk of unilateral strength training of the lower limb. J Appl Physiol [Internet] 2013;115: 167–75. Available from: http://www.jappl.org |
[65] | Hendy AM , Lamon S The cross-education phenomenon: Brain and beyond. Vol. 8, Frontiers in Physiology. Frontiers Research Foundation; 2017. |
[66] | Bolandzadeh N , Tam R , Handy TC , Nagamatsu LS , Hsu CL , Davis JC , Dao E , Beattie BL , Liu-Ambrose T Resistance Training and White Matter Lesion Progression in Older Women: Exploratory Analysis of a 12-Month Randomized Controlled Trial. J Am Geriatr Soc [Internet] 2015 Oct 1 [cited 2023 May 22];63: (10):2052–60. Available from: https://onlinelibrary.wiley.com/doi/full/10.1111/jgs.13644 |
[67] | Hortobágyi T , Vetrovsky T , Balbim GM , Sorte Silva NCB , Manca A , Deriu F , Kolmos M , Kruuse C , Liu-Ambrose T , Radák Z , Váczi M , Johansson H , dos Santos PCR , Franzén E , Granacher U Theimpact of aerobic and resistance training intensity on markers ofneuroplasticity in health and disease, Ageing Res Rev (2022) ;80: :101698. |
[68] | Cassilhas RC , Viana VAR , Grassmann V , Santos RT , Santos RF , Tufik S , Mello MT The impact of resistance exercise on the cognitive function of the elderly, Med Sci Sports Exerc (2007) ;39: (8):1401–7. |
[69] | Kjølhede T , Siemonsen S , Wenzel D , Stellmann JP , Ringgaard S , Pedersen BG , Stenager E , Petersen T , Vissing K , Heesen C , Dalgas U Can resistance training impact MRI outcomes in relapsing-remitting multiple sclerosis? Mult Scler [Internet]. 2018 Sep 1 [cited 2023 Oct 7];24: (10):1356–65. Available from: https://pubmed.ncbi.nlm.nih.gov/28752800/ |
[70] | amiri noosha , Moazzami M , Yaghoubi A Effect of Eight -Weeks of Resistance Training on Serum Levels of Neurofilament Light Chain and Tau Protein in Women with Multiple Sclerosis. Med Lab J [Internet] 2021 Jul 1 [cited 2023 May 22];15: (4):33–8. Available from: http://mlj.goums.ac.ir/article-1-1315-en.html |
[71] | Mockford M , Caulton JM Systematic review of progressive strength training in children and adolescents with cerebral palsy who are ambulatory, Pediatr Phys Ther (2008) ;20: (4):31833. |
[72] | Nishijima T , Kamidozono Y , Ishiizumi A , Amemiya S , Kita I Negative rebound in hippocampal neurogenesis following exercise cessation. Am J Physiol - Regul Integr Comp Physiol [Internet]. 2017 Mar 1 [cited 2023 Apr 11];312: (3):R347–57. Available from: https://journals.physiology.org/doi/10.1152/ajpregu.00397.2016 |
[73] | Hosp JA , Luft AR Cortical plasticity during motor learning and recovery after ischemic stroke. Neural Plast [Internet]. 2011 [cited 2023 Oct 7];2011. Available from: https://pubmed.ncbi.nlm.nih.gov/22135758/ |
[74] | Hirano T Regulation and Interaction of Multiple Types of Synaptic Plasticity in a Purkinje Neuron and Their Contribution to Motor Learning. Cerebellum [Internet] 2018 Dec 1 [cited 2023 Oct 7];17: (6):756–65. Available from: https://pubmed.ncbi.nlm.nih.gov/29995220/ |
[75] | Papale AE , Hooks BM Circuit changes in motor cortex during motor skill learning. Neuroscience [Internet]. 2018 Jan 1 [cited 2023 Oct 7];368: :283–97. Available from: https://pubmed.ncbi.nlm.nih.gov/28918262/ |
[76] | Wood AN New roles for dopamine in motor skill acquisition: lessons from primates, rodents, and songbirds. J Neurophysiol [Internet]. 2021 Jun 1 [cited 2023 Oct 7];125: (6):236174. Available from: https://pubmed.ncbi.nlm.nih.gov/33978497/ |
