Microbiota-Gut-Brain Axis Regulation of Adult Hippocampal Neurogenesis
Abstract
The birth, maturation, and integration of new neurons in the adult hippocampus regulates specific learning and memory processes, responses to stress, and antidepressant treatment efficacy. This process of adult hippocampal neurogenesis is sensitive to environmental stimuli, including peripheral signals from certain cytokines, hormones, and metabolites, which can promote or hinder the production and survival of new hippocampal neurons. The trillions of microorganisms resident to the gastrointestinal tract, collectively known as the gut microbiota, also demonstrate the ability to modulate adult hippocampal neurogenesis. In doing so, the microbiota-gut-brain axis can influence brain functions regulated by adult hippocampal neurogenesis. Unlike the hippocampus, the gut microbiota is highly accessible to direct interventions, such as prebiotics, probiotics, and antibiotics, and can be manipulated by lifestyle choices including diet. Therefore, understanding the pathways by which the gut microbiota shapes hippocampal neurogenesis may reveal novel targets for non-invasive therapeutics to treat disorders in which alterations in hippocampal neurogenesis have been implicated. This review first outlines the factors which influence both the gut microbiome and adult hippocampal neurogenesis, with cognizance that these effects might happen either independently or due to microbiota-driven mechanisms. We then highlight approaches for investigating the regulation of adult hippocampal neurogenesis by the microbiota-gut-brain axis. Finally, we summarize the current evidence demonstrating the gut microbiota’s ability to influence adult hippocampal neurogenesis, including mechanisms driven through immune pathways, microbial metabolites, endocrine signalling, and the nervous system, and postulate implications for these effects in disease onset and treatment.
1Adult hippocampal neurogenesis
1.1Introduction to adult hippocampal neurogenesis
The hippocampus is one of only two regions of the mammalian brain where new neurons are produced throughout life via a process called hippocampal neurogenesis which occurs in the neurogenic niche of the subgranular zone (SGZ) within the dentate gyrus (DG) of the hippocampus [1]. In 1965, by using thymidine-H3 tagging, Altman and Das discovered the existence of new-born granule cells in the adult rat dentate gyrus [2, 3]. Thymidine-H3 tagging was later combined with neuronal nuclear protein (NeuN), a marker of mature neurons, to confirm that neural stem cells proliferate and differentiate into mature granule neurons in the rat dentate gyrus [4], igniting interest in the functional relevance of these adult-born hippocampal neurons, and raising questions about whether adult hippocampal neurogenesis is also prevalent in humans.
By using a thymidine analogue called bromodeoxyuridine (BrdU), which incorporates into the DNA of all proliferating cells, and combining this with the neuronal markers NeuN, calbindin, and neuron specific enolase, a landmark paper in 1998 revealed evidence that adult hippocampal neurogenesis also occurs in the human dentate gyrus [1]. However, several publications in recent years have encouraged debate around the existence and importance of adult hippocampal neurogenesis in humans, and how it relates to brain disorders in humans [5–8].
Immunohistochemistry is currently the most widely used method to directly analyse hippocampal neurogenesis, as it allows for the identification and spatial understanding of specific proteins, or antigens, expressed during specific stages of the neurogenesis process. Various protein markers have been identified as being expressed at specific stages of neurogenesis such as during proliferation of neural stem cells, neuronal differentiation, maturation and survival, and integration into existing neuronal circuitry (Fig. 1) [9, 10].
Fig. 1
Common markers for various stages of hippocampal neurogenesis. Schematic representation of hippocampal neurogenesis in the mouse dentate gyrus (DG). Quiescent neural stem cells in the subgranular zone (SGZ) undergo activation yielding daughter cells that can self-renew the pool of neural stem cells or differentiate to generate neural progenitor cells and eventually neurons. Neuroblasts that survive subsequent phases of maturation will migrate into the granule cell layer (GCL) and develop into granule neurons. Finally, these cells will incorporate into hippocampal circuitry. Various commonly used markers expressed at different stages of hippocampal neurogenesis are indicated. In addition to these markers, endogenous cellular proliferation markers, such as Ki67, as well as administerable markers, such as BrdU, have become a widely used method to answer multiple questions around hippocampal neurogenesis. Upon administration via injection, BrdU incorporates into the DNA during the S-phase of the cell cycle, when cells are undergoing division [250]. Proliferation markers such as Ki67 and BrdU do not specifically label cells fated to become neurons, and thus must be used with at least one additional neuronal marker. Marker abbreviations: double cortin (DCX); glial fibrillary acidic protein (GFAP); neuronal nuclear protein (NeuN); neurogenic differentiation (NeuroD); prospero-related homeobox (Prox1); polysialylated neuronal cell adhesion molecule (PSA-NCAM); SRY-box transcription factor 2 (Sox2); homologue of the Drosophila tailless gene (TLX), also known as nuclear receptor subfamily 2 group E member 1 (NR2E1). Figure adapted from [10].
![Common markers for various stages of hippocampal neurogenesis. Schematic representation of hippocampal neurogenesis in the mouse dentate gyrus (DG). Quiescent neural stem cells in the subgranular zone (SGZ) undergo activation yielding daughter cells that can self-renew the pool of neural stem cells or differentiate to generate neural progenitor cells and eventually neurons. Neuroblasts that survive subsequent phases of maturation will migrate into the granule cell layer (GCL) and develop into granule neurons. Finally, these cells will incorporate into hippocampal circuitry. Various commonly used markers expressed at different stages of hippocampal neurogenesis are indicated. In addition to these markers, endogenous cellular proliferation markers, such as Ki67, as well as administerable markers, such as BrdU, have become a widely used method to answer multiple questions around hippocampal neurogenesis. Upon administration via injection, BrdU incorporates into the DNA during the S-phase of the cell cycle, when cells are undergoing division [250]. Proliferation markers such as Ki67 and BrdU do not specifically label cells fated to become neurons, and thus must be used with at least one additional neuronal marker. Marker abbreviations: double cortin (DCX); glial fibrillary acidic protein (GFAP); neuronal nuclear protein (NeuN); neurogenic differentiation (NeuroD); prospero-related homeobox (Prox1); polysialylated neuronal cell adhesion molecule (PSA-NCAM); SRY-box transcription factor 2 (Sox2); homologue of the Drosophila tailless gene (TLX), also known as nuclear receptor subfamily 2 group E member 1 (NR2E1). Figure adapted from [10].](https://content.iospress.com:443/media/bpl/2022/8-1/bpl-8-1-bpl220141/bpl-8-bpl220141-g001.jpg)
1.2Factors influencing adult hippocampal neurogenesis
The process of adult hippocampal neurogenesis is regulated by multiple endogenous factors. For instance, GABAergic excitation induces neuroblasts to develop into immature neurons [11]. Neurotrophic factors, such as brain-derived neurotrophic factor (BDNF), glia-derived nerve factor (GDNF), nerve growth factor (NGF) and insulin-like growth factor 1 (IGF-1) promote adult hippocampal neurogenesis [12–14]. Microglia, which are innate immune cells resident to the central nervous system, prune synapses of maturing neurons and can produce cytokines which can induce or hinder neuron development and survival [15].
Multiple exogenous factors also influence adult hippocampal neurogenesis (Fig. 2), including diet [16], stress [17–20], antidepressant treatment, exercise, and environmental stimuli [17, 21–23]. While the role of adult hippocampal neurogenesis in humans is not entirely clear, adult hippocampal neurogenesis has been shown to affect specific processes of learning and memory including spatial learning and memory [24–28], trace conditioning memory [29], pattern separation [30–32], and object recognition [33], and has been implicated in stress resilience [21, 34, 35], and antidepressant action [23, 36, 37] in rodents. While the existence of hippocampal neurogenesis during adulthood remains controversial in humans due to limitations such as tissue processing and the necessity to obtain brain tissue quickly post-mortem [38, 39], analysis of adult hippocampal neurogenesis in post-mortem human tissue has implicated adult hippocampal neurogenesis in Alzheimer’s Disease (AD) [40]. Due to the importance of adult hippocampal neurogenesis in regulating cognition, stress susceptibility, and antidepressant action, it is increasingly important to understand the factors influencing hippocampal neurogenesis (Fig. 2), and how they may potentially be utilized to improve current disease treatment options.
Fig. 2
Factors influencing adult hippocampal neurogenesis. Adult hippocampal neurogenesis is increased following exercise, antidepressant drug consumption, a healthy diet, neurogenic immune signalling, and a healthy gut microbiome. However, other factors can be detrimental to adult hippocampal neurogenesis, including aging, diets high in fat and sugar, stress, an aberrant immune system, and disruption of the gut microbiome. These factors can influence the proliferation, maturation and survival of neurons in the adult hippocampus. Figure adapted from [41].
![Factors influencing adult hippocampal neurogenesis. Adult hippocampal neurogenesis is increased following exercise, antidepressant drug consumption, a healthy diet, neurogenic immune signalling, and a healthy gut microbiome. However, other factors can be detrimental to adult hippocampal neurogenesis, including aging, diets high in fat and sugar, stress, an aberrant immune system, and disruption of the gut microbiome. These factors can influence the proliferation, maturation and survival of neurons in the adult hippocampus. Figure adapted from [41].](https://content.iospress.com:443/media/bpl/2022/8-1/bpl-8-1-bpl220141/bpl-8-bpl220141-g002.jpg)
2Factors that influence both adult hippocampal neurogenesis and the gut microbiome
Fascinatingly, several of the factors that are known to influence adult hippocampal neurogenesis demonstrate the parallel ability to influence the composition or function of the gut microbiome, the trillions of microorganisms which reside in the gastrointestinal tract (Fig. 3) [42]. The gut microbiota has been increasingly shown to manipulate cell processes and biological functions in the brain, including adult hippocampal neurogenesis, through what is known as the microbiota-gut-brain axis (Fig. 4) [43].
Fig. 3
Factors infleuncing the gut microbiota and hippocampal neurogenesis. Blue arrows indicate that the factors have been shown to influence hippocampal neurogenesis, while pink arrows indicate the factors have been shown to influence the gut microbiome. Currently, there is no evidence that birth mode (vaginal vs caesarean section), geography/culture, nor breastfeeding impact adult hippocampal neurogenesis. Some evidence indicates that early weaning decreases hippocampal neurogenesis in mice [51], although it is impossible to untangle whether this response was due to stress caused by premature maternal separation or the temporally altered change in nutrition.
![Factors infleuncing the gut microbiota and hippocampal neurogenesis. Blue arrows indicate that the factors have been shown to influence hippocampal neurogenesis, while pink arrows indicate the factors have been shown to influence the gut microbiome. Currently, there is no evidence that birth mode (vaginal vs caesarean section), geography/culture, nor breastfeeding impact adult hippocampal neurogenesis. Some evidence indicates that early weaning decreases hippocampal neurogenesis in mice [51], although it is impossible to untangle whether this response was due to stress caused by premature maternal separation or the temporally altered change in nutrition.](https://content.iospress.com:443/media/bpl/2022/8-1/bpl-8-1-bpl220141/bpl-8-bpl220141-g003.jpg)
Fig. 4
Proposed and established mechanisms of microbial regulation of adult hippocampal neurogenesis. Microbe-derived signals can be transmitted to the brain through neuronal, endocrine, metabolite-driven, and immune pathways. The vagus nerve allows for direct neuronal transmission of gut signals to the nucleus tractus solitarius in the brain, which can be relayed to the hippocampus via the medial septum. Through fecal microbiota transplant and probiotic supplementation, microbial signalling from the gut has been shown to alter levels of hippocampal BDNF and influence adult hippocampal neurogenesis. Immune cells circulating the gut, including monocytes and T cells, are sensitive to the gut microbiota, reacting to microbial cell wall components, such as lippopolysaccharide, and microbially-produced metabolites, such as short-chain fatty acids and indoles, that permeate the intestinal epithelium. These immune cells are also primed by the gut microbiota during early life, shaping their functionality in adulthood. T cells and monocytes can traffic to the brain, where they, along with resident microglia, can produce pro-inflammatory and anti-inflammatory cytokines, which have been shown to inhibit or promote hippocampal neurogenesis. Microglia are also sensitive to microbially-produced short-chain fatty acids, which can cross the intestinal epithelium and blood-brain barrier to directly modulate the functionality of microglia. In addition to cytokine production, microglia are responsible for pruning neuronal dendrites and supporting neuronal development and survival. The gut microbiota can metabolize tryptophan, a precursor to serotonin, kynurenine, and indole. Indole produced by the gut microbiota can directly bind to aryl hydrocarbon receptors (AhR) on neural progenitor cells, promoting neuronal differentiation. Immune cells are also sensitive to indoles through AhR receptors. Intestinal neurotransmitter and hormone production is mediated by enteroendocrine cells and under partial control by the gut microbiota, but whether microbial regulation of these metabolites directly influences adult hippocampal neurogenesis in vivo is unconfirmed. Studies involving germ-free mice and microbiota transplantation have demonstrated the importance of the gut microbiota for regulating the HPA axis, which contributes to the production of cortisol (or corticosterone in rodents). Glucocorticoids can directly bind to receptors on progenitor cells and immature neurons and generally have a supressive effect on hippocampal neuron proliferation and survival of newborn neurons. In addition to adult hippocampal neurogenesis, the gut microbiota contributes to neuroplasticity, long term potentiation, and intestinal barrier and blood brain barrier permiability, which offers some mechanistic insight into how the gut microbiota may regulate adult hippocampal neurogenesis and related behaviour. Abbreviations: Adrenocorticotropic hormone (ACTH); brain-derived neurotrophic factor (BDNF); orticotropin-releasing factor (CRF); enteroendocrine cell (EEC); hypothalamus-pituitary-adrenal (HPA).
