Diffusion tensor-MRI detects exercise-induced neuroplasticity in the hippocampal microstructure in mice
Abstract
Background:
Despite considerable research on exercise-induced neuroplasticity in the brain, a major ongoing challenge in translating findings from animal studies to humans is that clinical and preclinical settings employ very different techniques.
Objective:
Here we aim to bridge this divide by using diffusion tensor imaging MRI (DTI), an advanced imaging technique commonly applied in human studies, in a longitudinal exercise study with mice.
Methods:
Wild-type mice were exercised using voluntary free-wheel running, and MRI scans were at baseline and after four weeks and nine weeks of running.
Results:
Both hippocampal volume and fractional anisotropy, a surrogate for microstructural directionality, significantly increased with exercise. In addition, exercise levels correlated with effect size. Histological analysis showed more PDGFRα+ oligodendrocyte precursor cells in the corpus callosum of running mice.
Conclusions:
These results provide compelling in vivo support for the concept that similar adaptive changes occur in the brains of mice and humans in response to exercise.
INTRODUCTION
Physical activity’s capacity to preserve cognitive function during aging and dementia are well documented in animal models and human subjects [1–3], but the mechanisms underlying these benefits are less well understood. Translating research results from animals to humans is hampered by the fact that preclinical and clinical contexts use quite disparate approaches. Mouse studies usually rely on analyzing tissues samples collected post mortem, whereas human studies must generally employ non-invasive neuroimaging techniques. Rodent models investigating neuroplasticity in the hippocampus show that exercise increases dendritic complexity; raises the number of dendritic spines; enhances synaptic plasticity; improves de novo neurogenesis, angiogenesis, and cerebral blood volume in the dentate gyrus; and elevates oligodendrocyte precursor proliferation [4–10]. In humans, exercise increases both hippocampal volume and cerebral blood flow to the hippocampus [7, 11–13]. higher physical activity levels are associated with improved global white matter (WM) outcomes, including expanded WM volume, smaller WM lesions, and better WM microstructure [14]. Of note, to the best of our knowledge there are currently no studies that have investigated WM outcomes specifically in the hippocampus in humans.An important outstanding question is how animal studies can include noninvasive neuroimaging outcomes to link changes in biological substrates to their testable forms in humans. We address this question using the advanced imaging method diffusion tensor imaging MRI (in vivo DTI), which is usually employed in human studies, in a longitudinal study design with running exercise intervention. DTI is a neuroimaging technique that can evaluate the orientation and anisotropy of the brain’s white matter tracts (for a review of the physical concepts underlying DTI, see [15]). The literature regarding humans generally accepts DTI as a measure of “white matter integrity” and “white matter microstructure”, because water molecule diffusion is restricted by microstructural barriers, such as the myelin sheaths around axons. This phenomenon is generally thought to allow the visualization of large fiber bundles [16, 17]. We selected in vivo imaging in order to image the mice at multiple time-points in a longitudinal/interventional study design. This approach holds great value, as longitudinal/interventional studies with in vivo imaging can (1) mimic human clinical trial design, (2) track changes within the same animal over time, (3) produce/generate no fixation/post-processing artefacts, and (4) reduce mouse numbers via a within-subject (i.e., repeated) design. In addition, follow-up studies using ex vivo imaging can delineate the molecular mechanisms that underpin microstructural changes.In this proof-of-concept-study, we present in vivo evidence that exercise has similar structural benefits in mouse and human brains. Our study thus adds to the existing literature on the roles of new myelin and white matter microstructural adaptations in neuroplasticity [18–21]. Future studies will include a DTI study in human subjects to determine whether exercise can improve hippocampal FA in humans and mechanistic studies in mice to delineate the molecular mechanism that underly the microstructural changes.