[77] | Black JE , Isaacs KR , Anderson BJ , Alcantara AA , Greenough WT Learning causes synaptogenesis, whereas motor activity causes angiogenesis, in cerebellar cortex of adult rats. Proc Natl Acad Sci U S A [Internet] 1990 Jul 1 [cited 2019 Jan 15];87: (14):5568–72. Available from: http://www.ncbi.nlm.nih.gov/pubmed/1695380 |
[78] | Jones TA , Chu CJ , Grande LA , Gregory AD Motor Skills Training Enhances Lesion Induced Structural Plasticity in the Motor Cortex of Adult Rats. J Neurosci [Internet] 1999 Nov 15 [cited 2023 Oct 5];19: (22):10153–63. Available from: https://www.jneurosci.org/content/19/22/10153 |
[79] | Confortim HD , Deniz BF , de Almeida W , Miguel PM , Bronauth L , Vieira MC , de Oliveira BC , Pereira LO Neonatal hypoxia-ischemia caused mild motor dysfunction, recovered by acrobatic training, without affecting morphological structures involved in motor control in rats, Brain Res (1707) )27–44. |
[80] | Kleim JA , Swain RA , Armstrong KA , Napper RMA , Jones TA , Greenough WT Selective synaptic plasticity within the cerebellar cortex following complex motor skill learning, Neurobiol Learn Mem (1998) ;69: (3):274–89. |
[81] | Nudo RJ , Milliken GW Reorganization of movement representations in primary motor cortex following focal ischemic infarcts in adult squirrel monkeys. J Neurophysiol [Internet]. 1996 [cited 2023 Oct 7];75(5):2144-9.Available from: https://pubmed.ncbi.nlm.nih.gov/8734610/ |
[82] | Kleim JA , Hogg TM , VandenBerg PM , Cooper NR , Bruneau R , Remple M Cortical synaptogenesis and motor map reorganization occur during late, but not early, phase of motor skill learning. J Neurosci [Internet] 2004 Jan 21 [cited 2023 Oct 7];24: (3):628–33. Available from: https://pubmed.ncbi.nlm.nih.gov/14736848/ |
[83] | Wang L , Conner JM , Rickert J , Tuszynski MH Structural plasticity within highly specific neuronal populations identifies a unique parcellation of motor learning in the adult brain. Proc Natl Acad Sci U S A [Internet] 2011 Feb 8 [cited 2023 Oct 7];108: (6):2545–50. Available from: https://pubmed.ncbi.nlm.nih.gov/21257908/ |
[84] | Mediavilla T , Özalay Ö , Estévez-Silva HM , Frias B , Orädd G , Sultan FR , Brozzoli C , Garzón B , Lövdén M , Marcellino DJ Learning-related contraction of gray matter in rodent sensorimotor cortex is associated with adaptive myelination. Elife [Internet]. 2022 Nov 1 [cited 2023 Oct 7];11. Available from: https://pubmed.ncbi.nlm.nih.gov/36350292/ |
[85] | Keiner S , Niv F , Neumann S , Steinbach T , Schmeer C , Hornung K , Schlenker Y , Förster M , Witte OW , Redecker C Effect of skilled reaching training and enriched environment on generation of oligodendrocytes in the adult sensorimotor cortex and corpus callosum. BMCNeurosci [Internet]. 2017 Mar 9 [cited 2023 Apr 10];18: (1):1–13. Available from: https://link.springer.com/articles/10.1186/s12868-017-0347-2 |
[86] | Hwang FJ , Roth RH , Wu YW , Sun Y , Kwon DK , Liu Y , Ding JB Motor learning selectively strengthens cortical and striatal synapses of motor engram neurons. Neuron [Internet]. 2022 Sep 7 [cited 2023 Oct 7];110: (17):2790–2801.e5. Available from: http://www.cell.com/article/S089662732200544X/fulltext |
[87] | Streffing-Hellhake P , Luft AR , Hosp JA Motor Learning Induces Profound but Delayed Dendritic Plasticity in M1 Layer II/III Pyramidal Neurons. Neuroscience [Internet]. 2020 Aug 21 [cited 2023 Oct 7];442: :17–28. Available from: https://pubmed.ncbi.nlm.nih.gov/32634528/ |
[88] | Chen SX , Kim AN , Peters AJ , Komiyama T Subtypespecific plasticity of inhibitory circuits in motor cortex during motor learning. Nat Neurosci [Internet] 2015 Aug 30 [cited 2023 Oct 7];18: (8):1109–15. Available from: https://pubmed.ncbi.nlm.nih.gov/26098758/ |