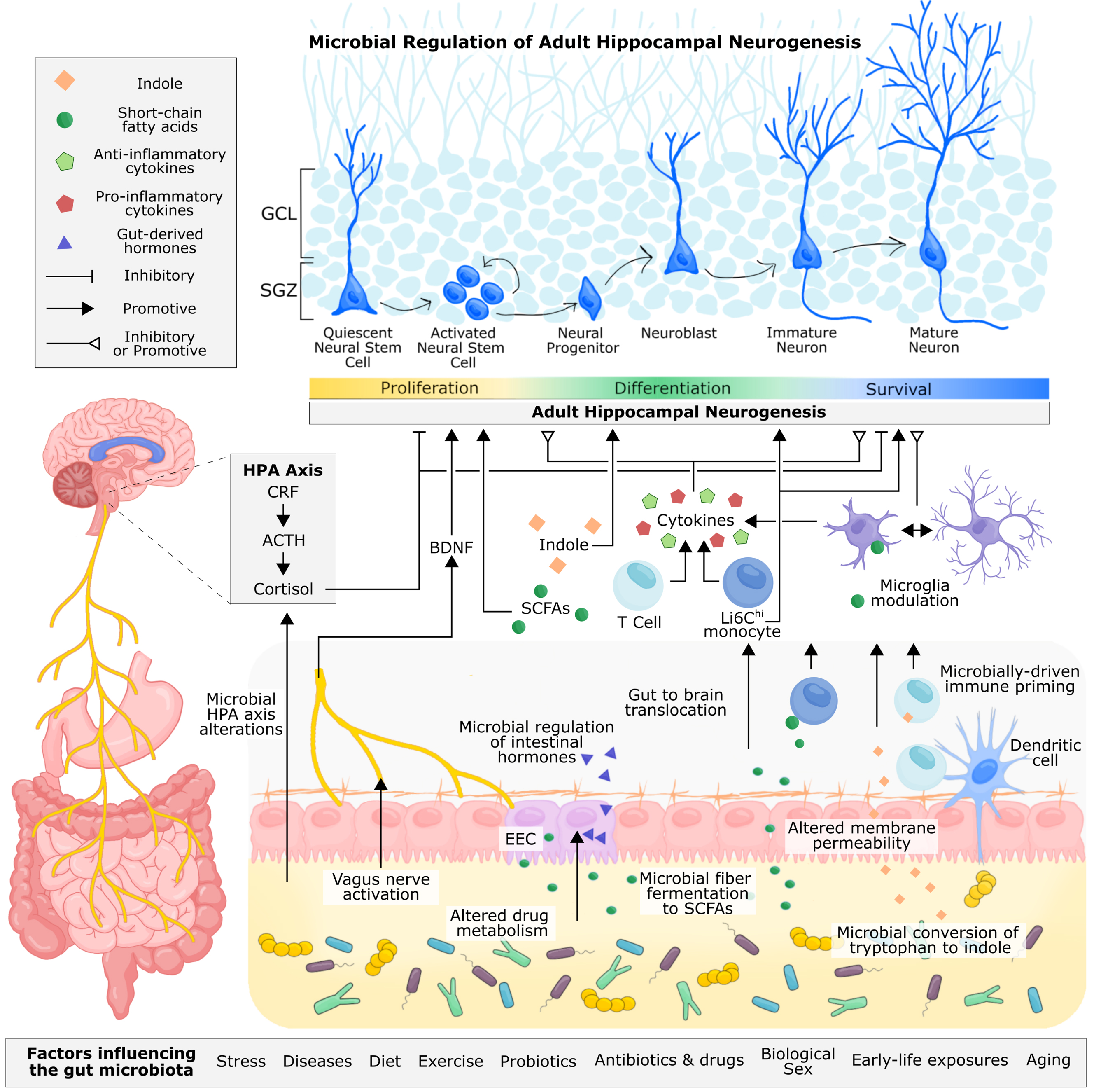
Although it is currently largely unknown whether lifestyle factors that influence adult hippocampal neurogenesis and the gut microbiome are driven by alterations to the gut microbiome or whether these effects happen independently and in parallel, increasing evidence through microbiota transplant studies [44–46], germ-free (GF) rodents completely devoid of microbes [47, 48], and microbiota-targeted interventions (such as antibiotics or dietary supplementation) [49, 50] have revealed a clear importance of the gut microbiota in hippocampal neurogenesis, as discussed later.
2.1Stress and antidepressants
Adult hippocampal neurogenesis is disrupted during stress [46, 52], and has emerged as a target of antidepressant action [52]; a hallmark of chronic antidepressant treatments is the ability to increase adult hippocampal neurogenesis [53–58], which is required for some of the behavioural effects of some antidepressants [36, 37, 59]. A direct causal link between disrupted adult hippocampal neurogenesis and depression has not yet been established in humans. Nonetheless, human post-mortem studies have reported that antidepressant-treated individuals with diagnosed depression had a higher number of neural progenitor cells in the dentate gyrus compared with those with depression that were not treated with antidepressants [57, 58]. Moreover, the serum concentration of BDNF (a protein supporting neurogenesis) is reduced in patients with depression as well as in stressed rats and is normalized following antidepressant treatment [60, 61], indicating similar pathologies between humans and model organisms.
Both stress [62, 63] and antidepressants [64] also have a noticeable impact on the gut microbiome, and the gut microbiome has also been found to contribute to the stress response in rodents [65, 66]. Stress induces volatility in the gut microbiome [63], and different types of stress have varying effects on the composition of the gut microbiome. Stress during early life appears to reduce Lactobacillus abundance in primates and infants, along with reducing Bifidobacterium in infants [67, 68]. Lactobacillus displays sensitivity to various types of stress, including acute social stress, chronic unpredictable mild stress, and restraint stress in rodents [46, 69], and interestingly, Lactobacillus rhamnosus supplementation reduced anxiety behavior and the acute stress-induced elevation in plasma corticosterone in mice [70]. Furthermore, Helicobacter pylori increased in the mouse stomach following long-term psychosocial stress [71], while people suffering from post-traumatic stress disorder appear to have a lower abundance of Actinobacteria, Lentisphaerae, and Verrucomicrobia compared with trauma-exposed individuals [72].
Moreover, the antimicrobial effects of some antidepressants suggest their action may be, at least in part, mediated by their ability to restructure the gut microbiome. For instance, serotonin reuptake inhibitor antidepressants, such as fluoxetine and sertraline, can inhibit efflux pumps on bacterial cell walls, and display antimicrobial properties against some gram-positive bacteria, including Enterococcus and Staphylococcus [73], while fluoxetine demonstrates the ability to decrease Lactobacillus rhamnosus, Escherichia coli, Lactobacillus johnsonii, and Bacteroidales S24-7 in the rodent gut [64, 74]. Moreover, several antidepressants, including fluoxetine, escitalopram, duloxetine, and desipramine, have been shown to decrease the relative abundance of Adlercreutzia and Ruminococcus in mice, while concomitant administration of Ruminococcus flavefaciens to mice treated with the antidepressant duloxetine was sufficient to abolish some antidepressant effects of duloxetine [75], suggesting that not only can antidepressants affect gut bacteria but that gut bacteria can also affect antidepressant action, though adult hippocampal neurogenesis was not assessed in this study. While this evidence is promising, there exists a gap in understanding as to whether the gut microbiota can mediate antidepressant-like behaviour through altering adult hippocampal neurogenesis. Therefore, mechanistic studies evaluating the potential of the gut microbiota to mediate adult hippocampal neurogenesis in the context of stress and depression should be pursued to explore potential, novel, microbiota-based treatment options.
Meanwhile, germ-free (GF) mice, devoid of all microbes, exhibited an exaggerated hypothalamic-pituitary-adrenal (HPA) stress response compared to specific pathogen free (SPF) mice, and expressed less BDNF in the hippocampus compared to SPF mice [65]. The colonization of GF mice with a single strain of Bifidobacterium infantis normalized their divergent stress response [65]. Furthermore, patients suffering from major depressive disorder harbour unique signatures in their gut microbiomes, including decreased microbial diversity and richness, and when their faecal microbiota was transferred to healthy, naive rats, the recipient rats displayed anxiety-like and anhedonia behaviours [66]. Similarly, transferring the microbiota from stressed mice into naive mice is sufficient to transfer biological and behavioural phenotypes induced by stress, including decreased hippocampal endocannabinoid signalling, altered serum metabolite levels, and despair behaviour [46].
2.2Environmental enrichment and exercise
Environmental enrichment, including exercise and learning tasks, increases adult hippocampal neurogenesis in healthy adult rodents [26, 76–78]. Through the promotion of hippocampal neurogenesis, environmental enrichment has been reported to promote the extinction of submissive and depressive-like phenotypes following social defeat stress and chronic exposure to an aggressor mouse, which are forms of psychosocial stressors [79]. Exercise is a form of environmental enrichment that promotes the production and survival of neurons in the adult hippocampus and has antidepressant-like behavioural effects [80, 81]. Although the mechanisms by which exercise promotes hippocampal neurogenesis are not fully established, exercise-induced release of serotonin in the central nervous system is key for the promotion of neuronal proliferation [82]. Moreover, exercise has been reported to increase serum BDNF in humans [81].
In parallel, it has been reported that exercise also impacts the gut microbiome [83, 84]. One study comparing endurance athletes to individuals with a high body mass index found that 48 taxa were increased in elite rugby players including increased Akkermansia and lower abundance of the taxon Bacteroidetes [83]. Exercise in humans has also been found to increase genera related to the production of short-chain fatty acids, including Faecalibacterium prausnitzii [85]. Moreover, the gut microbiota is able influence the trafficking of Ly6Chi monocytes, a known pathway by which exercise increases adult hippocampal neurogenesis [49, 86]. Recently, by transferring the microbes from mice exposed to environmental enrichment into standard rearing recipient mice, it was shown that the gut microbiota related to environmental enrichment can promote neuronal plasticity in the visual cortex, potentially via modulation of short-chain fatty acids [87], though whether the gut microbiota drives similar pro-plasticity occurrences in the hippocampus is currently unknown.
2.3Aging
Aging involves the gradual deterioration of homeostatic functions coinciding with an increased incidence of disease [88]. The characteristics of aging can be seen at a genetic and cellular level, resulting in immunosenescence, an imbalance of chemical messengers (including a reduction in growth hormones, disequilibrium of neurotransmitters) and disrupted hormonal and nutrient sensing [88]. This whole-body degeneration is also evident in the physiology and function of the gastrointestinal tract, with marked changes in gastric motility, declining gastrointestinal epithelial barrier integrity [89], complications in digestion (such as hypochlorhydria), and deterioration of the ENS contributing to striking alterations of the gut microbiota [90].
Hippocampal neurogenesis is substantially reduced during physiological aging in rodents, primates, and humans [4]. Aged rodents (20–24 months) have a substantial loss in dentate gyrus progenitor proliferation, leading to a reduction in the production of new-born granule cells [91, 92]. Furthermore, senescence of the hippocampal neurogenic niche reduces the proportion of neural stem cells that are actively dividing [93, 94], perhaps due to a depletion in the quantity of neural stem cells [95]. This reduction in neurogenesis is noticeable in mice as young as 6 months of age and correlates to impaired cognition in rodents [96] as well as the progression of cognitive decline in Alzheimer’s Disease in rodent models and humans [9, 40]. Rejuvenating the aged neurogenic niche through diet, exercise, or factors in the blood has been shown to rescue aging-associated declines in hippocampal neurogenesis and cognitive deficits, as reviewed extensively elsewhere [97], further implicating hippocampal neurogenic processes with age-related cognitive decline. Furthermore, lifestyle factors such as exercise and cognitive stimulation promote hippocampal neurogenesis and may be protective against aging-related neuropathologies including cognitive decline [78, 98, 99]. This evidence suggests that targeting the aging-associated decline in hippocampal neurogenesis may be an important strategy for developing novel therapeutics for treating declining cognition in aging.
In addition to declining hippocampal neurogenesis, aging triggers an increase in intestinal microbial diversity which is correlated to frailty, longevity [100–103], and other markers of health in aging [104, 105]. Studies involving centenarians have implicated the gut microbiota in longevity and extreme aging, revealing specific taxa such as Akkermansia that may contribute to healthy aging [100, 106, 107]. Interestingly, germ-free rodents, void of any microbiota, have extended lifespans [108]. Moreover, transferring gut microbiota from young to adult killifish extended the lifespan of recipient fish, while microbes from age-matched donors had no such effect [102]. The distinguishable shift in the composition and diversity of the gut microbiome during mammalian aging typically leads to an increase in gut microbiota diversity as dominant commensal taxa are lost and replaced by other commensals and pathobionts [109]. Aging commonly triggers a decrease in the genera Prevotella, Roseburia, Corpococcus, Faecalibacterium, and Bifidobacterium, the latter of which includes several probiotic strains which have been preclinically shown to increase adult hippocampal neurogenesis, hippocampal BDNF expression, and convey anxiolytic effects to their host following stress [110–112]. Several other taxa begin to emerge in the aging gut and have been grouped into two categories; those associated with healthy aging (such as Akkermansia, Odoribacter, and Christensenellaceae) and pathobionts associated with worsened health in aging (such as Bilophila, Desulgovibrio, Fusobacterium, and Enterovacteriaceae) [109]. Moreover, the retention of abundance of Bacteroides or low microbial uniqueness correlated with increased mortality in elderly people [113]. Notably, the aged gut microbiota has been shown to promote systemic inflammation and immunosenescence, leading to T-cell activation and disrupting intestinal barrier integrity [114]. The capability for aging-associated gut microbiota to perturb adult hippocampal neurogenesis is discussed in section 3.1.3.
2.4Diet
Preclinical dietary manipulations have revealed the importance of diet in promoting and maintaining adult hippocampal neurogenesis. Diets high in fat and sugar decrease hippocampal cell proliferation, the survival of newly born neurons, and hippocampal-dependent behaviour in mice and rats [50, 115, 116], potentially by influencing neuronal mitochondria biogenesis [50] and/or the increase in circulating corticosterone [116]. Meanwhile, long-term running exercise was able to counteract the blunting consequences of a high fat diet on the survival of newly born hippocampal neurons and promote spatial learning and reversal learning performance in the Morris water maze task in mice [117]. On the other hand, dietary patterns which can influence the gut microbiome, including caloric restriction and intermittent fasting, promote longevity and improve memory [118–127] potentially through the upregulation of brain-derived neurotrophic factor [128, 129].
Some dietary compounds have been deemed prebiotics since they are selectively utilized by the gut microbiome, such as fermentable fibres and phenolics [130]. Diets rich in prebiotics appear to ameliorate aging-related cognitive and immune deficits [131, 132] and have also been shown to alter hippocampal neurogenesis. For instance, higher consumption of certain prebiotic polyphenols can promote hippocampal neuron proliferation and survival [133–135]. Dietary supplementation with specific bacteria can directly alter adult hippocampal neurogenesis [46, 49, 110, 136, 137], as discussed in section 3.1.4.
3The gut microbiota regulates adult hippocampal neurogenesis
The parallel between factors that influence both adult hippocampal neurogenesis and the gut microbiome make it less surprising that the gut microbiota can orchestrate alterations to neurogenesis in the hippocampus. While the factors outlined above could exert independent effects on gut microbiota and hippocampal neurogenesis, there is emerging evidence that the gut microbiota can regulate adult hippocampal neurogenesis. Manipulations of the gut microbiota, including germ-free rodents, antibiotics, microbiota transfers, and prebiotic dietary interventions, as well as studies interrogating potential mechanisms of action through the vagus nerve, immune system, and metabolite-driven routes, have laid solid preclinical evidence that there are multiple pathways by which the gut microbiota can regulate adult hippocampal neurogenesis, summarized in Fig. 4.