MATERIALS AND METHODS
Animal studies
All animal procedures were approved by the Institutional Animal Care and Use Committee (IACUC) of the Massachusetts General Hospital (MGH). Wild-type C57BL/6J (Jax stock no. 000664) were obtained from The Jackson Laboratory. Mice were maintained in an SPF environment at an MGH animal facility, where they were kept under 12-h light/12-h dark cycles (7:00 am-7:00 pm) at a constant temperature (22°C). Mice had free access to water and food, specifically a standard diet (Prolab® IsoPro® RMH 3000, Irradiated).
Study design
Male seven-week-old wild-type C57BL/6J mice (n = 8) were weighed, and MRI scans of their brains were performed. Then the mice were placed individually in cages with stainless steel running wheels (Starr Life Sciences). After four weeks and nine weeks, the mice were weighed again and the MRI scans were repeated (n = 8 and n = 7, respectively). The mice were euthanized using isoflurane anesthesia, then perfused with 4% paraformaldehyde (PFA), and their brains were collected. In addition, brains were collected from age- and sex-matched sedentary control mice (n = 7), which were individually housed in identical cages without a running wheel for the duration of the experiment and euthanized on the same day. Animal #8 died after the MRI scan at four weeks. Running activity was tracked using a revolution counter that collected data every hour (Starr Life Sciences).
MRI mouse procedure
Mice were scanned on a 4.7T preclinical scanner (BioSpec 47/40 USR, Bruker Corporation, Billerica, MA) with a four-channel phased array coil under anesthesia (2% isoflurane) with physiological monitoring including respiratory rate and temperature. Localizers were performed with accompanying T1-weighted (TR = 222 ms, TE = 3.2 ms, flip angle = 40deg, 0.2×0.2×0.75 mm3, FOV = 20 mm×20 mm) and T2-weighted (TR = 2315 ms, TE = 44.3 ms, 0.2×0.2×0.75 mm3, FOV = 20 mm×20 mm) anatomical scans. DTI was performed using a single shot 2D echo planar imaging (TR = 2300 ms, TE = 23.14 ms, NEX = 4 averages, 0.2×0.2×0.75 mm3, FOV = 20 mm×20 mm) with 30 directions and b-value = 1000 s/mm2). Total scan time was ∼45 minutes.
MRI image and data analysis
For each animal across all imaging sessions, the T2-weighted images underwent blinded manual segmentation to estimate hippocampal volume. These hippocampal regions of interest (ROI) were then mapped to the DTI b0 space via conventional affine registration with mutual information (FLIRT) for subject-specific DTI parameter estimation [22]. DTI parameter mapping used conventional log linear least squares fitting to fit the self-diffusion tensor [23], in which mean diffusivity (MD) and fractional anisotropy (FA) were calculated from the eigenvalue decomposition of the tensor. Resulting eigenvalues (λ1 > λ2 > λ3) were used to also calculate axial (λa = λ1) and radial (λr = (λ2 + λ3) / 2) diffusivities.
Immunofluorescence
Mouse brains were fixed for 24 hours in 4% PFA in phosphate-buffered saline (PBS) at 4°C, then stored in 30% sucrose at 4°C. Coronal sections (–1.70 to – 2.30 bregma) with a thickness of 35μm were used for immunohistochemistry. For MBP staining, brain sections were incubated overnight with anti-MBP antibody (1:100, ab7349, Abcam). After washing with PBS, the brain sections were then incubated with secondary antibody (1:500; Jackson Immunoresearch Laboratories) for 1 hour at room temperature. Next, the sections were covered with ProLong Gold mountant with DAPI (ThermoFisher). Immunostaining was analyzed with a fluorescence microscope (Nikon) interfaced with a digital charge-coupled device camera and an image analysis system. For PDGFRα staining, brain sections were incubated overnight with anti-PDGFRα antibody (1:100, AF1062, R&D). After washing with TBS, the brain sections were incubated with secondary antibody (1:500; Jackson Immunoresearch Laboratories) for 1 hour at room temperature. Nuclei were labeled with DAPI (1:5000, Invitrogen). The sections were then covered with Fluoromount-G mounting media (Southern Biotech). Immunostaining was analyzed fluorescence microscope (Axio Image A2, Zeiss) interfaced with a digital charge-coupled device camera and an image analysis system. The images were analyzed using ImageJ. Regions of interest (ROIs) for different (peri-) hippocampal regions were defined as indicated on representative images. The average pixel intensity from each ROI (4 images (L/R x 2 sections) for each animal) was used as a measure of myelin density. PDGFRα+ cells were counted in each ROI (4 images (L/R x 2 sections)) using thresholding and expressed as number of cells per mm2.