[89] | Klintsova AY , Dickson E , Yoshida R , Greenough WT Altered expression of BDNF and its high-affinity receptor TrkB in response to complex motor learning and moderate exercise. Brain Res [Internet] 2004 Nov 26 [cited 2019 Jan 15];1028: (1):92–104. Available from: https://www.sciencedirect.com/science/article/pii/S0006899304015008 |
[90] | Kim HT , Kim IH , Lee KJ , Lee JR , Park SK , Chun YH , Kim H , Rhyu IJ Specific plasticity of parallel fiber/Purkinje cell spine synapses by motor skill learning. Neuroreport [Internet] 2002 Sep 16 [cited 2023 Oct 7];13: (13):1607–10. Available from: https://pure.korea.ac.kr/en/publications/specific-plasticity-of-parallel-fiberpurkinje-cell-spine-synapses |
[91] | Sampaio ASB , Real CC , Gutierrez RMS , Singulani MP , Alouche SR , Britto LR , Pires RS Neuroplasticity induced by the retention period of a complex motor skill learning in rats. Behav Brain Res [Internet]. 2021 Sep 24 [cited 2023 Oct 7];414. Available from: https://pubmed.ncbi.nlm.nih.gov/34302881/ |
[92] | Gursky ZH , Klintsova AY Wheel Running and Environmental Complexity as a Therapeutic Intervention in an Animal Model of FASD. J Vis Exp [Internet]. 2017 [cited 2019 Dec 3];(120):54947. Available from: www.jove.comurl:https://www.jove.com/video/54947 |
[93] | Ramírez-Rodríguez GB , Gutiérrez-Vera B , Ortiz-LópezL , Vega-Rivera NM , Juan DMS , Granados-Juárez A , Aquino de DVC , Castro-García M , Ramos MF Environmental enrichment: dissociated effects between physical activity and changing environmental complexity on anxiety and neurogenesis in adult male Balb/C mice. Physiol Behav [Internet]. 2022 Oct 1 [cited 2023 Oct 5];254. Available from: https://pubmed.ncbi.nlm.nih.gov/35700814/ |
[94] | Grońska-Peski M , Goncalves J T , Hébert JM Enriched Environment Promotes Adult Hippocampal Neurogenesis through FGFRs. J Neurosci [Internet] 2021 Mar 31 [cited 2023 Oct 5];41: (13):2899–910. Available from: https://pubmed.ncbi.nlm.nih.gov/33637561/ |
[95] | Yamada J , Nadanaka S , Kitagawa H , Takeuchi K , Jinno S Increased Synthesis of Chondroitin Sulfate Proteoglycan Promotes Adult Hippocampal Neurogenesis in Response to Enriched Environment. J Neurosci [Internet] 2018 Sep 26 [cited 2023 Oct 5];38: (39):8496–513. Available from: https://www.jneurosci.org/content/38/39/8496 |
[96] | Zarif H , Nicolas S , Guyot M , Hosseiny S , Lazzari A , Canali MM , Cazareth J , Brau F , Golzné V , Dourneau E , Maillaut M , Luci C , Paquet A , Lebrigand K , Arguel MJ , Daoudlarian D , Heurteaux C , Glaichenhaus N , Chabry J , Guyon A , Petit-Paitel A CD8+T cells are essential for the effects of enriched environment on hippocampus-dependent behavior, hippocampal neurogenesis and synaptic plasticity. Brain Behav Immun. (2018) ;69: :235–54. |
[97] | Singhal G , Morgan J , Jawahar MC , Corrigan F , Jaehne EJ , Toben C , Breen J , Pederson SM , Hannan AJ , Baune BT Short-term environmental enrichment, and not physical exercise, alleviate cognitive decline and anxiety from middle age onwards without affecting hippocampal gene expression. Cogn Affect Behav Neurosci [Internet]. 2019 Oct 1 [cited 2023 Oct 5];19: (5):1143–69. Available from: https://pubmed.ncbi.nlm.nih.gov/31463713/ |
[98] | Kempermann G Environmental enrichment, new neurons and the neurobiology of individuality. Nat Rev Neurosci [Internet] 2019 Apr 1 [cited 2023 Oct 7];20: (4):235–45. Available from: https://pubmed.ncbi.nlm.nih.gov/30723309/1594 |