3.1Evidence from gut microbiota manipulation studies
3.1.1Germ-free rodents
As discussed previously, GF animals are completely sterile from all microorganisms, and therefore act as a clean slate for understanding how the microbiota shapes host biology. In addition to having distinctly aberrant immune profiles, gut abnormalities, and behavioural abnormalities including hyperactivity, cognitive deficits, and altered anxiety-like and depressive-like behaviour, GF rodents have unique disruptions within the brain, culminating in impaired blood-brain barrier function, immature microglia, increased myelination, and altered hippocampal neurogenesis [138]. In 2015, Ogbonnaya and colleagues found that adult GF mice had increased numbers of BrdU+/NeuN+ cells in the hippocampus [47]. Moreover, colonizing 3-week-old GF Swiss Webster mice with microbiota was unable to rescue these effects [47]. Others have since observed alterations in hippocampal neurogenesis in GF mice seems to be sex-, age-, and potentially strain-sensitive. Specifically, male C57BL/6J GF mice were shown to have fewer DCX+ maturing neurons in their hippocampus compared to conventional C57BL/6J mice at 4 weeks old –a transient effect which was not apparent at 8 or 12 weeks of age in this study [48], though the reduction in DCX+ cells in the hippocampus of adult male C57BL/6J GF mice compared to SPF mice was still significantly apparent in another study [139]. Meanwhile, the density of maturing neurons in 4-week-old female C57BL/6J mice did not significantly diverge from their conventional counterparts, and instead had a greater density of hippocampal DCX+ cells at 8 weeks of age, but not at 12 weeks of age [48]. Similar findings were observed in the proliferation and death of cells in the dentate gyrus [48]. Moreover, hippocampal serotonergic signalling [140] and serotonin biosynthesis [141] are disrupted in GF rodents, both of which are important regulators of hippocampal neurogenesis.
3.1.2Antibiotics
While commonly administered to treat infections clinically, oral antibiotics also act as a useful tool for perturbing the gut microbiota and can therefore be useful for understanding the relationship between the gut microbiota and hippocampal neurogenesis. In mice, an orally administered cocktail of antibiotics consisting of ampicillin, vancomycin, ciprofloxacin, imipenem, and metronidazole for 7 weeks decreased hippocampal cell proliferation (BrdU), neuron maturation (BrdU/DCX/NeuN) and neuron survival (BrdU/NeuN), and also impaired novel object recognition [49]. Interestingly, this effect was prevented when mice were allowed free access to a running wheel or administered a faecal transplant from health SPF mice [49]. Moreover, through adoptive transfer or elimination of Ly6Chi monocytes, Möhle and colleagues demonstrated that gut microbiota signaling to trafficking Ly6Chi monocytes drive alterations to adult hippocampal neurogenesis [49]. Antibiotic use has been clinically associated with neurotoxicity in at-risk patients, including elderly and juvenile individuals [142], and chronic antibiotic use in middle-aged women was associated with decreased cognitive ability after a roughly 7-year follow-up [143]. Whether these clinical effects are mediated by the gut microbiota or are rather off-target direct consequences of antibiotics has not been confirmed.
3.1.3Faecal microbiota transplant
Faecal microbiota transplant (FMT) involves the transfer of an entire microbiota from one organism into another organism and is therefore a valuable technique for interrogating how specific naturally existing microbial communities influence their host. FMT has been popularized medically for its 90% success rate in treating recurrent Clostridioides difficile infection, a life-threatening infection which is often resistant to existing antibiotics [144], and is now being trialled for other gastrointestinal diseases as well as neurological disorders, including depression [145].
In 2016, FMT from depressed patients into naïve rats was shown to induce anxiety-like and depressive-like phenotypes in the recipient rats, a phenomenon which did not occur when rats were given FMT from healthy people, however adult hippocampal neurogenesis was not investigated in this study [66]. Since then, FMT from depressed patients, as well as chronically stressed mice, has been shown to decrease the quantity of DCX+ immature neurons in the dentate gyrus of recipient naïve mice and to also induce depressive-like behaviour [46, 137], confirming that the gut microbiota is an active regulator of hippocampal neurogenesis, and also indicating that the gut microbiota may play an active role in the progression of diseases in which disrupted hippocampal neurogenesis has been implicated.
In addition to stress-related conditions, the gut microbiota has also been shown to play a causative role in the deficit in cognitive ability that occurs with aging. For instance, transplanting microbiota from aged mice into young mice impaired spatial memory and learning ability and altered synaptic plasticity-related protein expression [146]. Counterintuitively, despite the decreased hippocampal neurogenesis occurring in aged mice, when microbiota from aged mice where transplanted into young germ-free mice, the hippocampus of young recipient mice had increased DCX+ immature neurons in their dentate gyrus compared to their counterparts which received microbiota from young mice [44]. Meanwhile, FMT from 18-month old C57BL/6 mice into old germ-free mice did not induce differences in the density of hippocampal DCX+ cells [44], which indicates that, while the gut microbiota can confer alterations to hippocampal neurogenesis, the innate biology of the recipient is also critical for achieving specific microbiota-driven effects. Separately, adult hippocampal neurogenesis and memory function were impaired in 8-week-old male C57Bl/6 mice who were administered FMT from 9-month old 5xFAD mice (a mouse model of Alzheimer’s disease), but not from aged-matched C57BL/6 mice [147], potentially implicating the gut microbiome in the pathology of Alzheimer’s disease. There appears to be limitations for the ability of the gut microbiota to restore the aging-related reduction in hippocampal neurogenesis; a study investigating the impacts of faecal microbiota transfer from young mice to aged mice on the aging brain did not observe improvements in the survival of newly born hippocampal neurons, although some aspects of neurogenesis-linked spatial learning and memory were rescued following this microbiota transfer [148]. In addition to aging and stress related disruptions to hippocampal neurogenesis, FMT has also been utilized to demonstrate that the drug-induced restructuring of the gut microbiota also reshapes its ability to alter hippocampal neurogenesis. Mice that consumed a high-fat diet and also administered metformin, a drug commonly prescribed to treat diabetes, did not suffer from high-fat diet-induced deficits to hippocampal neurogenesis and cognitive ability. Moreover, when microbiota from these metformin-treated mice were transferred into mice currently on a high-fat diet but otherwise drug-naïve, FMT was sufficient to increase the number of proliferating cells in the hippocampus and improve novel object recognition [149].
3.1.4Individual bacteria and consortia administration
While FMT is a valuable tool for understanding how complex communities of microorganisms impact their host, it does not allow for the granularity in deciphering whether specific microbes within the community are driving the observed effects. Moreover, FMT is an invasive procedure when conducted clinically, and carries a risk for transferring pathogenic bacteria. Therefore, administration of single bacterial strains or consortia of bacteria are useful approaches to tease out mechanistic details of how the gut microbiota impacts adult hippocampal neurogenesis. The discovery of individual probiotic strains or consortia of bacteria has historically been driven by correlative evidence showing a decreased relative abundance of specific bacteria in diseased populations and remains largely explorative. Nonetheless, through correlative approaches, a handful of bacteria have been shown to regulate hippocampal neurogenesis. For instance, administration of Lactobacilli plantarumWJL to chronically stressed mice for 5 weeks was sufficient to increase hippocampal cell proliferation and the survival of newly born neurons in the dentate gyrus [46]. Moreover, a consortium consisting of eight bacterial strains, including Lactobacillus acidophilus, Lactobacillus plantarum, Lactobacillus paracasei, Lactobacillus delbrueckii subsp. Bulgaricus and also Streptococcus thermophilus, Bifidobacterium breve, Bifidobacterium longum, Bifidobacterium infantis increased hippocampal neurogenesis in mice subjected to unpredictable chronic mild stress, an effect which FMT from SPF mice was unable to achieve [49]. A separate multi-strain mixture of bacteria, consisting primarily of Bifidobacterium animalis subsp. lactis and Streptococcus thermophilus along with other Lactobacillus and Lactiplantibacillus strains increased the number of DCX+ cells in the dentate gyrus and mitigated neuroinflammatory responses in mice injected with lipopolysaccharide, a component of the outer membrane of some bacteria [136]. Moreover, 2-week consumption of a dual-strain probiotic consisting of L. helveticus R0052 and B. longum R0175 increased the number of DCX+ cells in the dentate gyrus of male C57Bl/6 mice that had previously been exposed to water avoidance stress [110], and has clinically been shown to alleviate the severity of depression [150, 151].
While some bacteria appear to harness the ability to increase adult hippocampal neurogenesis, other strains have been shown to disrupt it. For instance, one study found that administration of Bacteroides uniformis or Bacteroides fragilis to naïve, antibiotic pre-treated mice reduced the mean number of DCX+ cells in the dentate gyrus compared to naïve, antibiotic pre-treated mice that received pasteurized Bacteroidetes [137].
3.1.5Prebiotics and diet
Diets including various fruits and vegetables, which are high in prebiotic polyphenols [152], have been clinically associated with improvements in depressive symptomology [153], and yields antidepressant and anti-anxiolytic effects in various rodent models [154–156]. Meanwhile, a Mediterranean-style diet, which is high in fish oils, was sufficient to improve mental health scores in individuals with depression [157] and improve cognitive performance in aging humans [158]. Omega-3 fatty acids, which are prevalent in high-fish diets, have been shown to promote hippocampal neurogenesis in mice [159] and lobsters [160]. Separately, omega-3 fatty acids were able to act directly on cultured human hippocampal progenitor cells, therein preventing cortisol induced reductions in neurogenesis [161]. Nonetheless, the mechanisms of action for how specific diets and dietary compounds, such as polyphenols and fibres, exert their effects on the brain remains unknown, though their ability to influence the gut microbiota may allow for their potential effects on hippocampal neuroplasticity-related behaviours [132, 162, 163].
As defined earlier, prebiotics are substrates selectively utilized by the gut microbiome, such as fermentable fibres and phenolics [130]. Fermentable fibres can be digested by bacteria into short-chain fatty acids including acetate, butyrate, and propionate, which have immunomodulatory and histone deacetylase inhibitory properties, including directly in the brain [164, 165]. When administered orally to pigs for 4 weeks, sodium butyrate increased hippocampal neurogenesis and hippocampal granular cell layer volume [166]. Oral sodium butyrate has also been shown to ameliorate cognitive deficits and increase the expression of neurotrophic factors in rats subjected to early life stress [167], and decrease hippocampal neuroinflammation in aging mice [168]. The soluble dietary fibres fructo-oligosaccharides (FOS) and galacto-oligosaccharides (GOS) have been shown to regulate BDNF and synaptic protein expression in rodents [169, 170], though whether they can directly alter hippocampal neurogenesis has not yet been demonstrated.
In addition to fibre, various flavonols exert neurotrophic effects, although it is largely unclear whether these effects require the gut microbiome or simply restructure the gut microbiota independently from their effects in the hippocampus. For example, the polyphenol quercetin can enhance learning and memory, alleviate anxiety-like behaviour, increase neural stem cell and progenitor cell proliferation, promote neurotrophic factors including BDNF, and increase the quantity of DCX+ dentate gyrus cells in rats [162, 171]. Meanwhile, a citrus flavonoid, 3,5,6,7,8,3′,4′-Heptamethoxyflavone, increases adult hippocampal neurogenesis in mice that were exposed to chronic unpredictable mild stress [172]. Moreover, the peel of the Citrus kawachiensis fruit, which contains 3,5,6,7,8,3′,4′-Heptamethoxyflavone and other bioactive compounds prevented hippocampal microglia activation and ameliorated deficits in hippocampal neurogenesis in senescence-accelerated mouse-prone 8 (SAMP8) mice, a model of accelerated aging [173]. Another flavonoid, spinosin, exhibited a dose-dependent increase in dentate gyrus DCX+ neurons, as well as increased cell proliferation, survival, and improved cognitive performance in the passive avoidance test [174]. Meanwhile, the flavonoid oroxylin A increased the survival of newly generated hippocampal neurons in adult mice [175], although these effects may occur directly, rather than through the gut microbiome [176].
3.2Potential mechanisms by which the gut microbiota influences adult hippocampal neurogenesis
There are several proposed mechanisms by which the gut microbiota may exert influence on adult hippocampal neurogenesis, including immune, neuronal, endocrine, and metabolite-driven pathways (Fig. 4).
3.2.1Immune system and neuroinflammation
The gut microbiota regulates several aspects of the host immune system, including in the brain, potentially allowing the gut microbiota to influence hippocampal neurogenesis via immune signaling pathways. For instance, germ-free mice have defective hippocampal microglia, and a higher density of microglia overall [177]. The regulation of microglia by the gut microbiota appears to be at least partially controlled by microbially produced acetate, which can translocate from the gut to influence the maturity and function of microglia in the brain [165]. Microglia contribute to the balance of neuronal cell birth and death in the subgranular zone through apoptosis-coupled phagocytosis [178], allowing them to clear debris from destroyed cells. In response to phagocytosis, the microglia secretome is altered towards substrates that have been shown to support new neuron proliferation, such as VEGF and FGF2 [178, 179]. Furthermore, upon activation, microglia produce various cytokines to induce an immune response to dangerous threats. Neuroinflammation, mediated by proinflammatory cytokines including interleukin (IL)-1β, tumour necrosis factor α (TNFα), and IL-6, has detrimental consequences to the proliferation and development of newly born hippocampal neuronal cells [180–183], and immune responses, including cytokine release, are blunted in GF mice [184]. By regulating the function and maturity of hippocampal microglia, the gut microbiota may be able to reshape hippocampal neurogenesis, although this direct mechanistic link is yet to be established.
In addition to impacting the function of microglia, the gut microbiota contributes to the systemic priming of immune cells that may traffic to the brain. Through the use of genetic knockout mice and antibody depletion, circulating Ly6Chi monocytes were shown to play a pivotal role in promoting hippocampal neurogenesis [49]. Ly6Chi monocytes traffic throughout the body and are highly sensitive to gut microbiota-derived signals. Following antibiotic treatment, mice had lower numbers of Ly6Chi monocytes in the brain, blood, and bone marrow, and displayed deficits in hippocampal neurogenesis and behaviour [49]. Adaptive transfer of Ly6Chi monocytes from the bone marrow of C57BL/6 mice to antibiotic-treated mice was able to restore the proliferation and survival of newly born neurons in the dentate gyrus [49]. Fascinatingly, supplementation with an 8-strain probiotic cocktail was also able to rescue antibiotic-induced deficits in hippocampal neurogenesis and Ly6Chi monocyte levels, providing further evidence that the gut microbiota can regulate hippocampal neurogenesis through Ly6Chi monocytes [49].
The gut microbiome has been shown to modulate the function of various types of T cells, including CD8+ T cells [185, 186], CD4+ T cells [187, 188], and Th17 cells [189, 190], suggesting potential T cell-dependent mechanisms by which the gut microbiota might influence hippocampal neurogenesis. T cells are essential regulators of hippocampal neurogenesis [191]. For instance, CD8+ T cells are required for the beneficial effects of environmental enrichment on hippocampal neurogenesis, synaptic plasticity, and behaviour including anxiety-like behaviour and spatial learning in mice [192]. Meanwhile, depletion of CD4+ T lymphocytes significantly reduced hippocampal neurogenesis, while neuronal precursor cell proliferation was increased in Rag2-/- mice, mice which produce no mature T cells or B cells, following repopulation of CD4+ cells [193]. Another subset of T cells, T helper 17 (Th17) cells, can also produce IL-17 [194], which inhibits adult hippocampal neurogenesis in mice [195], and can promote susceptibility to depressive-like behaviour in mice [196].