Experimental design and statistical analyses
Data analysis was performed using GraphPad Prism 7 software. Unpaired and paired two-tailed Student’s t-tests were performed to compare two groups. One-way repeated-measures ANOVA with Tukey test post hoc test where appropriate was used to analyze three groups. Outcomes in MRI measurements (volume, MD, and FA) and histological analysis (MBP and number of PDGFR α+ cells) were correlated with exercise performance over time using a Pearson correlation (two-tailed), and a linear regression analysis was conducted. To be able to perform repeated-measures one-way ANOVA for the MRI measurements with every time point, missing values (animal #8 died after the MRI scan at four weeks), were substituted using a mean substitution. To be able to perform repeated-measures one-way ANOVA for the running activity, missing values (the sensor for animal #2 did not record for days 24, 25, and 26) were substituted using a mean substitution. Significance was assigned to differences with a p-value less than 0.05. n.s.=not significant. Pooled data are presented as mean ± SEM.
RESULTS
Longitudinal study design to assess exercise-induced changes in brain microstructure using diffusion tensor-magnetic resonance imaging (DTI)
Recent advances in MRI for neuroimaging applications have greatly expanded the range of biological processes that can be investigated. In this longitudinal study, we used DTI to assess exercise-induced changes in the hippocampal microstructure of wild-type mice. We chose a longitudinal design to take advantage of neuroimaging’s capacity to track changes over time within a single subject, instead comparing two groups, to test whether exercise-induced changes in neuroplasticity can be monitored in vivo and to replicate a common approach in human studies (Fig. 1A). Seven-week-old wild-type C57BL/6J mice were singly housed with access to a running wheel for nine weeks. At base line (0 weeks), 4 weeks, and 9 weeks, the mice underwent a DTI scan, with anesthesia, using a 4.7T preclinical scanner (BioSpec 47/40 USR, Bruker Corporation) with a four-channel phased array coil. These time frames were chosen because they had previously been shown in mice to enhance adult hippocampal neurogenesis, hippocampal synaptic plasticity, and spatial learning and memory [6]. To confirm mice sufficiently exercised, body weight and running activity were monitored (Fig. 1B-D). The mice ran 7.5 ± 0.66 km/d at four weeks and 6.1 ± 0.62 km/d at nine weeks, which is on par with previously reported running activity for mice [6].
Fig. 1
Longitudinal study design to assess exercise-induced changes in brain microstructure using diffusion tensor-magnetic resonance imaging (DTI). (A) Schematic of running exercise protocol. Mice brains were scanned by MRI at baseline (0 weeks), 4 weeks, and 9 weeks. (B) Body weight of mice at 0, 4, and 9 weeks. Data are shown as g, expressed as mean ± SEM. (C) Running per day and (D) Total running over the nine-week time period were measured by a sensor attached to the running wheel. Data were obtained as revolutions per minute and then converted to km. Data are expressed as mean ± SEM.