[99] | Loisy M , Farah A , Fafouri A , Fanton A , Ahmadi M , Therreau L , Chevaleyre V , Piskorowski RA Environmental enrichment and social isolation modulate inhibitory transmission and plasticity in hippocampal area CA2. Hippocampus [Internet] 2023 Mar 1 [cited 2023 Oct 5];33: (3):197–207. Available from: https://onlinelibrary.wiley.com/doi/full/10.1002/hipo.23478 |
[100] | Dahlmanns M , Dahlmanns JK , Schmidt CC , Valero-Aracama MJ , Zheng F , Alzheimer C Environmental enrichment recruits activin A to recalibrate neural activity in mouse hippocampus. Cereb Cortex [Internet] 2023 Jan 5 [cited 2023 Oct 5];33: (3):663–75. Available from: https://dx.doi.org/10.1093/cercor/bhac092 |
[101] | Nicholson M , Wood RJ , Gonsalvez DG , Hannan AJ , Fletcher JL , Xiao J , Murray SS Remodelling of myelinated axons and oligodendrocyte differentiation is stimulated by environmental enrichment in the young adult brain. Eur J Neurosci [Internet] 2022 Dec 1 [cited 2023 Oct 5];56: (12):6099–114. Available from: https://pubmed.ncbi.nlm.nih.gov/36217300/ |
[102] | Borgmeyer M , Coman C , Has C , Schött HF , Li T , Westhoff P , Cheung YFH , Hoffmann N , Yuanxiang PA , Behnisch T , Gomes GM , Dumenieu M , Schweizer M , Chocholousková M , Holcapek M , Mikhaylova M , Kreutz MR , Ahrends R Multiomics of synaptic junctions revealsaltered lipid metabolism and signaling following environmentalenrichment, Cell Rep (2021) ;37: (1):109797. |
[103] | Van Praag H , Christie BR , Sejnowski TJ , Gage FH Running enhances neurogenesis, learning, and long-term potentiation in mice, Proc Natl Acad Sci U S A (1999) ;96: (23):13427–31. |
[104] | Steiner B , Zurborg S , Hörster H , Fabel K , Kempermann G Differential 24 h responsiveness of Prox1-expressing precursor cells in adult hippocampal neurogenesis to physical activity, environmental enrichment, and kainic acid-induced seizures. Neuroscience [Internet] 2008 Jun 23 [cited 2023 Oct 7];154: (2):521–9. Available from: https://pubmed.ncbi.nlm.nih.gov/18502050/ |
[105] | Fabel K , Wolf SA , Ehninger D , Babu H , Leal-Galicia P , Kempermann G Additive effects of physical exercise and environmental enrichment on adult hippocampal neurogenesis in mice. Front Neurosci [Internet]. 2009 [cited 2023 Oct 7];3(NOV). Available from: https://pubmed.ncbi.nlm.nih.gov/20582277/ |
[106] | Moreno-Jiménez EP , Jurado-Arjona J , Avila J , Martín ML The Social Component of Environmental Enrichment Is a Pro-neurogenic Stimulus in Adult c57BL6 Female Mice. Front cell Dev Biol [Internet]. 2019 [cited 2023 Oct 7];7(APR). Available from: https://pubmed.ncbi.nlm.nih.gov/31080799/ |
[107] | Watanasriyakul WT , Normann MC , Akinbo OI , Colburn W , Dagner A , Grippo AJ Protective neuroendocrine effects of environmental enrichment and voluntary exercise against social isolation: evidence for mediation by limbic structures. Stress [Internet] 2019 [cited 2023 Oct 7];22: (5):603–18. Available from: https://pubmed.ncbi.nlm.nih.gov/31134849/ |
[108] | Brenes JC , Fornaguera J , Sequeira-Cordero A Environmental Enrichment and Physical Exercise Attenuate the Depressive-Like Effects Induced by Social Isolation Stress in Rats. Front Pharmacol [Internet]. 2020 May 29 [cited 2023 Oct 7];11. Available from: https://pubmed.ncbi.nlm.nih.gov/32547399/ |
[109] | Mansk LMZ , Jaimes LF , Dias TL , Pereira GS Social recognition memory differences between mouse strains: On the effects of social isolation, adult neurogenesis, and environmental enrichment. Brain Res. (2023) ;1819: :148535. |