By microbial crosstalk with microglia, monocytes, and T cells, the gut microbiota demonstrates the ability to influence hippocampal neurogenesis in adult rodents. More research needs to be conducted however to fully understand the cellular pathways and scope of capability of microbiota-immune signalling on influencing hippocampal neurogenesis.
3.2.2Vagus nerve signalling
The vagus nerve innervates the gastrointestinal tract, allowing for bidirectional communication between the gut and the brain. Through virus-based tracing techniques, it was shown that vagal signals derived from the gut are relayed from the medial nucleus tractus solitarius through the medial septum and to dorsal hippocampal glutamatergic neurons, allowing gut-derived signals transmitted through the vagus nerve to control hippocampus-dependent episodic and spatial memory, and reduced the expression of neurogenic and neurotrophic markers (DCX and BDNF) in the dorsal hippocampus [197] though whether these signals were derived specifically from microbiota-vagus nerve interactions was not determined. Nonetheless, specific gut bacteria, such as Lactobacillus rhamnosus, demonstrate the ability to alter depressive-like behaviour, hippocampal microglia density, and specific hippocampal protein expression through the vagus nerve [70, 198]. The capacity for oral Lactobacillus rhamnosus JB1 treatment to alleviate the impact of stress on behaviour may be related to its ability to decrease the expression of the hippocampal GABAB1b gene [70], a receptor subunit that plays a crucial role in mediating stress resilience and the production of new hippocampal neurons [199]. Moreover, it was recently demonstrated that the vagus nerve is integral for the decreased hippocampal neurogenesis and hippocampus-dependent memory impairment caused by FMT from aged mice or humans into young mice [45]. However, the mechanisms by which bacteria can influence hippocampal neurogenesis directly through vagal pathways remains unclear.
Independent from the gut microbiota, stimulation of the vagus nerve has been reported to trigger increased progenitor cell proliferation, numbers of maturing neurons, and increased protein expression of the neurotrophic factor BDNF in the hippocampus of rodents [200–202]. Chronic simulation of the vagus nerve also promotes dendritic branching on immature hippocampal neurons [200]. Meanwhile, severing the vagus nerve via subdiaphragmatic vagotomy was sufficient to reduce hippocampal cell proliferation, neuronal differentiation, and BDNF mRNA expression in the adult hippocampus [197, 203].
3.2.3Microbial Metabolites
Due to complexities in tracing the origins of metabolites within a living organism, it is difficult to decipher whether specific gut microbiota-derived molecules can translocate from the gut to the brain, where they have the potential to directly hinder or support neuronal birth, maturation, and survival. As such, few radioactive tracing studies exist that examine gut microbiota-derived metabolites within the brain. Recently, one study utilizing radioactive labelling revealed that the microbe-derived short chain fatty acid acetate translocated from the gut to the brain of mice, where it influenced hippocampal microglia metabolism and function [165]. While it is possible to speculate that alteration in the functionality of microglia would be able to alter hippocampal neurogenesis, this study did not examine hippocampal neurogenesis. Nonetheless, short chain fatty acids, including acetate, butyrate, and propionate, have been shown to directly promote neuronal differentiation in neural stem cells in vitro when applied in physiologically relevant levels [50, 204], and the oral administration of sodium butyrate increased proliferating cells, DCX+ neurons and granular cell layer volume in the hippocampus of pigs [166]. Furthermore, short chain fatty acid supplementation improved performance or prevented impairments in spatial learning and memory tasks, and increased BDNF protein expression in preclinical neurodegenerative disease models [205–207], although hippocampal neurogenesis was not specifically assessed.
As discussed extensively elsewhere [208], the gut microbiota can influence the bioavailability of hormones and neurotransmitters including within the tryptophan-kynurenine- serotonin pathways by synthesizing or degrading components of this pathway. These hormones have been strongly implicated in depression [208]. Of interest, tryptophan and its metabolites are able to be modulated by the gut microbiota, and can impact host cognitive function and mood [209], as well as hippocampal neurogenesis [139]. Recent research has found that indoles that are produced by the gut microbiota-driven metabolism of tryptophan can modulate hippocampal neuroplasticity and promote the neuronal differentiation of hippocampal neural progenitor cells through the activation of the aryl hydrocarbon receptor [139]. The gut microbiota can also regulate the production of serotonin in the gut [210]. Serotonin is integral for neuronal differentiation, neurogenesis, and the in vitro proliferation and survival of neurospheres derived from adult neural stem cells [211–213]. Germ-free mice have increased hippocampal serotonin [140] however whether gut-derived serotonin can translocate to the brain to in turn promote hippocampal neurogenesis is currently unknown.
3.2.4The hypothalamic-pituitary-adrenal (HPA) axis and hormonal signalling
The hypothalamic-pituitary-adrenal (HPA) axis is a key endocrine pathway regulating the physiological response to stress. HPA axis activation triggers the release of glucocorticoids (including cortisol, or corticosterone in rodents) which can directly bind to receptors on neural stem and progenitor cells and hinder their proliferation and differentiation in vitro and in rodent models [214–216]. While a direct link between specific microbes directly regulating adult hippocampal neurogenesis through the HPA axis has not yet been elucidated, the gut microbiota can influence HPA axis activation. For instance, the HPA axis of male germ-free mice is hyperactive in response to restraint stress, resulting in increased corticosterone relative to SPF mice [65, 140], though whether this results in altered hippocampal neurogenesis post-stress is unknown. It may be plausible that the gut microbiota can adjust adult hippocampal neurogenesis through the HPA axis.
Several peptide hormones that regulate appetite and food intake also demonstrate the ability to influence adult hippocampal neurogenesis such as ghrelin [217–220], leptin [221–224], cholecystokinin [225–227], neuropeptide Y [227, 228], insulin-like growth factor 1 [229–232], gastric inhibitory polypeptide [233], and orexin-A [234]. Moreover, the gut microbiome has been linked to the regulation of several of these hormones including ghrelin [235, 236], leptin [236, 237], cholecystokinin [236, 238, 239], neuropeptide Y [237], although the mechanisms underlying these relationships remain largely elusive [240]. By targeting some of these molecules, there have been improvements in aging-associated impairments in hippocampal neurogenesis. For instance, by restoring insulin-like growth factor 1 levels in mice, researchers rescued the age-related decline in adult hippocampal neurogenesis [241, 242]. Mechanistic research needs to be conducted to better understand the involvement of the gut microbiota in regulating adult hippocampal neurogenesis through hormone-driven pathways.
4Conclusions and Future Perspectives
In this review, we aimed to provide an overview of the current literature investigating the gut microbiota and adult hippocampal neurogenesis, including the impacts of specific microbes and mechanisms by which the gut microbiota has been shown to influence the proliferation, development, and survival of new-born neurons in the adult hippocampus. We first discussed overlaps in the factors that are known to influence adult hippocampal neurogenesis and the gut microbiome, postulating that these effects might be occurring independently and unrelatedly to one another, but that they also might be linked through microbiota-driven alterations to gut-brain dialogue. Nevertheless, there is a growing body of evidence that suggests that the gut microbiota regulates adult hippocampal neurogenesis. Therefore, we then provided the current state of evidence demonstrating that the gut microbiota plays an active role in shaping neurogenesis in the adult dentate gyrus. In doing so, we have outlined influential factors and biological pathways underlying the regulation of adult hippocampal neurogenesis by the gut microbiota.
The gut microbiota harbours the ability to utilize a variety of pathways to promote or hinder adult hippocampal neurogenesis, including direct communication through the nervous system, or indirect communications via microbial metabolites, the immune system, and hormones. Still, some potential avenues of the microbiota-gut-brain axis have yet to be investigated in the context of hippocampal neurogenesis. For instance, the gut microbiota contributes to the secretion of hormones by intestinal cells, such as ghrelin [240]. Meanwhile, intraperitoneal injection of ghrelin increases BrdU+/DCX+ cells in the dentate gyrus [217]. However, whether the microbial control of adult hippocampal neurogenesis is mediated by ghrelin, or other intestinal peptides, has not yet been demonstrated and should be explored. Moreover, it is currently unclear how an individual’s mode of birth and weaning timeframe impact hippocampal neurogenesis. Mouse pups born by caesarean section demonstrated heightened anxiety-like behaviour and altered neurochemistry that persists into adulthood –phenotypes which appear reversible by targeting the early life gut microbiota [243]. Moreover, the weaning timeframe is highly critical for gut microbiota-mediated early life immune system development, and substantially impacts regulatory T cells [244]. Therefore, it is plausible that early life events, including caesarean section birth and altered weaning timeframes may impact adult hippocampal neurogenesis in a microbiota-dependent manner.
Alterations to the gut microbiota are present in numerous brain disorders, including depression [66], Alzheimer’s Disease [245], and epilepsy [246], conditions in which adult hippocampal neurogenesis has been observed to be disrupted clinically or in relevant animal models [10, 246, 247]. Given the strong preclinical evidence demonstrating the ability of the gut microbiota to influence various aspects of adult hippocampal neurogenesis, the gut microbiota may be a viable therapeutic target for treating and preventing these diseases through its ability to alter adult hippocampal neurogenesis. The gut microbiota is highly accessible to interventions, including via probiotics, antibiotics, and lifestyle choices such as diet, which presents multiple opportunities to remediate abnormal hippocampal neurogenesis which may contribute to some brain disorders.
Currently, clinical conclusions about the mechanistic ability of the gut microbiota to influence adult hippocampal neurogenesis are highly limited by the technical challenges of assessing neurogenesis in living humans. Therefore, evidence demonstrating the role of the gut microbiota in modulating adult hippocampal neurogenesis, as well as its impact on behaviour and disease progression, is largely dependent on preclinical interrogations. While there are vast biological similarities between mouse and humans, evidence collected in rodent models might not fully reflect clinical biological scenarios. Importantly, the murine and human gut microbiomes are distinctly unique, and, although biological functionality appears relatively conserved between these species, differences in anatomy and physiology between mice and humans may mean that preclinically established mechanisms might not be capable of translating to humans [248, 249]. Therefore, it is difficult to confirm whether the gut microbiota utilizes the same pathways observed in preclinical studies to impact human adult hippocampal neurogenesis. Nonetheless, largescale clinical trials involving microbiota interventions will be incredibly useful for understanding how the gut microbiota may sculpt favourable effects on the brain and related behaviour and identifying clinical biomarkers of microbiota-driven health benefits to potentially reveal novel therapeutic options. Ultimately, understanding the pathways by which the gut microbiota influence hippocampal neurogenesis may allow for the development of novel therapeutics to ameliorate hippocampal neurogenesis dysfunction, which could provide crucial treatment options for improving cognition and mood in brain disorders.
ACKNOWLEDGMENTS
The authors have no acknowledgments to report.
Funding
KEG was supported by an Irish Research Council (IRC) Government of Ireland Postgraduate Scholarship (GOIPG/2019/3198). JFC is funded by Science Foundation Ireland SFI/12/RC/2273_P2, the Saks Kavanaugh Foundation, EU H2020 project DLV-848228 DIS-COvERIE, and Swiss National Science Foundation project CRSII5_186346/NMS2068. OFO is funded by Science Foundation Ireland 20/FFP-P/8758, the Biostime Institute Nutrition and Care (BINC) –Geneva Funding Programs, and is a Funded Investigator at APC Microbiome Ireland, a research Institute funded by Science Foundation Ireland (SFI/12/RC/2273_P2).
CONFLICTS OF INTEREST
JFC has received research funding from 4D Pharma, Cremo, Dupont, Mead Johnson, Nutricia, and Pharmavite; has been an invited speaker at meetings organized by Alimentary Health, Alkermes, Ordesa, and Yakult; and has served as a consultant for Alkermes and Nestle. This support neither influenced not constrained the contents of this article. OFO has received funding for unrelated contract research from Alkermes plc. and has received renumeration as an invited speaker at a meeting organised by Janssen OFO is currently conducting unrelated collaborative research with Marigot Ltd. This support neither influenced not constrained the contents of this article. KEG declares no conflicts of interest.