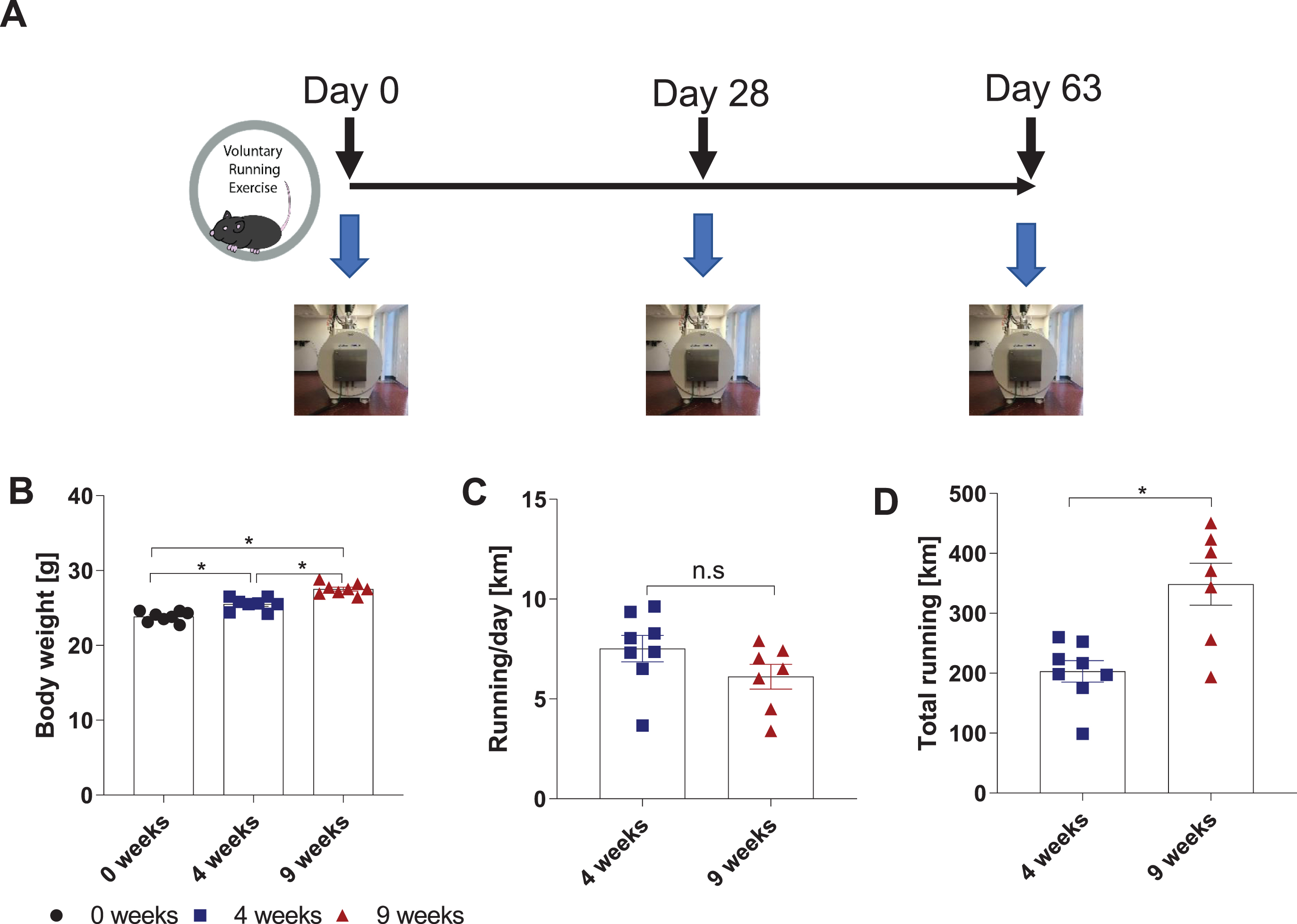
Voluntary running changes mouse brain MRI measurements
The hippocampus, an area of the brain closely involved in declarative memory and spatial awareness [24], is compromised early in aging and neurodegenerative diseases [25, 26]; furthermore, the hippocampal formation is a key recipient of exercise’s beneficial effects on the brain [1]. For these reasons, our study focused on changes in the hippocampal formation. T1-weighted (T1w) and T2-weighted (T2w) MRI was used to locate the anatomical region of interest (ROI) and to calculate the hippocampal volume. DTI was performed to measure mean diffusivity (MD) and fractional anisotropy (FA) in the ROI. MD measures the overall magnitude of diffusion motility of water and therefore, MD is expected to be lower in more densely packed tissue, which restricts diffusion motility, and to be higher when less structure is present, for example in edema. FA is a surrogate for microstructural directionality: the higher the FA, the more directionality is present in the microstructure. Representative images used to analyze hippocampal volume, FA, and MD are shown in Fig. 2A-C. Quantification of the MRI outcomes are shown in Fig. 2D-F. All anatomical measurements taken by MRI are summarized in Table 1.Running exercise increased the hippocampal volume over time (F (2,14) = 6.76, p = 0.009, one-way ANOVA, n = 8 mice; 0 weeks vs. 4 weeks: n.s., 0 weeks vs 9 weeks: p = 0.007, 4 weeks vs 9 weeks: n.s., Tukey test). At base line, mean hippocampal volume measured 1.84 ± 0.06 mm3. After four weeks of exercise, the hippocampal volume rose to 1.92 ± 0.10 mm3, but the rise was not statistically significant. However, nine weeks of running significantly increased the hippocampal volume to 2.10 ± 0.10 mm3 (p = 0.009 compared to baseline) (Fig. 2D). More importantly, running exercise significantly improved the