[110] | Melani R , Chelini G , Cenni MC , Berardi N Enriched environment effects on remote object recognition memory. Neuroscience [Internet] 2017 Jun 3 [cited 2023 Oct 7];352: :296–305. Available from: https://pubmed.ncbi.nlm.nih.gov/28412502/ |
[111] | González-Pardo H , Arias JL , Vallejo G , Conejo NM Environmental enrichment effects after early stress on behavior and functional brain networks in adult rats. PLoS One [Internet]. 2019 Dec 1 [cited 2023 Oct 7];14: (12).Available from: https://pubmed.ncbi.nlm.nih.gov/31830106/ |
[112] | Forbes TA , Goldstein EZ , Dupree JL , Jablonska B , Scafidi J , Adams KL , Imamura Y , Hashimoto-Torii K , Gallo V Environmental enrichment ameliorates perinatal brain injury and promotes functional white matter recovery. Nat Commun [Internet] 2020 Dec 1 [cited 2023 Oct 7];11: (1). Available from: https://pubmed.ncbi.nlm.nih.gov/32075970/ |
[113] | Durán-Carabali LE , Arcego DM , Odorcyk FK , Reichert L , Cordeiro JL , Sanches EF , Freitas LD , Dalmaz C , Pagnussat A , Netto CA Prenatal and Early Postnatal Environmental Enrichment Reduce Acute Cell Death and Prevent Neurodevelopment and Memory Impairments in Rats Submitted to Neonatal Hypoxia Ischemia. Mol Neurobiol [Internet] 2018 May 1 [cited 2023 Oct 7];55: (5):3627–41. Available from: https://pubmed.ncbi.nlm.nih.gov/28523564/ |
[114] | Goncalves LV , Herlinger AL , Ferreira TAA , Coitinho JB , Pires RGW , Martins-Silva C Environmental enrichment cognitive neuroprotection in an experimental model of cerebral ischemia: biochemical and molecular aspects. Behav Brain Res [Internet] 2018 Aug 1 [cited 2023 Oct 7];348: :171–83. Available from: https://pubmed.ncbi.nlm.nih.gov/29684474/ |
[115] | Grech AM , Ratnayake U , Hannan AJ , van den Buuse M , Hill RA Sex-dependent effects of environmental enrichment on spatial memory and brain-derived neurotrophic factor (BDNF) signaling in a developmental “two-hit” mouse model combining bdnf haploinsufficiency and chronic glucocorticoid stimulation. Front Behav Neurosci. (2018) ;12: :394724. |
[116] | Zajac MS , Renoir T , Perreau VM , Li S , Adams W , van den Buuse M , Hannan AJ Short Term Environmental Stimulation Spatiotemporally Modulates Specific Serotonin Receptor Gene Expression and Behavioral Pharmacology in a Sexually Dimorphic Manner in Huntington’s Disease Transgenic Mice. Front Mol Neurosci [Internet]. 2018 Dec 10 [cited 2023 Oct 5];11. Available from: https://pubmed.ncbi.nlm.nih.gov/30618600/ |
[117] | Wang XM , Pan W , Xu N , Zhou ZQ , Zhang GF , Shen JC Environmental enrichment improves long-term memory impairment and aberrant synaptic plasticity by BDNF/TrkB signaling in nerve-injured mice, Neurosci Lett (2019) ;694: :93–8. |
[118] | Gualtieri F , Brégère C , Laws GC , Armstrong EA , Wylie NJ , Moxham TT , Guzman R , Boswell T , Smulders T V Effects of Environmental Enrichment on Doublecortin and BDNF Expression along the Dorso-Ventral Axis of the Dentate Gyrus. Front Neurosci [Internet]. 2017 Sep 15 [cited 2023 Oct 7];11(SEP). Available from: https://pubmed.ncbi.nlm.nih.gov/28966570/ |
[119] | Coutens B , Lejards C , Bouisset G , Verret L , Rampon C , Guiard BP Enriched environmental exposure reduces the onset of action of the serotonin norepinephrin reuptake inhibitor venlafaxine through its effect on parvalbumin interneurons plasticity in mice. Transl Psychiatry 2023 131 [Internet] 2023 Jun 26 [cited 2023 Oct 7];13: (1):1–10. Available from: https://www.nature.com/articles/s41398-023-02519-x |