REFERENCES
[1] | Eriksson PS , Perfilieva E , Bjork-Eriksson T , Alborn AM , Nordborg C , Peterson DA ,et al., Neurogenesis in the adult human hippocampus, Nat Med (1998) ;4: (11):1313–7. |
[2] | Altman J , Das GD , Autoradiographic and histological evidence of postnatal hippocampal neurogenesis in rats, J Comp Neurol (1965) ;124: (3):319–35. |
[3] | Cameron HA , Woolley CS , McEwen BS , Gould E , Differentiation of newly born neurons and glia in the dentate gyrus of the adult rat, Neuroscience (1993) ;56: (2):337–44. |
[4] | Kuhn HG , Dickinson-Anson H , Gage FH , Neurogenesis in the dentate gyrus of the adult rat: age-related decrease of neuronal progenitor proliferation, J Neurosci (1996) ;16: (6):2027–33. |
[5] | Sorrells SF , Paredes MF , Cebrian-Silla A , Sandoval K , Qi D , Kelley KW , et al., Human hippocampal neurogenesis drops sharply in children to undetectable levels in adults, Nature, (2018) ;555: (7696):377–81. |
[6] | Tobin MK , Musaraca K , Disouky A , Shetti A , Bheri A , Honer WG , et al., Human Hippocampal Neurogenesis Persists in Aged Adults and Alzheimer’s Disease Patients, Cell Stem Cell (2019) ;24: (6):974–82.e3. |
[7] | Boldrini M , Fulmore CA , Tartt AN , Simeon LR , Pavlova I , Poposka V , et al., Human Hippocampal Neurogenesis Persists throughout Aging, Cell Stem Cell (2018) ;22: (4):589–99.e5. |
[8] | Spalding KL , Bergmann O , Alkass K , Bernard S , Salehpour M , Huttner HB , et al., Dynamics of hippocampal neurogenesis in adult humans, Cell (2013) ;153: (6):1219–27. |
[9] | Kozareva DA , Cryan JF , Nolan YM , Born this way: Hippocampal neurogenesis across the lifespan, Aging Cell (2019) ;18: (5):e13007. |
[10] | Babcock KR , Page JS , Fallon JR , Webb AE , Adult Hippocampal Neurogenesis in Aging and Alzheimer’s Disease, Stem Cell Reports (2021) ;16: (4):681–93. |
[11] | Tozuka Y , Fukuda S , Namba T , Seki T , Hisatsune T , GABAergic excitation promotes neuronal differentiation in adult hippocampal progenitor cells, Neuron (2005) ;47: (6):803–15. |
[12] | Vicario-Abejon C , Johe KK , Hazel TG , Collazo D , McKay RD , Functions of basic fibroblast growth factor and neurotrophins in the differentiation of hippocampal neurons, Neuron (1995) ;15: (1):105–14. |
[13] | Choi SH , Li Y , Parada LF , Sisodia SS , Regulation of hippocampal progenitor cell survival, proliferation and dendritic development by BDNF, Mol Neurodegener (2009) ;4: :52. |
[14] | Shohayeb B , Diab M , Ahmed M , Ng DCH , Factors that influence adult neurogenesis as potential therapy, Transl Neurodegener (2018) ;7: :4. |
[15] | Chintamen S , Imessadouene F , Kernie SG , Immune Regulation of Adult Neurogenic Niches in Health and Disease, Front Cell Neurosci (2020) ;14: :571071. |
[16] | Stangl D , Thuret S , Impact of diet on adult hippocampal neurogenesis, Genes Nutr (2009) ;4: (4):271–82. |
[17] | Mirescu C , Gould E , Stress and adult neurogenesis, Hippocampus (2006) ;16: (3):233–8. |
[18] | Gould E , Tanapat P , Stress and hippocampal neurogenesis, Biol Psychiatry (1999) ;46: (11):1472–9. |
[19] | Naninck EF , Hoeijmakers L , Kakava-Georgiadou N , Meesters A , Lazic SE , Lucassen PJ , et al., Chronic early life stress alters developmental and adult neurogenesis and impairs cognitive function in mice, Hippocampus (2015) ;25: (3):309–28. |
[20] | Tanapat P , Galea LA , Gould E , Stress inhibits the proliferation of granule cell precursors in the developing dentate gyrus, Int J Dev Neurosci (1998) ;16: (3-4):235–9. |
[21] | Levone BR , Cryan JF , O’Leary OF , Role of adult hippocampal neurogenesis in stress resilience, Neurobiol Stress (2015) ;1: :147–55. |
[22] | Duman RS , Nakagawa S , Malberg J , Regulation of adult neurogenesis by antidepressant treatment, Neuropsychopharmacology (2001) ;25: (6):836–44. |
[23] | Surget A , Tanti A , Leonardo ED , Laugeray A , Rainer Q , Touma C , et al., Antidepressants recruit new neurons to improve stress response regulation, Mol Psychiatry (2011) ;16: (12):1177–88. |
[24] | Epp JR , Scott NA , Galea LA , Strain differences in neurogenesis and activation of new neurons in the dentate gyrus in response to spatial learning, Neuroscience (2011) ;172: :342–54. |
[25] | Epp JR , Spritzer MD , Galea LA , Hippocampus-dependent learning promotes survival of new neurons in the dentate gyrus at a specific time during cell maturation, Neuroscience (2007) ;149: (2):273–85. |
[26] | Gould E , Beylin A , Tanapat P , Reeves A , Shors TJ , Learning enhances adult neurogenesis in the hippocampal formation, Nat Neurosci (1999) ;2: (3):260–5. |
[27] | Hairston IS , Little MT , Scanlon MD , Barakat MT , Palmer TD , Sapolsky RM , et al., Sleep restriction suppresses neurogenesis induced by hippocampus-dependent learning, J Neurophysiol (2005) ;94: (6):4224–33. |
[28] | Ambrogini P , Cuppini R , Cuppini C , Ciaroni S , Cecchini T , Ferri P , et al., Spatial learning affects immature granule cell survival in adult rat dentate gyrus, Neurosci Lett (2000) ;286: (1):21–4. |
[29] | Shors TJ , Miesegaes G , Beylin A , Zhao M , Rydel T , Gould E , Neurogenesis in the adult is involved in the formation of trace memories, Nature (2001) ;410: (6826):372–6. |
[30] | Clelland CD , Choi M , Romberg C , Clemenson GD Jr , Fragniere A , Tyers P , et al., A functional role for adult hippocampal neurogenesis in spatial pattern separation, Science (2009) ;325: (5937):210–3. |
[31] | Sahay A , Scobie KN , Hill AS , O’Carroll CM , Kheirbek MA , Burghardt NS , et al., Increasing adult hippocampal neurogenesis is sufficient to improve pattern separation, Nature (2011) ;472: (7344):466–70. |
[32] | Tronel S , Belnoue L , Grosjean N , Revest JM , Piazza PV , Koehl M , et al., Adult-born neurons are necessary for extended contextual discrimination, Hippocampus (2012) ;22: (2):292–8. |
[33] | Jessberger S , Clark RE , Broadbent NJ , Clemenson GD Jr , , Consiglio A , Lie DC et al., Dentate gyrus-specific knockdown of adult neurogenesis impairs spatial and object recognition memory in adult rats, Learn Mem (2009) ;16: (2):147–54. |
[34] | Snyder JS , Soumier A , Brewer M , Pickel J , Cameron HA , Adult hippocampal neurogenesis buffers stress responses and depressive behaviour, Nature (2011) ;476: (7361):458–61. |
[35] | Planchez B , Lagunas N , Le Guisquet AM , Legrand M , Surget A , Hen R , et al., Increasing Adult Hippocampal Neurogenesis Promotes Resilience in a Mouse Model of Depression, Cells (2021) ;10: (5). |
[36] | Hill AS , Sahay A , Hen R , Increasing Adult Hippocampal Neurogenesis is Sufficient to Reduce Anxiety and Depression-Like Behaviors, Neuropsychopharmacology (2015) ;40: (10):2368–78. |
[37] | Santarelli L , Saxe M , Gross C , Surget A , Battaglia F , Dulawa S , et al., Requirement of hippocampal neurogenesis for the behavioral effects of antidepressants, Science (2003) ;301: (5634)805–9. |
[38] | Terstege DJ , Addo-Osafo K , Teskey GC , Epp JR New neurons in old brains: A cautionary tale for the analysis of neurogenesis in post-mortem tissue. bioRxiv. 2021. |
[39] | Kempermann G , Gage FH , Aigner L , Song H , Curtis MA , Thuret S , et al., Human Adult Neurogenesis: Evidence and Remaining Questions. Cell Stem Cell. 2018. |
[40] | Moreno-Jimenez EP , Flor-Garcia M , Terreros-Roncal J , Rabano A , Cafini F , Pallas-Bazarra N , et al., Adult hippocampal neurogenesis is abundant in neurologically healthy subjects and drops sharply in patients with Alzheimer’s disease, Nat Med (2019) ;25: (4):554–60. |
[41] | Cruz-Pereira JS , Rea K , Nolan YM , O’Leary OF , Dinan TG , Cryan JF , Depression’s Unholy Trinity: Dysregulated Stress, Immunity, and the Microbiome, Annu Rev Psychol (2020) ;71: :49–78. |
[42] | Sarubbo F , Cavallucci V , Pani G , The Influence of Gut Microbiota on Neurogenesis: Evidence and Hopes, Cells (2022) ;11: (3). |
[43] | Cryan JF , O’Riordan KJ , Cowan CSM , Sandhu KV , Bastiaanssen TFS , Boehme M , et al., The Microbiota-Gut-Brain Axis, Physiol Rev (2019) ;99: (4):1877–2013. |
[44] | Kundu P , Lee HU , Garcia-Perez I , Tay EXY , Kim H , Faylon LE , et al., Neurogenesis and prolongevity signaling in young germ-free mice transplanted with the gut microbiota of old mice, Sci Transl Med (2019) ;11: (518):eaau4760. |
[45] | Rei D , Saha S , Haddad M , Haider Rubio A , Perlaza BL , Berard M , et al., Age-associated gut microbiota impairs hippocampus-dependent memory in a vagus-dependent manner. JCI Insight. 2022. |
[46] | Chevalier G , Siopi E , Guenin-Macé L , Pascal M , Laval T , Rifflet A , et al., Effect of gut microbiota on depressive-like behaviors in mice is mediated by the endocannabinoid system, Nature Communications (2020) ;11: (1). |
[47] | Ogbonnaya ES , Clarke G , Shanahan F , Dinan TG , Cryan JF , O’Leary OF , Adult Hippocampal Neurogenesis Is Regulated by the Microbiome, Biol Psychiatry (2015) ;78: (4):e7–9. |
[48] | Scott GA , Terstege DJ , Vu AP , Law S , Evans A , Epp JR , Disrupted Neurogenesis in Germ-Free Mice: Effects of Age and Sex, Front Cell Dev Biol (2020) ;8: :407. |
[49] | Mohle L , Mattei D , Heimesaat MM , Bereswill S , Fischer A , Alutis M , et al., Ly6C(hi) Monocytes Provide a Link between Antibiotic-Induced Changes in Gut Microbiota and Adult Hippocampal Neurogenesis, Cell Rep (2016) ;15: (9):1945–56. |
[50] | Ribeiro MF , Santos AA , Afonso MB , Rodrigues PM , Sa Santos S , Castro RE , et al., Diet-dependent gut microbiota impacts on adult neurogenesis through mitochondrial stress modulation, Brain Commun.fcaa (2020) ;2: (2):165. |
[51] | Kikusui T , Ichikawa S , Mori Y , Maternal deprivation by early weaning increases corticosterone and decreases hippocampal BDNF and neurogenesis in mice, Psychoneuroendocrinology (2009) ;34: (5):762–72. |
[52] | Sahay A , Hen R , Adult hippocampal neurogenesis in depression, Nat Neurosci (2007) ;10: (9):1110–5. |
[53] | Malberg JR , Eisch AJ , Nestler EJ , Duman RS , Chronic Antidepressant Treatment Increases Neurogenesis in Adult Rat Hippocampus, The Journal of Neuroscience (2000) ;20: (24):9104–10. |
[54] | Perera TD , Coplan JD , Lisanby SH , Lipira CM , Arif M , Carpio C , et al., Antidepressant-induced neurogenesis in the hippocampus of adult nonhuman primates, J Neurosci (2007) ;27: (18):4894–901. |
[55] | Madsen TM , Treschow A , Bengzon J , Bolwig TG , Lindvall O , Tingstrom A , Increased neurogenesis in a model of electroconvulsive therapy, Biol Psychiatry (2000) ;47: (12):1043–9. |
[56] | van Praag H , Christie BR , Sejnowski TJ , Gage FH , Running enhances neurogenesis, learning, and long-term potentiation in mice, Proc Natl Acad Sci U S A (1999) ;96: (23):13427–31. |
[57] | Boldrini M , Hen R , Underwood MD , Rosoklija GB , Dwork AJ , Mann JJ , et al., Hippocampal angiogenesis and progenitor cell proliferation are increased with antidepressant use in major depression, Biol Psychiatry (2012) ;72: (7):562–71. |
[58] | Boldrini M , Underwood MD , Hen R , Rosoklija GB , Dwork AJ , John Mann J , et al., Antidepressants increase neural progenitor cells in the human hippocampus, Neuropsychopharmacology (2009) ;34: (11):2376–89. |
[59] | Petrik D , Lagace DC , Eisch AJ , The neurogenesis hypothesis of affective and anxiety disorders: are we mistaking the scaffolding for the building? Neuropharmacology (2012) ;62: (1):21–34. |
[60] | Molendijk ML , Spinhoven P , Polak M , Bus BA , Penninx BW , Elzinga BM , Serum BDNF concentrations as peripheral manifestations of depression: evidence from a systematic review and meta-analyses on 179 associations (N=Mol Psychiatry (2014) ;19: (7):791–800. |
[61] | Nibuya M , Morinobu S , Duman RS , Regulation of BDNF and trkB mRNA in rat brain by chronic electroconvulsive seizure and antidepressant drug treatments, J Neurosci (1995) ;15: (11):7539–47. |
[62] | O’Mahony SM , McVey Neufeld KA , Waworuntu RV , Pusceddu MM , Manurung S , Murphy K , et al., The enduring effects of early-life stress on the microbiota-gut-brain axis are buffered by dietary supplementation with milk fat globule membrane and a prebiotic blend, Eur J Neurosci (2020) ;51: (4):1042–58. |
[63] | Bastiaanssen TFS , Gururajan A , van de Wouw M , Moloney GM , Ritz NL , Long-Smith CM , et al., Volatility as a Concept to Understand the Impact of Stress on the Microbiome, Psychoneuroendocrinology (2021) ;124: :105047. |
[64] | Cussotto S , Strain CR , Fouhy F , Strain RG , Peterson VL , Clarke G , et al., Differential effects of psychotropic drugs on microbiome composition and gastrointestinal function, Psychopharmacology (Berl) (2019) ;236: (5):1671–85. |
[65] | Sudo N , Chida Y , Aiba Y , Sonoda J , Oyama N , Yu XN , et al., Postnatal microbial colonization programs the hypothalamic-pituitary-adrenal system for stress response in mice, J Physiol (2004) ;558: (Pt 1):263–75. |
[66] | Kelly JR , Borre Y , C OB , Patterson E , El Aidy S , Deane J , et al., Transferring the blues: Depression-associated gut microbiota induces neurobehavioural changes in the rat, J Psychiatr Res (2016) ;82: :109–18. |
[67] | Bailey MT , Coe CL , Maternal separation disrupts the integrity of the intestinal microflora in infant rhesus monkeys, Dev Psychobiol (1999) ;35: (2):146–55. |
[68] | Zijlmans MA , Korpela K , Riksen-Walraven JM , de Vos WM , de Weerth C , Maternal prenatal stress is associated with the infant intestinal microbiota, Psychoneuroendocrinology (2015) ;53: :233–45. |
[69] | Galley JD , Nelson MC , Yu Z , Dowd SE , Walter J , Kumar PS , et al., Exposure to a social stressor disrupts the community structure of the colonic mucosa-associated microbiota, BMC Microbiol (2014) ;14: :189. |