hippocampal microstructure in terms of directionality/fractional anisotropy (F (2,14) = 11.43, p = 0.001, one-way ANOVA; 0 weeks vs. 4 weeks: p = 0.007, 0 weeks vs. 9 weeks: p = 0.001, 4 weeks vs. 9 weeks: n.s., Tukey test). The hippocampal FA rose significantly from 0.35 ± 0.015 at 0 weeks to 0.39 ± 0.014 AU at four weeks (p = 0.007) and to 0.40 ± 0.016 AU at nine weeks (p = 0.001), respectively (Fig. 2E). However, mean diffusivity in the hippocampus did not significantly change with running exercise (F (2,14) = 0.15, p = 0.856, one-way ANOVA) (Fig. 2F). Of note, because the hippocampal volume and the FA data sets each contained a value that was further away from the mean than the rest, we performed the ROUT method (Q-value = 1%) to identify outliers. No significant outliers were found in either the hippocampal volume or the FA data set.
Fig. 2
Voluntary running induces changes in hippocampal volume and microstructure as measured by DTI. Representative MRI images of hippocampal volume (A), fractional anisotropy (B), and mean diffusivity (C). The red box in (A) outlines the area of the hippocampal formation. The white area in (B) denotes the region of interest: the hippocampal formation, including CA1, CA2, CA3, and DG. The various colors in (B) represent orientation of the microstructure in space: red is lateral (from left to right), green is superior to inferior, and blue is anterior to posterior. Note the corpus callosum in red is traveling from left to right. (D) Hippocampal volume is shown in mm3. Data are expressed as mean ± SEM. *p < 0.05 using repeated-measures one-way ANOVA. (E) Hippocampal fractional anisotropy (FA) and (F) mean diffusivity (MD) are depicted as arbitrary units (AU). Data are expressed as mean ± SEM. *p < 0.001 and #p < 0.01 are compared to baseline scan at 0 weeks using repeated-measures one-way ANOVA. n.s. = not significant, p > 0.05.