[120] | Hamilton GF , Boschen KE , Goodlett CR , Greenough WT , Klintsova AY Housing in Environmental Complexity Following Wheel Running Augments Survival of Newly Generated Hippocampal Neurons in a Rat Model of Binge Alcohol Exposure During the Third Trimester Equivalent, Alcohol Clin Exp Res (2012) ;36: (7):1196–204. |
[121] | Gursky ZH , Klintsova AY Wheel Running and Environmental Complexity as a Therapeutic Intervention in an Animal Model of FASD. J Vis Exp [Internet]. 2017 [cited 2019 Dec 3];(120):54947. Available from: www.jove.comurl:https://www.jove.com/video/54947 |
[122] | Milbocker KA , Klintsova AY Examination of cortically projecting cholinergic neurons following exercise and environmental intervention in a rodent model of fetal alcohol spectrum disorders. Birth Defects Res. 2020;1-15. |
[123] | Ballesteros S , Voelcker-Rehage C , Bherer L Editorial: Cognitive and brain plasticity induced by physical exercise, cognitive training, video games, and combined interventions. Vol. 12, Frontiers in Human Neuroscience. Frontiers Media S. A; 2018. |
[124] | Norouzi-Gheidari N , Archambault PS , Monte-Silva K , Kairy D , Sveistrup H , Trivino M , Levin MF , Milot MH Feasibility and preliminary efficacy of a combined virtual reality, robotics and electrical stimulation intervention in upper extremity stroke rehabilitation, J Neuroeng Rehabil (2021) ;18: (1). |
[125] | Li XL , Tao X , Li TC , Zhu ZM , Huang PL , Gong WJ Cognitive-exercise dual-task intervention ameliorates cognitive decline in natural aging rats through reducing oxidative stress and enhancing synaptic plasticity. Exp Gerontol [Internet]. 2022 Nov 1 [cited 2023 Oct 7];169. Available from: https://pubmed.ncbi.nlm.nih.gov/36270545/ |
[126] | Klein AB , Williamson R , Santini MA , Clemmensen C , Ettrup A , Rios M , Knudsen GM , Aznar S Blood BDNF concentrations reflect brain-tissue BDNF levels across species, Int J Neuropsychopharmacol (2011) ;14: (3):347–53. |
[127] | Andrews SC , Curtin D , Hawi Z , Wongtrakun J , Stout JC , Coxon JP Intensity Matters: High-intensity Interval Exercise Enhances Motor Cortex Plasticity More Than Moderate Exercise, Cereb Cortex (2020) ;30: (1):101–12. |
[128] | Zhan Y , Li MZ , Yang L , Feng XF , Lei JF , Zhang N , Zhao YY , Zhao H The three-phase enriched environment paradigm promotes neurovascular restorative and prevents learning impairment after ischemic stroke in rats, Neurobiol Dis (2020) ;146: :105091. |
[129] | Brannen DE , Wynn S , Shuster J , Howell M Pandemic Isolation and Mental Health Among Children. Disaster Med Public Health Prep [Internet] 2023 Jan 11 [cited 2023 Oct 7];17: (3). Available from: https://pubmed.ncbi.nlm.nih.gov/36628622/ |
[130] | Brandt L , Liu S , Heim C , Heinz A The effects of social isolation stress and discrimination on mental health. Transl Psychiatry [Internet] 2022 Dec 1 [cited 2023 Oct 7];12: (1). Available from: https://pubmed.ncbi.nlm.nih.gov/36130935/ |
[131] | Orben A , Tomova L , Blakemore SJ The effects of social deprivation on adolescent development and mental health. Lancet Child Adolesc Heal [Internet] 2020 Aug 1 [cited 2023 Oct 7];4: (8):634–40. Available from: https://pubmed.ncbi.nlm.nih.gov/32540024/ |
[132] | Magson NR , Freeman JYA , Rapee RM , Richardson CE , Oar EL , Fardouly J Protective Factors for Prospective Changes in Adolescent Mental Health during the COVID-19 Pandemic. J Youth Adolesc [Internet] 2021 Jan 1 [cited 2023 Oct 7];50: (1):44–57. Available from: https://pubmed.ncbi.nlm.nih.gov/33108542/ |