[70] | Bravo JA , Forsythe P , Chew MV , Escaravage E , Savignac HM , Dinan TG , et al., Ingestion of Lactobacillus strain regulates emotional behavior and central GABA receptor expression in a mouse via the vagus nerve, Proc Natl Acad Sci U S A (2011) ;108: (38):16050–5. |
[71] | Guo G , Jia KR , Shi Y , Liu XF , Liu KY , Qi W , et al., Psychological stress enhances the colonization of the stomach by Helicobacter pylori in the BALB/c mouse, Stress (2009) ;12: (6):478–85. |
[72] | Hemmings SMJ , Malan-Muller S , van den Heuvel LL , Demmitt BA , Stanislawski MA , Smith DG , et al., The Microbiome in Posttraumatic Stress Disorder and Trauma-Exposed Controls: An Exploratory Study, Psychosom Med (2017) ;79: (8):936–46. |
[73] | Ayaz M , Subhan F , Ahmed J , Khan AU , Ullah F , Ullah I , et al., Sertraline enhances the activity of antimicrobial agents against pathogens of clinical relevance, J Biol Res (Thessalon) (2015) ;22: (1):4. |
[74] | Lyte M , Daniels KM , Schmitz-Esser S , Fluoxetine-induced alteration of murine gut microbial community structure: evidence for a microbial endocrinology-based mechanism of action responsible for fluoxetine-induced side effects, PeerJ (2019) ;7: :e6199. |
[75] | Lukic I , Getselter D , Ziv O , Oron O , Reuveni E , Koren O , et al., Antidepressants affect gut microbiota and Ruminococcus flavefaciens is able to abolish their effects on depressive-like behavior, Transl Psychiatry (2019) ;9: (1):133. |
[76] | van Praag H , Shubert T , Zhao C , Gage FH , Exercise enhances learning and hippocampal neurogenesis in aged mice, J Neurosci (2005) ;25: (38):8680–5. |
[77] | Aimone JB , Wiles J , Gage FH , Potential role for adult neurogenesis in the encoding of time in new memories, Nat Neurosci (2006) ;9: (6):723–7. |
[78] | Kempermann G , Kuhn HG , Gage FH , More hippocampal neurons in adult mice living in an enriched environment, Nature (1997) ;386: (6624):493–5. |
[79] | Schloesser RJ , Lehmann M , Martinowich K , Manji HK , Herkenham M , Environmental enrichment requires adult neurogenesis to facilitate the recovery from psychosocial stress, Mol Psychiatry (2010) ;15: (12):1152–63. |
[80] | Duman CH , Schlesinger L , Russell DS , Duman RS , Voluntary exercise produces antidepressant and anxiolytic behavioral effects in mice, Brain Res (2008) ;1199: :148–58. |
[81] | Laske C , Banschbach S , Stransky E , Bosch S , Straten G , Machann J , et al., Exercise-induced normalization of decreased BDNF serum concentration in elderly women with remitted major depression, Int J Neuropsychopharmacol (2010) ;13: (5):595–602. |
[82] | Klempin F , Beis D , Mosienko V , Kempermann G , Bader M , Alenina N , Serotonin is required for exercise-induced adult hippocampal neurogenesis, J Neurosci (2013) ;33: (19):8270–5. |
[83] | Clarke SF , Murphy EF , O’Sullivan O , Lucey AJ , Humphreys M , Hogan A , et al., Exercise and associated dietary extremes impact on gut microbial diversity, Gut (2014) ;63: (12):1913–20. |
[84] | Bressa C , Bailen-Andrino M , Perez-Santiago J , Gonzalez-Soltero R , Perez M , Montalvo-Lominchar MG , et al., Differences in gut microbiota profile between women with active lifestyle and sedentary women, PLoS One (2017) ;12: (2):e0171352. |
[85] | Monda V , Villano I , Messina A , Valenzano A , Esposito T , Moscatelli F , et al., Exercise Modifies the Gut Microbiota with Positive Health Effects, Oxid Med Cell Longev (2017) ;2017: :3831972. |
[86] | Cavallucci V , Fidaleo M , Pani G , Nutrients and neurogenesis: the emerging role of autophagy and gut microbiota, Curr Opin Pharmacol (2020) ;50: :46–52. |
[87] | Lupori L , Cornuti S , Mazziotti R , Borghi E , Ottaviano E , Cas MD , et al., The gut microbiota of environmentally enriched mice regulates visual cortical plasticity, Cell Rep (2022) ;38: (2):110212. |
[88] | Lopez-Otin C , Blasco MA , Partridge L , Serrano M , Kroemer G , The hallmarks of aging, Cell (2013) ;153: (6):1194–217. |
[89] | Tran L , Greenwood-Van Meerveld B , Age-associated remodeling of the intestinal epithelial barrier, J Gerontol A Biol Sci Med Sci (2013) ;68: (9):1045–56. |
[90] | Konturek PC , Haziri D , Brzozowski T , Hess T , Heyman S , Kwiecien S , et al., Emerging role of fecal microbiota therapy in the treatment of gastrointestinal and extra-gastrointestinal diseases, J Physiol Pharmacol (2015) ;66: (4):483–91. |
[91] | Kempermann G , Kuhn HG , Gage FH , Experience-induced neurogenesis in the senescent dentate gyrus, J Neurosci (1998) ;18: (9):3206–12. |
[92] | Klempin F , Kempermann G , Adult hippocampal neurogenesis and aging, Eur Arch Psychiatry Clin Neurosci (2007) ;257: (5):271–80. |
[93] | Diaz-Moreno M , Armenteros T , Gradari S , Hortiguela R , Garcia-Corzo L , Fontan-Lozano A , et al., Noggin rescues age-related stem cell lossin the brain of senescent mice with neurodegenerative pathology, Proc Natl Acad Sci U S A (2018) ;115: (45):11625–30. |
[94] | Kalamakis G , Brune D , Ravichandran S , Bolz J , Fan W , Ziebell F , et al., Quiescence Modulates Stem Cell Maintenance and Regenerative Capacity in the Aging Brain, Cell (2019) ;176: (6):1407–19 e14. |
[95] | Encinas JM , Michurina TV , Peunova N , Park JH , Tordo J , Peterson DA , et al., Division-coupled astrocytic differentiation and age-related depletion of neural stem cells in the adult hippocampus, Cell Stem Cell (2011) ;8: (5):566–79. |
[96] | Imayoshi I , Sakamoto M , Ohtsuka T , Takao K , Miyakawa T , Yamaguchi M , et al., Roles of continuous neurogenesis in the structural and functional integrity of the adult forebrain, Nat Neurosci (2008) ;11: (10):1153–61. |
[97] | Navarro Negredo P , Yeo RW , Brunet A , Aging and Rejuvenation of Neural Stem Cells and Their Niches, Cell Stem Cell (2020) ;27: (2):202–23. |
[98] | Kramer AF , Erickson KI , Colcombe SJ , Exercise, cognition, and the aging brain, J Appl Physiol (2006) ;101: (4):1237–42. |
[99] | Kramer AF , Hahn S , Cohen NJ , Banich MT , McAuley E , Harrison CR , et al., Ageing, fitness and neurocognitive function, Nature (1999) ;400: (6743):418–9. |
[100] | Biagi E , Franceschi C , Rampelli S , Severgnini M , Ostan R , Turroni S , et al., Gut Microbiota and Extreme Longevity, Curr Biol (2016) ;26: (11):1480–5. |
[101] | Biagi E , Rampelli S , Turroni S , Quercia S , Candela M , Brigidi P , The gut microbiota of centenarians: Signatures of longevity in the gut microbiota profile, Mech Ageing Dev (2017) ;165: (Pt B):180–4. |
[102] | Smith P , Willemsen D , Popkes M , Metge F , Gandiwa E , Reichard M , et al., Regulation of life span by the gut microbiota in the short-lived African turquoise killifish, Elife (2017) ;6: :e27014. |
[103] | Dinan TG , Cryan JF , Gut instincts: microbiota as a key regulator of brain development, ageing and neurodegeneration, J Physiol (2017) ;595: (2):489–503. |
[104] | Claesson MJ , Jeffery IB , Conde S , Power SE , O’Connor EM , Cusack S , et al., Gut microbiota composition correlates with diet and health in the elderly, Nature. (2012) ;488: (7410):178–84. |
[105] | Jackson MA , Jeffery IB , Beaumont M , Bell JT , Clark AG , Ley RE , et al., Signatures of early frailty in the gut microbiota, Genome Med (2016) ;8: (1):8. |
[106] | Odamaki T , Kato K , Sugahara H , Hashikura N , Takahashi S , Xiao JZ , et al., Age-related changes in gut microbiota composition from newborn to centenarian: a cross-sectional study, BMC Microbiol (2016) ;16: :90. |
[107] | Biagi E , Nylund L , Candela M , Ostan R , Bucci L , Pini E , et al., Through ageing, and beyond: gut microbiota and inflammatory status in seniors and centenarians, PLoS One (2010) ;5: (5):e 10667. |
[108] | Snyder DL , Pollard M , Wostmann BS , Luckert P , Life span, morphology, and pathology of diet-restricted germ-free and conventional Lobund-Wistar rats, J Gerontol (1990) ;45: (2):B52–8. |
[109] | Ghosh TS , Shanahan F , O’Toole PW , The gut microbiome as a modulator of healthy ageing. Nat Rev Gastroenterol Hepatol. 2022. |
[110] | Ait-Belgnaoui A , Colom A , Braniste V , Ramalho L , Marrot A , Cartier C , et al., Probiotic gut effect prevents the chronic psychological stress-induced brain activity abnormality in mice, Neurogastroenterol Motil (2014) ;26: (4):510–20. |
[111] | Mohammadi G , Dargahi L , Naserpour T , Mirzanejad Y , Alizadeh SA , Peymani A , et al., Probiotic mixture of Lactobacillus helveticus Rand Bifidobacterium longum Rattenuates hippocampal apoptosis induced by lipopolysaccharide in rats, Int Microbiol (2019) ;22: (3):317–23. |
[112] | Mohammadi G , Dargahi L , Peymani A , Mirzanejad Y , Alizadeh SA , Naserpour T , et al., The Effects of Probiotic Formulation Pretreatment (Lactobacillus helveticus Rand Bifidobacterium longum Ron a Lipopolysaccharide Rat Model, J Am Coll Nutr (2019) ;38: (3):209–17. |
[113] | Wilmanski T , Diener C , Rappaport N , Patwardhan S , Wiedrick J , Lapidus J , et al., Gut microbiome pattern reflects healthy ageing and predicts survival in humans, Nat Metab (2021) ;3: (2):274–86. |
[114] | Fransen F , van Beek AA , Borghuis T , Aidy SE , Hugenholtz F , van der Gaast-de Jongh C , et al., Aged Gut Microbiota Contributes to Systemical Inflammaging after Transfer to Germ-Free Mice, Front Immunol (2017) ;8: :1385. |
[115] | Robison LS , Albert NM , Camargo LA , Anderson BM , Salinero AE , Riccio DA , et al., High-Fat Diet-Induced Obesity Causes Sex-Specific Deficits in Adult Hippocampal Neurogenesis in Mice, eNeuro (2020) ;7: (1). |
[116] | Lindqvist A , Mohapel P , Bouter B , Frielingsdorf H , Pizzo D , Brundin P , et al., High-fat diet impairs hippocampal neurogenesis in male rats, Eur J Neurol (2006) ;13: (12):1385–8. |
[117] | Klein C , Jonas W , Iggena D , Empl L , Rivalan M , Wiedmer P , et al., Exercise prevents high-fat diet-induced impairment of flexible memory expression in the water maze and modulates adult hippocampal neurogenesis in mice, Neurobiol Learn Mem (2016) ;131: :26–35. |
[118] | Parikh I , Guo J , Chuang KH , Zhong Y , Rempe RG , Hoffman JD , et al., Caloric restriction preserves memory and reduces anxiety of aging mice with early enhancement of neurovascular functions, Aging (Albany NY) (2016) ;8: (11):2814–26. |
[119] | Brandhorst S , Choi IY , Wei M , Cheng CW , Sedrakyan S , Navarrete G , et al., A Periodic Diet that Mimics Fasting Promotes Multi-System Regeneration, Enhanced Cognitive Performance, and Healthspan, Cell Metab (2015) ;22: (1):86–99. |
[120] | Graja A , Ghattassi K , Boudhina N , Bouzid MA , Chahed H , Ferchichi S , et al., Effect of Ramadan intermittent fasting on cognitive, physical and biochemical responses to strenuous short-term exercises in elite young female handball players. Physiol Behav. 2020:113241. |
[121] | Ooi TC , Meramat A , Rajab NF , Shahar S , Ismail IS , Azam AA , et al., Intermittent Fasting Enhanced the Cognitive Function in Older Adults with Mild Cognitive Impairment by Inducing Biochemical and Metabolic changes: A 3-Year Progressive Study, Nutrients (2020) ;12: (9):2644. |
[122] | Singh R , Lakhanpal D , Kumar S , Sharma S , Kataria H , Kaur M , et al., Late-onset intermittent fasting dietary restriction as a potential intervention to retard age-associated brain function impairments in male rats, Age (Dordr) (2012) ;34: (4):917–33. |
[123] | Dal-Pan A , Pifferi F , Marchal J , Picq JL , Aujard F , Consortium R , Cognitive performances are selectively enhanced during chronic caloric restriction or resveratrol supplementation in a primate, PLoS One (2011) ;6: (1):e16581. |
[124] | Bellush LL , Wright AM , Walker JP , Kopchick J , Colvin RA , Caloric restriction and spatial learning in old mice, Physiol Behav (1996) ;60: (2):541–7. |
[125] | Ma L , Wang R , Dong W , Zhao Z , Caloric restriction can improve learning and memory in C57/BL mice probably via regulation of the AMPK signaling pathway, Exp Gerontol (2018) ;102: :28–35. |
[126] | Kuhla A , Lange S , Holzmann C , Maass F , Petersen J , Vollmar B , et al., Lifelong caloric restriction increases working memory in mice, PLoS One (2013) ;8: (7):e68778. |
[127] | Means LW , Higgins JL , Fernandez TJ , Mid-life onset of dietary restriction extends life and prolongs cognitive functioning, Physiol Behav (1993) ;54: (3):503–8. |
[128] | Lee J , Duan W , Mattson MP , Evidence that brain-derived neurotrophic factor is required for basal neurogenesis and mediates, in part, the enhancement of neurogenesis by dietary restriction in the hippocampus of adult mice, J Neurochem (2002) ;82: (6):1367–75. |
[129] | Lee J , Seroogy KB , Mattson MP , Dietary restriction enhances neurotrophin expression and neurogenesis in the hippocampus of adult mice, J Neurochem (2002) ;80: (3):539–47. |
[130] | Gibson GR , Hutkins R , Sanders ME , Prescott SL , Reimer RA , Salminen SJ , et al., Expert consensus document: The International Scientific Association for Probiotics and Prebiotics (ISAPP) consensus statement on the definition and scope of prebiotics, Nature Reviews Gastroenterology & Hepatology (2017) ;14: :491–502. |
[131] | Fernandez-Fernandez L , Comes G , Bolea I , Valente T , Ruiz J , Murtra P , et al., LMN diet, rich in polyphenols and polyunsaturated fatty acids, improves mouse cognitive decline associated with aging and Alzheimer’s disease, Behav Brain Res (2012) ;228: (2):261–71. |