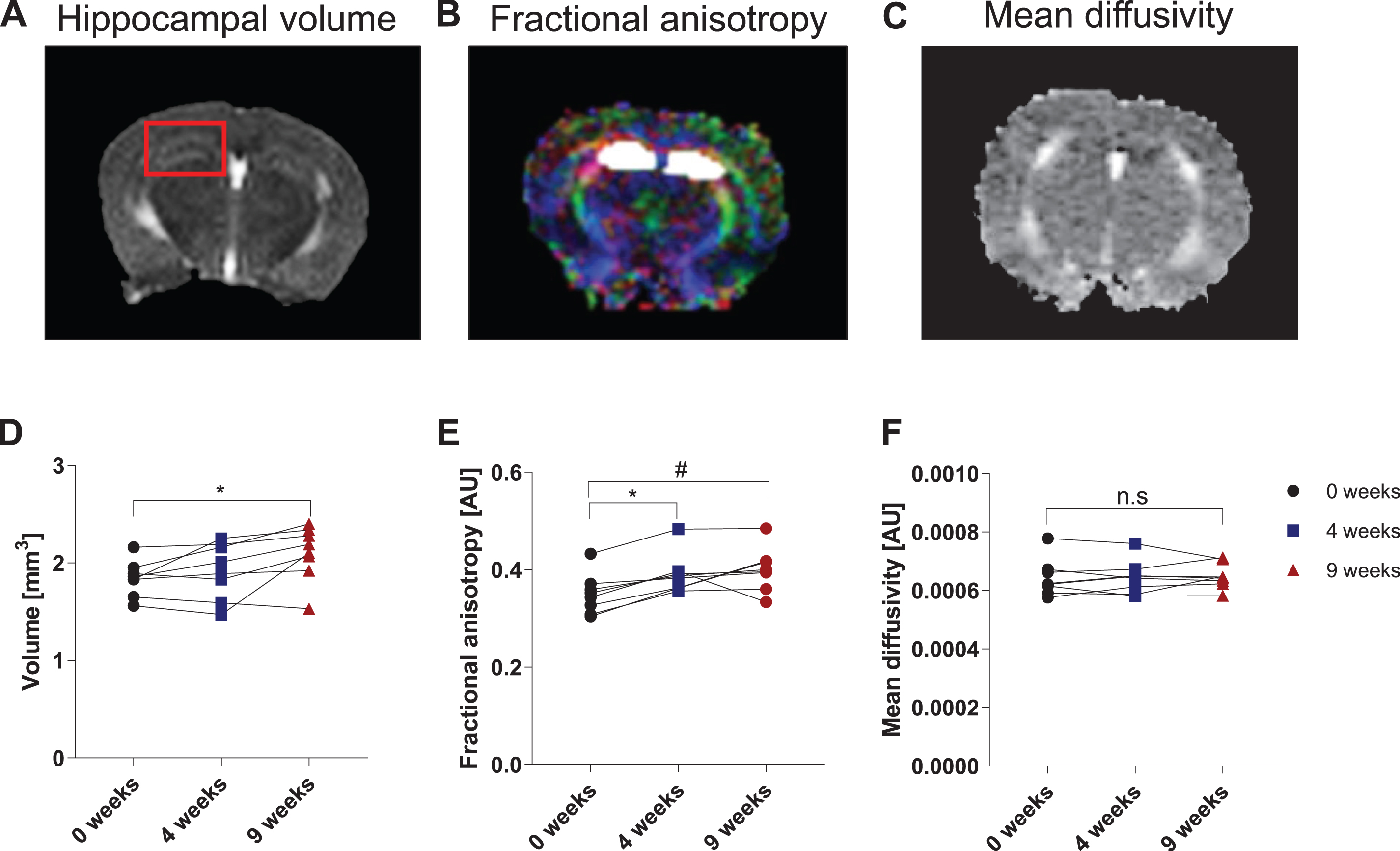
Table 1
MRI measurements of the hippocampal formation at zero, four, and nine weeks of running exercise. Mouse hippocampal volume was measured in mm3; MD (mean diffusivity), FA (fractional anisotropy), axial diffusivity (AD), and radial diffusivity (RD) were measured in arbitrary units. SEM =Standard Error of Mean, MS = Mean substitution
0 week | 4 weeks | 9 weeks | ||||||||||||||||
Animal | Voxels | Volume | MD | FA | AD | RD | Voxels | Volume | MD | FA | AD | RD | Voxels | Volume | MD | FA | AD | RD |
1 | 72 | 2.16 | 0.0006 | 0.3083 | 0.0007 | 0.0006 | 73 | 2.19 | 0.0006 | 0.3560 | 0.0007 | 0.0005 | 76 | 2.28 | 0.0006 | 0.3602 | 0.0007 | 0.0005 |
2 | 61 | 1.83 | 0.0006 | 0.3273 | 0.0008 | 0.0005 | 75 | 2.25 | 0.0006 | 0.3625 | 0.0008 | 0.0006 | 78 | 2.34 | 0.0006 | 0.4175 | 0.0008 | 0.0006 |
3 | 62 | 1.86 | 0.0006 | 0.3040 | 0.0008 | 0.0005 | 67 | 2.01 | 0.0006 | 0.3620 | 0.0007 | 0.0006 | 73 | 2.19 | 0.0006 | 0.4157 | 0.0008 | 0.0005 |
4 | 65 | 1.95 | 0.0006 | 0.4329 | 0.0007 | 0.0006 | 72 | 2.16 | 0.0007 | 0.4831 | 0.0008 | 0.0006 | 80 | 2.4 | 0.0006 | 0.4845 | 0.0008 | 0.0005 |
5 | 55 | 1.65 | 0.0007 | 0.3432 | 0.0008 | 0.0006 | 53 | 1.59 | 0.0007 | 0.3967 | 0.0009 | 0.0006 | 51 | 1.53 | 0.0007 | 0.3335 | 0.0009 | 0.0006 |
6 | 61 | 1.83 | 0.0007 | 0.3712 | 0.0009 | 0.0006 | 63 | 1.89 | 0.0006 | 0.3827 | 0.0009 | 0.0005 | 64 | 1.92 | 0.0006 | 0.3941 | 0.0008 | 0.0005 |