[133] | Mikics É , Guirado R , Umemori J , Tóth M , Biró L , Miskolczi C , Balázsfi D , Zelena D , Castrén E , Haller J , Karpova NN Social Learning Requires Plasticity Enhanced by Fluoxetine Through Prefrontal Bdnf-TrkB Signaling to Limit Aggression Induced by Post-Weaning Social Isolation. Neuropsychopharmacology [Internet] 2018 [cited 2023 Oct 7];43: (2):235–45. Available from: https://pubmed.ncbi.nlm.nih.gov/28685757/ |
[134] | Begni V , Sanson A , Pfeiffer N , Brandwein C , Inta D , Talbot SR , Riva MA , Gass P , Mallien AS Social isolation in rats: Effects on animal welfare and molecular markers for neuroplasticity. PLoS One [Internet] 2020 Oct 1 [cited 2023 Oct 7];15: (10). Available from: https://pubmed.ncbi.nlm.nih.gov/33108362/ |
[135] | Gong WG , Wang YJ , Zhou H , Li XL , Bai F , Ren QG , Zhang ZJ Citalopram Ameliorates Synaptic Plasticity Deficits in Different Cognition-Associated Brain Regions Induced by Social Isolation in Middle-Aged Rats. Mol Neurobiol [Internet] 2017 Apr 1 [cited 2023 Oct 7];54: (3):1927–38. Available from: https://pubmed.ncbi.nlm.nih.gov/26899575/ |
[136] | Yamamuro K , Yoshino H , Ogawa Y , Makinodan M , Toritsuka M , Yamashita M , Corfas G , Kishimoto T Social Isolation During the Critical Period Reduces Synaptic and Intrinsic Excitability of a Subtype of Pyramidal Cell in Mouse Prefrontal Cortex. Cereb Cortex [Internet] 2018 Mar 1 [cited 2023 Oct 7];28: (3):998–1010. Available from: https://dx.doi.org/10.1093/cercor/bhx010 |
[137] | Yamamuro K , Bicks LK , Leventhal MB , Kato D , Im S , Flanigan ME , Garkun Y , Norman KJ , Caro K , Sadahiro M , Kullander K , Akbarian S , Russo SJ , Morishita H A prefrontal paraventricular thalamus circuit requires juvenile social experience to regulate adult sociability in mice. Nat Neurosci [Internet] 2020 Oct 1 [cited 2023 Oct 7];23: (10):1240–52. Available from: https://pubmed.ncbi.nlm.nih.gov/32868932/ |
[138] | Shan Q , Yu X , Tian Y Adolescent social isolation shifts the balance of decision-making strategy from goal-directed action to habitual response in adulthood via suppressing the excitatory neurotransmission onto the direct pathway of the dorsomedial striatum. Cereb Cortex [Internet] 2023 Mar 1 [cited 2023 Oct 7];33: (5):1595–609. Available from: https://pubmed.ncbi.nlm.nih.gov/35524719/ |
[139] | Li X , Sun H , Zhu Y , Wang F , Wang X , Han L , et al. Dysregulation of prefrontal parvalbumin interneurons leads to adult aggression induced by social isolation stress during adolescence, Front. Mol. Neurosci (2022) ;15: :1010152. |
[140] | Cuesta S , Funes A , Pacchioni AM Social Isolation in Male Rats During Adolescence Inhibits the Wnt/β-Catenin Pathway in the Prefrontal Cortex and Enhances Anxiety and Cocaine-Induced Plasticity in Adulthood. Neurosci Bull [Internet] 2020 Jun 1 [cited 2023 Oct 7];36: (6):611–24. Available from: https://pubmed.ncbi.nlm.nih.gov/32078732/ |
[141] | McLeod F , Salinas PC Wnt proteins as modulators of synaptic plasticity, Curr Opin Neurobiol (2018) ;53: :90–5. |
[142] | Narvaes RF , Furini CRG Role ofWntsignaling in synaptic plasticity and memory. Neurobiol Learn Mem [Internet]. 2022 Jan 1 [cited 2023 Oct 7];187. Available from: https://pubmed.ncbi.nlm.nih.gov/34808336/ |
[143] | Biggio F , Mostallino MC , Talani G , Locci V , Mostallino R , Calandra G , Sanna E , Biggio Social enrichment reverses the isolation-induced deficits of neuronal plasticity in the hippocampus of male rats. Neuropharmacology [Internet]. 2019;151: (March):45–54. Available from: https://doi.org/10.1016/j.neuropharm.2019.03.030 |