[132] | Boehme M , van de Wouw M , Bastiaanssen TFS , Olavarria-Ramirez L , Lyons K , Fouhy F , et al., Mid-life microbiota crises: middle age is associated with pervasive neuroimmune alterations that are reversed by targeting the gut microbiome, Mol Psychiatry (2020) ;25: (10):2567–83. |
[133] | An L , Zhang YZ , Yu NJ , Liu XM , Zhao N , Yuan L , et al., The total flavonoids extracted from Xiaobuxin-Tang up-regulate the decreased hippocampal neurogenesis and neurotrophic molecules expression in chronically stressed rats, Prog Neuropsychopharmacol Biol Psychiatry (2008) ;32: (6):1484–90. |
[134] | Casadesus G , Shukitt-Hale B , Stellwagen HM , Zhu X , Lee HG , Smith MA , et al., Modulation of hippocampal plasticity and cognitive behavior by short-term blueberry supplementation in aged rats, Nutr Neurosci (2004) ;7: (5-6):309–16. |
[135] | Sarubbo F , Moranta D , Pani G , Dietary polyphenols and neurogenesis: Molecular interactions and implication for brain ageing and cognition, Neurosci Biobehav Rev (2018) ;90: :456–70. |
[136] | Petrella C , Strimpakos G , Torcinaro A , Middei S , Ricci V , Gargari G , et al., Proneurogenic and neuroprotective effect of a multi strain probiotic mixture in a mouse model of acute inflammation: Involvement of the gut-brain axis, Pharmacol Res (2021) ;172: :105795. |
[137] | Zhang Y , Fan Q , Hou Y , Zhang X , Yin Z , Cai X , et al., Bacteroides species differentially modulate depression-like behavior via gut-brain metabolic signaling, Brain Behav Immun (2022) ;102: :11–22. |
[138] | Spichak S , Guzzetta KE , O’Leary OF , Clarke G , Dinan TG , Cryan JF , Without a bug’s life: Germ-free rodents to interrogate microbiota-gut-neuroimmune interactions, Drug Discovery Today (2018) ;28: :79–93. |
[139] | Wei GZ , Martin KA , Xing PY , Agrawal R , Whiley L , Wood TK , et al., Tryptophan-metabolizing gut microbes regulate adult neurogenesis via the aryl hydrocarbon receptor, Proc Natl Acad Sci U S A (2021) ;118: (27):e2021091118. |
[140] | Clarke G , Grenham S , Scully P , Fitzgerald P , Moloney RD , Shanahan F , et al., The microbiome-gut-brain axis during early life regulates the hippocampal serotonergic system in a sex-dependent manner, Mol Psychiatry (2013) ;18: (6):666–73. |
[141] | Yano JM , Yu K , Donaldson GP , Shastri GG , Ann P , Ma L , et al., Indigenous bacteria from the gut microbiota regulate host serotonin biosynthesis, Cell (2015) ;161: (2):264–76. |
[142] | Grill MF , Maganti RK , Neurotoxic effects associated with antibiotic use: management considerations, Br J Clin Pharmacol (2011) ;72: (3):381–93. |
[143] | Mehta RS , Lochhead P , Wang Y , Ma W , Nguyen LH , Kochar B , et al., Association of midlife antibiotic use with subsequent cognitivefunction in women, PLoS One (2022) ;17: (3):e0264649. |
[144] | Han S , Shannahan S , Pellish R , Fecal Microbiota Transplant: Treatment Options for Clostridium difficile Infection in the Intensive Care Unit, J Intensive Care Med (2016) ;31: (9):577–86. |
[145] | Sorbara MT , Pamer EG , Microbiome-based therapeutics. Nat Rev Microbiol. 2022. |
[146] | D’Amato A , Di Cesare Mannelli L , Lucarini E , Man AL , Le Gall G , Branca JJV , et al., Faecal microbiota transplant from aged donor mice affects spatial learning and memory via modulating hippocampal synaptic plasticity- and neurotransmission-related proteins in young recipients, Microbiome (2020) ;8: (1):140. |
[147] | Kim N , Jeon SH , Ju IG , Gee MS , Do J , Oh MS , et al., Transplantation of gut microbiota derived from Alzheimer’s disease mouse model impairs memory function and neurogenesis in C57BL/6 mice, Brain Behav Immun (2021) ;98: :357–65. |
[148] | Boehme M , Guzzetta KE , Bastiaanssen TFS , van de Wouw M , Moloney GM , Gual-Grau A , et al., Microbiota from young mice counteracts selective age-associated behavioral deficits, Nature Aging (2021) ;1: (8):666–76. |
[149] | Ma X , Xiao W , Li H , Pang P , Xue F , Wan L , et al., Metformin restores hippocampal neurogenesis and learning and memory via regulating gut microbiota in the obese mouse model, Brain Behav Immun (2021) ;95: :68–83. |
[150] | Kazemi A , Noorbala AA , Azam K , Eskandari MH , Djafarian K , Effect of probiotic and prebiotic vs placebo on psychological outcomes in patients with major depressive disorder: A randomized clinical trial, Clin Nutr (2019) ;38: (2):522–8. |
[151] | Wallace CJK , Milev RV , The Efficacy, Safety, and Tolerability of Probiotics on Depression: Clinical Results From an Open-Label Pilot Study, Front Psychiatry (2021) ;12: :618279. |
[152] | Vauzour D , Dietary polyphenols as modulators of brain functions: biological actions and molecular mechanisms underpinning their beneficial effects, Oxid Med Cell Longev (2012) ;2012: :914273. |
[153] | Bayes J , Schloss J , Sibbritt D , Effects of Polyphenols in a Mediterranean Diet on Symptoms of Depression: A Systematic Literature Review, Adv Nutr (2020) ;11: (3):602–15. |
[154] | Anjaneyulu M , Chopra K , Kaur I , Antidepressant activity of quercetin, a bioflavonoid, in streptozotocin-induced diabetic mice, J Med Food (2003) ;6: (4):391–5. |
[155] | Kulkarni SK , Bhutani MK , Bishnoi M , Antidepressant activity of curcumin: involvement of serotonin and dopamine system, Psychopharmacology (Berl) (2008) ;201: (3):435–42. |
[156] | Bouayed J , Polyphenols: A Potential New Strategy for the Prevention and Treatment of Anxiety and Depression, Current Nutrition & Food Science (2010) ;6: :13–8. |
[157] | Parletta N , Zarnowiecki D , Cho J , Wilson A , Bogomolova S , Villani A , et al., A Mediterranean-style dietary intervention supplemented with fish oil improves diet quality and mental health in people with depression: A randomized controlled trial (HELFIMED), Nutr Neurosci (2019) ;22: (7):474–87. |
[158] | Ghosh TS , Rampelli S , Jeffery IB , Santoro A , Neto M , Capri M , et al., Mediterranean diet intervention alters the gut microbiome in older people reducing frailty and improving health status: the NU-AGE 1-year dietary intervention across five European countries, Gut (2020) ;69: (7):1218–28. |
[159] | Grundy T , Toben C , Jaehne EJ , Corrigan F , Baune BT , Long-term omega-3 supplementation modulates behavior, hippocampal fatty acid concentration, neuronal progenitor proliferation and central TNF-alpha expression in 7 month old unchallenged mice. Frontiers in Cellular Neuroscience. 2014;8. |
[160] | Beltz BS , Tlusty MF , Benton JL , Sandeman DC , Omega-3 fatty acids upregulate adult neurogenesis, Neurosci Lett (2007) ;415: (2):154–8. |
[161] | Borsini A , Stangl D , Jeffries AR , Pariante CM , Thuret S , The role of omega-3 fatty acids in preventing glucocorticoid-induced reduction in human hippocampal neurogenesis and increase in apoptosis, Transl Psychiatry (2020) ;10: (1):219. |
[162] | Donoso F , Egerton S , Bastiaanssen TFS , Fitzgerald P , Gite S , Fouhy F , et al., Polyphenols selectively reverse early-life stress-induced behavioural, neurochemical and microbiota changes in the rat, Psychoneuroendocrinology (2020) ;116: :104673. |
[163] | Egerton S , Donoso F , Fitzgerald P , Gite S , Fouhy F , Whooley J , et al., Investigating the potential of fish oil as a nutraceutical in an animal model of early life stress, Nutr Neurosci (2020) ;25: (2):1–23. |
[164] | van de Wouw M , Boehme M , Lyte JM , Wiley N , Strain C , O’Sullivan O , et al., Short-chain fatty acids: microbial metabolites that alleviate stress-induced brain-gut axis alterations, J Physiol (2018) ;596: (20):4923–44. |
[165] | Erny D , Dokalis N , Mezo C , Castoldi A , Mossad O , Staszewski O , et al., Microbiota-derived acetate enables the metabolic fitness of the brain innate immune system during health and disease, Cell Metab (2021) ;33: (11):2260–76 e7. |
[166] | Val-Laillet D , Guerin S , Coquery N , Nogret I , Formal M , Rome V , et al., Oral sodium butyrate impacts brain metabolism and hippocampal neurogenesis, with limited effects on gut anatomy and function in pigs, FASEB J (2018) ;32: (4):2160–71. |
[167] | Valvassori SS , Varela RB , Arent CO , Dal-Pont GC , Bobsin TS , Budni J , et al., Sodium Butyrate Functions as an Antidepressant and Improves Cognition with Enhanced Neurotrophic Expression in Models of Maternal Deprivation and Chronic Mild Stress, Curr Neurovasc Res (2014) ;11: (4):359–66. |
[168] | Matt SM , Allen JM , Lawson MA , Mailing LJ , Woods JA , Johnson RW , Butyrate and Dietary Soluble Fiber Improve Neuroinflammation Associated With Aging in Mice, Front Immunol (2018) ;9: :1832. |
[169] | Savignac HM , Corona G , Mills H , Chen L , Spencer JPE , Tzortzis G , et al., Prebiotic feeding elevates central brain derived neurotrophic factor, N-methyl-D-aspartate receptor subunits and D-serine, Neurochem Int (2013) ;63: (8):756–64. |
[170] | Williams S , Chen L , Savignac HM , Tzortzis G , Anthony DC , Burnet PWJ , Neonatal prebiotic (BGOS) supplementation increases the levels of synaptophysin, GluN2A-subunits and BDNF proteins in the adult rat hippocampus, Synapse (2016) ;70: (3):121–5. |
[171] | Karimipour M , Rahbarghazi R , Tayefi H , Shimia M , Ghanadian M , Mahmoudi J , et al., Quercetin promotes learning and memory performance concomitantly with neural stem/progenitor cell proliferation and neurogenesis in the adult rat dentate gyrus, Int J Dev Neurosci (2019) ;74: :18–26. |
[172] | Sawamoto A , Okuyama S , Amakura Y , Yoshimura M , Yamada T , Yokogoshi H , et al., 3,5,6,7,8,3 ’,4 ’-Heptamethoxyflavone Ameliorates Depressive-Like Behavior and Hippocampal Neurochemical Changes in Chronic Unpredictable Mild Stressed Mice by Regulating the Brain-Derived Neurotrophic Factor: Requirement for ERK Activation, International Journal of Molecular Sciences (2017) ;18: (10). |
[173] | Okuyama S , Kotani Y , Yamamoto K , Sawamoto A , Sugawara K , Sudo M , et al., The peel of Citrus kawachiensis (kawachi bankan) ameliorates microglial activation, tau hyper-phosphorylation, and suppression of neurogenesis in the hippocampus of senescence-accelerated mice, Biosci Biotechnol Biochem (2018) ;82: (5):869–78. |
[174] | Lee Y , Jeon SJ , Lee HE , Jung IH , Jo YW , Lee S , et al., Spinosin, a C-glycoside flavonoid, enhances cognitive performance and adult hippocampal neurogenesis in mice, Pharmacol Biochem Behav (2016) ;145: :9–16. |
[175] | Lee S , Kim DH , Lee DH , Jeon SJ , Lee CH , Son KH , et al., Oroxylin A, a flavonoid, stimulates adult neurogenesis in the hippocampal dentate gyrus region of mice, Neurochem Res (2010) ;35: (11):1725–32. |
[176] | Jeon SJ , Rhee SY , Seo JE , Bak HR , Lee SH , Ryu JH , et al., Oroxylin Aincreases BDNF production by activation of MAPK-CREB pathway in ratprimary cortical neuronal culture, Neurosci Res (2011) ;69: (3):214–22. |
[177] | Erny D , Hrabe de Angelis AL , Jaitin D , Wieghofer P , Staszewski O , David E , et al., Host microbiota constantly control maturation and function of microglia in the CNS, Nat Neurosci (2015) ;18: (7):965–77. |
[178] | Sierra A , Encinas JM , Deudero JJ , Chancey JH , Enikolopov G , Overstreet-Wadiche LS , et al., Microglia shape adult hippocampal neurogenesis through apoptosis-coupled phagocytosis, Cell Stem Cell (2010) ;7: (4):483–95. |
[179] | Diaz-Aparicio I , Paris I , Sierra-Torre V , Plaza-Zabala A , Rodriguez-Iglesias N , Marquez-Ropero M , et al., Microglia Actively Remodel Adult Hippocampal Neurogenesis through the Phagocytosis Secretome, J Neurosci (2020) ;40: (7):1453–82. |
[180] | Ekdahl CT , Claasen JH , Bonde S , Kokaia Z , Lindvall O , Inflammation is detrimental for neurogenesis in adult brain, Proc Natl Acad Sci U S A (2003) ;100: (23):13632–7. |
[181] | Monje ML , Toda H , Palmer TD , Inflammatory blockade restores adult hippocampal neurogenesis, Science (2003) ;302: (5651)1760–5. |
[182] | Green HF , Nolan YM , Unlocking mechanisms in interleukin-1beta-induced changes in hippocampal neurogenesis–a role for GSK-3beta and TLX, Transl Psychiatry.e (2012) ;2: :194. |
[183] | Pawley LC , Hueston CM , O’Leary JD , Kozareva DA , Cryan JF , O’Leary OF , et al., Chronic intrahippocampal interleukin-1beta overexpression in adolescence impairs hippocampal neurogenesis but not neurogenesis-associated cognition, Brain Behav Immun (2020) ;83: :172–9. |
[184] | Luczynski P , McVey Neufeld KA , Oriach CS , Clarke G , Dinan TG , Cryan JF , Growing up in a Bubble: Using Germ-Free Animals to Assess the Influence of the Gut Microbiota on Brain and Behavior, Int J Neuropsychopharmacol (2016) ;19: (8):pyw020. |
[185] | Gonzalez-Perez G , Lamouse-Smith ES , Gastrointestinal Microbiome Dysbiosis in Infant Mice Alters Peripheral CD8(+) T Cell Receptor Signaling, Front Immunol (2017) ;8: :265. |
[186] | Yu AI , Zhao L , Eaton KA , Ho S , Chen J , Poe S , et al., Gut MicrobiotaModulate CD8 T Cell Responses to Influence Colitis-AssociatedTumorigenesis, Cell Rep (2020) ;31: (1):107471. |
[187] | Dillon SM , Lee EJ , Kotter CV , Austin GL , Gianella S , Siewe B , et al., Gut dendritic cell activation links an altered colonic microbiome to mucosal and systemic T-cell activation in untreated HIV-1 infection, Mucosal Immunol (2016) ;9: (1):24–37. |
[188] | Edelblum KL , Sharon G , Singh G , Odenwald MA , Sailer A , Cao S , et al., The Microbiome Activates CD4 T-cell-mediated Immunity to Compensate for Increased Intestinal Permeability, Cell Mol Gastroenterol Hepatol (2017) ;4: (2):285–97. |
[189] | Medina-Rodriguez EM , Madorma D , O’Connor G , Mason BL , Han D , Deo SK , et al., Identification of a Signaling Mechanism by Which the Microbiome Regulates Th17 Cell-Mediated Depressive-Like Behaviors in Mice, Am J Psychiatry (2020) ;177: (10):974–90. |