[144] | Popa N , Boyer F , Jaouen F , Belzeaux R , Gascon E Social Isolation and Enrichment Induce Unique miRNA Signatures in the Prefrontal Cortex and Behavioral Changes in Mice. iScience [Internet] 2020 Dec 12 [cited 2023 Oct 5];23: (12). Available from: /pmc/articles/PMC7701176/ |
[145] | Sukegawa M , Yoshihara T , Hou S , Asano M , Hannan AJ , Wang DO Long-lasting housing environment manipulation and acute loss of environmental enrichment impact BALB/c mice behaviour in multiple functional domains. Eur J Neurosci [Internet] 2022 Mar 1 [cited 2023 Oct 5];55: (5):1118–40. Available from: https://onlinelibrary.wiley.com/doi/full/10.1111/ejn.15602 |
[146] | Xiong Y , Hong H , Liu C , Zhang YQ Social isolation and the brain: effects and mechanisms. Mol Psychiatry [Internet] 2023 Jan 1 [cited 2023 Oct 7];28: (1):191–201. Available from: https://pubmed.ncbi.nlm.nih.gov/36434053/ |
[147] | Fraser SA , Li KZH , Berryman N , Desjardins-Crépeau L , Lussier M , Vadaga K , Lehr L , Vu TTM , Bosquet L , Bherer L Does Combined Physical and Cognitive Training Improve Dual-Task Balance and Gait Outcomes in Sedentary Older Adults? Front Hum Neurosci [Internet]. 2017 Jan 18 [cited 2023 Oct 7];10. Available from: https://pubmed.ncbi.nlm.nih.gov/28149274/ |
[148] | Caeyenberghs K , Clemente A , Imms P , Egan G , Hocking DR , Leemans A , Metzler Baddeley C , Jones DK , Wilson PH Evidence for Training-Dependent Structural Neuroplasticity in Brain-Injured Patients: A Critical Review. Neurorehabil Neural Repair [Internet] 2018 Feb 1 [cited 2023 Oct 7];32: (2):99–114. Available from: https://pubmed.ncbi.nlm.nih.gov/29357743/ |
[149] | Tooley UA , Bassett DS , Mackey AP Environmental influences on the pace of brain development. Nat Rev Neurosci [Internet] 2021 Jun 1 [cited 2023 Oct 7];22: (6):372–84. Available from: https://pubmed.ncbi.nlm.nih.gov/33911229/ |
[150] | Sheridan MA , Peverill M , Finn AS , McLaughlin KA Dimensions of childhood adversity have distinct associations with neural systems underlying executive functioning. Dev Psychopathol 1901 [Internet] 2017 Dec 1 [cited 2023 Oct 7];29: (5):1777–94. Available from: https://pubmed.ncbi.nlm.nih.gov/29162183/ |
[151] | Amso D , Salhi C , Badre D The relationship between cognitive enrichment and cognitive control: A systematic investigation of environmental influences on development through socioeconomic status. Dev Psychobiol [Internet] 2019 Mar 1 [cited 2023 Oct 7];61: (2):159–78. Available from: https://pubmed.ncbi.nlm.nih.gov/30375651/ |
[152] | Cramer SC , Sur M , Dobkin BH , O’Brien C , Sanger TD , Trojanowski JQ , Rumsey JM , Hicks R , Cameron J , Chen D , Chen WG , Cohen LG , Decharms C , Duffy CJ , Eden GF , Fetz EE , Filart R , Freund M , Grant SJ , Haber S , Kalivas PW , Kolb B , Kramer AF , Lynch M , Mayberg HS , McQuillen PS , Nitkin R , Pascual-Leone A , Reuter-Lorenz P , Schiff N , Sharma A , Shekim L , Stryker M , Sullivan E V , Vinogradov S. Harnessing neuroplasticity for clinical applications. Brain [Internet]. 2011 Jun [cited 2023 Oct 7];134: (Pt 6):1591–609. Available from: https://pubmed.ncbi.nlm.nih.gov/21482550/ |
[153] | Wilkinson ST , Holtzheimer PE , Gao S , Kirwin DS , Price RB Leveraging Neuroplasticity to Enhance Adaptive Learning: The Potential for Synergistic Somatic Behavioral Treatment Combinations to Improve Clinical Outcomes in Depression. Biol Psychiatry [Internet] 2019 Mar 15 [cited 2023 Oct 7];85: (6):454–65. Available from: https://pubmed.ncbi.nlm.nih.gov/30528745/ |