[190] | Ivanov II , Atarashi K , Manel N , Brodie EL , Shima T , Karaoz U , et al., Induction of intestinal Th17 cells by segmented filamentous bacteria, Cell (2009) ;139: (3):485–98. |
[191] | Walker TL , Schallenberg S , Rund N , Gronnert L , Rust R , Kretschmer K , et al., T Lymphocytes Contribute to the Control of Baseline Neural Precursor Cell Proliferation but Not the Exercise-Induced Up-Regulation of Adult Hippocampal Neurogenesis, Front Immunol (2018) ;9: :2856. |
[192] | Zarif H , Nicolas S , Guyot M , Hosseiny S , Lazzari A , Canali MM , et al., CD8(+) T cells are essential for the effects of enriched environment on hippocampus-dependent behavior, hippocampal neurogenesis and synaptic plasticity, Brain Behav Immun (2018) ;69: :235–54. |
[193] | Wolf SA , Steiner B , Akpinarli A , Kammertoens T , Nassenstein C , Braun A , et al., CD4-positive T lymphocytes provide a neuroimmunological link in the control of adult hippocampal neurogenesis, J Immunol (2009) ;182: (7):3979–84. |
[194] | Langrish CL , Chen Y , Blumenschein WM , Mattson J , Basham B , Sedgwick JD , et al., IL-23 drives a pathogenic T cell population that induces autoimmune inflammation, J Exp Med (2005) ;201: (2):233–40. |
[195] | Liu Q , Xin W , He P , Turner D , Yin J , Gan Y , et al., Interleukin-17 inhibits adult hippocampal neurogenesis, Sci Rep (2014) ;4: :7554. |
[196] | Beurel E , Harrington LE , Jope RS , Inflammatory T helper 17 cells promote depression-like behavior in mice, Biol Psychiatry (2013) ;73: (7):622–30. |
[197] | Suarez AN , Hsu TM , Liu CM , Noble EE , Cortella AM , Nakamoto EM , et al., Gut vagal sensory signaling regulates hippocampus function through multi-order pathways, Nat Commun (2018) ;9: (1):2181. |
[198] | Liu Y , Sanderson D , Mian MF , McVey Neufeld KA , Forsythe P , Loss of vagal integrity disrupts immune components of the microbiota-gut-brain axis and inhibits the effect of Lactobacillus rhamnosus on behavior and the corticosterone stress response, Neuropharmacology (2021) ;195: :108682. |
[199] | O’Leary OF , Felice D , Galimberti S , Savignac HM , Bravo JA , Crowley T , et al., GABAB(1) receptor subunit isoforms differentially regulate stress resilience, Proc Natl Acad Sci U S A (2014) ;111: (42):15232–7. |
[200] | Biggio F , Gorini G , Utzeri C , Olla P , Marrosu F , Mocchetti I , et al., Chronic vagus nerve stimulation induces neuronal plasticity in the rat hippocampus, Int J Neuropsychopharmacol (2009) ;12: (9):1209–21. |
[201] | Revesz D , Tjernstrom M , Ben-Menachem E , Thorlin T , Effects of vagus nerve stimulation on rat hippocampal progenitor proliferation, Exp Neurol (2008) ;214: (2):259–65. |
[202] | Gebhardt N , Bar KJ , Boettger MK , Grecksch G , Keilhoff G , Reichart R , et al., Vagus nerve stimulation ameliorated deficits in one-way active avoidance learning and stimulated hippocampal neurogenesis in bulbectomized rats, Brain Stimul (2013) ;6: (1):78–83. |
[203] | O’Leary OF , Ogbonnaya ES , Felice D , Levone BR , L CC , Fitzgerald P , et al., The vagus nerve modulates BDNF expression and neurogenesis in the hippocampus, Eur Neuropsychopharmacol (2018) ;28: (2):307–16. |
[204] | Yang LL , Millischer V , Rodin S , MacFabe DF , Villaescusa JC , Lavebratt C , Enteric short-chain fatty acids promote proliferation of human neural progenitor cells, J Neurochem (2020) ;154: (6):635–46. |
[205] | Stilling RM , van de Wouw M , Clarke G , Stanton C , Dinan TG , Cryan JF , The neuropharmacology of butyrate: The bread and butter of the microbiota-gut-brain axis? Neurochem Int (2016) ;99: :110–32. |
[206] | Dash PK , Orsi SA , Moore AN , Histone deactylase inhibition combined with behavioral therapy enhances learning and memory following traumatic brain injury, Neuroscience (2009) ;163: (1):1–8. |
[207] | Barichello T , Generoso JS , Simoes LR , Faller CJ , Ceretta RA , Petronilho F , et al., Sodium Butyrate Prevents Memory Impairment by Re-establishing BDNF and GDNF Expression in Experimental Pneumococcal Meningitis, Mol Neurobiol (2015) ;52: (1):734–40. |
[208] | Gheorghe CE , Martin JA , Manriquez FV , Dinan TG , Cryan JF , Clarke G , Focus on the essentials: tryptophan metabolism and the microbiome-gut-brain axis, Curr Opin Pharmacol (2019) ;48: :137–45. |
[209] | Jenkins TA , Nguyen JCD , Polglaze KE , Bertrand PP , Influence of Tryptophan and Serotonin on Mood and Cognition with a Possible Role of the Gut-Brain Axis, Nutrients (2016) ;8: (1). |
[210] | Reigstad CS , Salmonson CE , Rainey JF , 3rd, Szurszewski JH , Linden DR , Sonnenburg JL , et al., Gut microbes promote colonic serotonin production through an effect of short-chain fatty acids on enterochromaffin cells, FASEB J (2015) ;29: (4):1395–403. |
[211] | Alenina N , Klempin F , The role of serotonin in adult hippocampal neurogenesis, Behav Brain Res (2015) ;277: :49–57. |
[212] | Benninghoff J , Gritti A , Rizzi M , Lamorte G , Schloesser RJ , Schmitt A , et al., Serotonin depletion hampers survival and proliferation in neurospheres derived from adult neural stem cells, Neuropsychopharmacology (2010) ;35: (4):893–903. |
[213] | Lauder JM , Krebs H , Serotonin as a differentiation signal in early neurogenesis, Dev Neurosci (1978) ;1: (1):15–30. |
[214] | Moors M , Bose R , Johansson-Haque K , Edoff K , Okret S , Ceccatelli S , Dickkopf 1 mediates glucocorticoid-induced changes in human neural progenitor cell proliferation and differentiation, Toxicol Sci (2012) ;125: (2):488–95. |
[215] | Odaka H , Numakawa T , Yoshimura A , Nakajima S , Adachi N , Ooshima Y , et al., Chronic glucocorticoid exposure suppressed the differentiation and survival of embryonic neural stem/progenitor cells: Possible involvement of ERK and PI3K/Akt signaling in the neuronal differentiation, Neurosci Res (2016) ;113: :28–36. |
[216] | Wong EY , Herbert J , Roles of mineralocorticoid and glucocorticoid receptors in the regulation of progenitor proliferation in the adult hippocampus, Eur J Neurosci (2005) ;22: (4):785–92. |
[217] | Moon M , Kim S , Hwang L , Park S , Ghrelin Regulates Hippocampal Neurogenesis in Adult Mice, Endocr J (2009) ;56: (3):525–31. |
[218] | Li E , Chung H , Kim Y , Kim DH , Ryu JH , Sato T , et al., Ghrelin directly stimulates adult hippocampal neurogenesis: implications for learning and memory, Endocr J (2013) ;60: (6):781–9. |
[219] | Chung H , Li E , Kim Y , Kim S , Park S , Multiple signaling pathways mediate ghrelin-induced proliferation of hippocampal neural stem cells, J Endocrinol (2013) ;218: (1):49–59. |
[220] | Chung H , Park S , Ghrelin regulates cell cycle-related gene expression in cultured hippocampal neural stem cells, J Endocrinol (2016) ;230: (2):239–50. |
[221] | Avraham Y , Davidi N , Lassri V , Vorobiev L , Kabesa M , Dayan M , et al., Leptin induces neuroprotection neurogenesis and angiogenesis after stroke, Curr Neurovasc Res (2011) ;8: (4):313–22. |
[222] | Garza JC , Guo M , Zhang W , Lu XY , Leptin increases adult hippocampalneurogenesis in vivo and in vitro, J Biol Chem (2008) ;283: (26):18238–47. |
[223] | Garza JC , Guo M , Zhang W , Lu XY , Leptin restores adult hippocampal neurogenesis in a chronic unpredictable stress model of depression and reverses glucocorticoid-induced inhibition of GSK-3beta/beta-catenin signaling, Mol Psychiatry (2012) ;17: (8):790–808. |
[224] | Perez-Gonzalez R , Antequera D , Vargas T , Spuch C , Bolos M , Carro E , Leptin induces proliferation of neuronal progenitors and neuroprotection in a mouse model of Alzheimer’s disease, J Alzheimers Dis (2011) ;24: (Suppl 2):17–25. |
[225] | Sui Y , Vermeulen R , Hokfelt T , Horne MK , Stanic D , Female mice lacking cholecystokinin 1 receptors have compromised neurogenesis, and fewer dopaminergic cells in the olfactory bulb, Front Cell Neurosci (2013) ;7: :13. |
[226] | Langmesser S , Cerezo-Guisado MI , Lorenzo MJ , Garcia-Marin LJ , Bragado MJ , CCK1 and 2 receptors are expressed in immortalized rat brain neuroblasts: intracellular signals after cholecystokinin stimulation, J Cell Biochem (2007) ;100: (4):851–64. |
[227] | Stanic D , Paratcha G , Ledda F , Herzog H , Kopin AS , Hokfelt T , Peptidergic influences on proliferation, migration, and placement of neural progenitors in the adult mouse forebrain, Proc Natl Acad Sci U S A (2008) ;105: (9):3610–5. |
[228] | Howell OW , Doyle K , Goodman JH , Scharfman HE , Herzog H , Pringle A , et al., Neuropeptide Y stimulates neuronal precursor proliferation in the post-natal and adult dentate gyrus, J Neurochem (2005) ;93: (3):560–70. |
[229] | Brooker GJ , Kalloniatis M , Russo VC , Murphy M , Werther GA , Bartlett PF , Endogenous IGF-1 regulates the neuronal differentiation of adult stem cells, J Neurosci Res (2000) ;59: (3):332–41. |
[230] | Niu X , Zhao Y , Yang N , Zhao X , Zhang W , Bai X , et al., Proteasome activation by insulin-like growth factor-1/nuclear factor erythroid 2-related factor 2 signaling promotes exercise-induced neurogenesis, Stem Cells 2019. |
[231] | Aberg MA , Aberg ND , Hedbacker H , Oscarsson J , Eriksson PS , Peripheral infusion of IGF-I selectively induces neurogenesis in the adult rat hippocampus, J Neurosci (2000) ;20: (8):2896–903. |
[232] | Aberg MA , Aberg ND , Palmer TD , Alborn AM , Carlsson-Skwirut C , Bang P , et al., IGF-I has a direct proliferative effect in adult hippocampal progenitor cells, Mol Cell Neurosci (2003) ;24: (1):23–40. |
[233] | Faivre E , Gault VA , Thorens B , Holscher C , Glucose-dependent insulinotropic polypeptide receptor knockout mice are impaired in learning, synaptic plasticity, and neurogenesis, J Neurophysiol (2011) ;105: (4):1574–80. |
[234] | Forte N , Boccella S , Tunisi L , Fernandez-Rilo AC , Imperatore R , Iannotti FA , et al., Orexin-A and endocannabinoids are involved in obesity-associated alteration of hippocampal neurogenesis, plasticity, and episodic memory in mice, Nat Commun (2021) ;12: (1):6137. |
[235] | Khosravi Y , Seow SW , Amoyo AA , Chiow KH , Tan TL , Wong WY , et al., Helicobacter pylori infection can affect energy modulating hormones and body weight in germ free mice, Sci Rep (2015) ;5: :8731. |
[236] | Duca FA , Swartz TD , Sakar Y , Covasa M , Increased oral detection, but decreased intestinal signaling for fats in mice lacking gut microbiota, PLoS One (2012) ;7: (6):e39748. |
[237] | Schele E , Grahnemo L , Anesten F , Hallen A , Backhed F , Jansson JO , The gut microbiota reduces leptin sensitivity and the expression of the obesity-suppressing neuropeptides proglucagon (Gcg) and brain-derived neurotrophic factor (Bdnf) in the central nervous system, Endocrinology (2013) ;154: (10):3643–51. |
[238] | Pen J , Welling GW , Influence of the microbial flora on the amount of CCK8- and secretin21-27-like immunoreactivity in the intestinal tract of mice, Comp Biochem Physiol B (1983) ;76: (3):585–9. |
[239] | Woods SE , Leonard MR , Hayden JA , Brophy MB , Bernert KR , Lavoie B , et al., Impaired cholecystokinin-induced gallbladder emptying incriminated in spontaneous “black” pigment gallstone formation in germfree Swiss Webster mice, Am J Physiol Gastrointest Liver Physiol (2015) ;308: (4):G335–49. |
[240] | Leeuwendaal NK , Cryan JF , Schellekens H , Gut peptides and the microbiome: focus on ghrelin, Curr Opin Endocrinol Diabetes Obes (2021) ;28: (2):243–52. |
[241] | Lichtenwalner RJ , Forbes ME , Bennett SA , Lynch CD , Sonntag WE , Riddle DR , Intracerebroventricular infusion of insulin-like growth factor-I ameliorates the age-related decline in hippocampal neurogenesis, Neuroscience (2001) ;107: (4):603–13. |
[242] | Pardo J , Abba MC , Lacunza E , Ogundele OM , Paiva I , Morel GR , et al., IGF-I Gene Therapy in Aging Rats Modulates Hippocampal Genes Relevant to Memory Function, J Gerontol A Biol Sci Med Sci (2018) ;73: (4):459–67. |
[243] | Morais LH , Golubeva AV , Moloney GM , Moya-Perez A , Ventura-Silva AP , Arboleya S , et al., Enduring Behavioral Effects Induced by Birth by Caesarean Section in the Mouse, Curr Biol (2020) ;30: (19):3761–74 e6. |
[244] | Al Nabhani Z , Dulauroy S , Marques R , Cousu C , Al Bounny S , Dejardin F , et al., A Weaning Reaction to Microbiota Is Required for Resistance to Immunopathologies in the Adult, Immunity (2019) ;50: (5):1276–88 e5. |
[245] | Vogt NM , Kerby RL , Dill-McFarland KA , Harding SJ , Merluzzi AP , Johnson SC , et al., Gut microbiome alterations in Alzheimer’s disease, Sci Rep (2017) ;7: (1):13537. |
[246] | Dohm-Hansen S , Donoso F , Lucassen PJ , Clarke G , Nolan YM , The gut microbiome and adult hippocampal neurogenesis: A new focal point for epilepsy? Neurobiol Dis (2022) ;170: :105746. |
[247] | Toda T , Parylak SL , Linker SB , Gage FH , The role of adult hippocampal neurogenesis in brain health and disease, Mol Psychiatry (2019) ;24: (1):67–87. |
[248] | Park JC , Im SH , Of men in mice: the development and application of a humanized gnotobiotic mouse model for microbiome therapeutics, Exp Mol Med (2020) ;52: (9):1383–96. |
[249] | Krych L , Hansen CH , Hansen AK , van den Berg FW , Nielsen DS , Quantitatively different, yet qualitatively alike: a meta-analysis of the mouse core gut microbiome with a view towards the human gut microbiome, PLoS One (2013) ;8: (5):e62578. |
[250] | TaupinP. BrdU immunohistochemistry for studying adult neurogenesis: paradigms, pitfalls, limitations, and validation. Brain Res Rev. (2007) ;53: (1):198–214. |