7 | 63 | 1.89 | 0.0008 | 0.3523 | 0.0009 | 0.0007 | 61 | 1.83 | 0.0008 | 0.3912 | 0.0009 | 0.0007 | 69 | 2.07 | 0.0007 | 0.3958 | 0.0009 | 0.0006 |
8 | 52 | 1.56 | 0.0006 | 0.3591 | 0.0008 | 0.0005 | 49 | 1.47 | 0.0006 | 0.3874 | 0.0008 | 0.0005 | MS | 2.1 | 0.0006 | 0.4002 | 0.0008 | 0.0006 |
Mean | 1.84125 | 0.00064 | 0.34979 | 0.00081 | 0.00056 | 1.92375 | 0.00064 | 0.39020 | 0.00080 | 0.00056 | 2.10429 | 0.00065 | 0.40019 | 0.00081 | 0.00057 | |||
SEM | 0.06022 | 0.00002 | 0.01359 | 0.00002 | 0.00002 | 0.09419 | 0.00002 | 0.01339 | 0.00003 | 0.00002 | 0.09238 | 0.00001 | 0.01466 | 0.00002 | 0.00001 |
Voluntary running levels positively correlate with MRI measurements
Next, we asked whether MRI outcomes were correlated with the amount of exercise the mice performed. Total running activity (total running in km) at four weeks did not significantly correlate with any of the MRI parameters (Fig. 3A–C). Interestingly, total running activity after nine weeks was significantly correlated with hippocampal volume (R2 = 0.52, p = 0.045) (Fig. 3D) and microstructure directionality/FA (R2 = 0.85, p = 0.001) (Fig. 3E) but not MD (Fig. 3F).
Fig. 3
Changes in hippocampal volume and microstructure correlate with exercise level. Correlations of hippocampal volume (A), fractional anisotropy (B), and mean diffusivity (C) with the total amount of running at four weeks were calculated using the two-tailed Pearson correlation test. An individual dot represents one mouse. Data are expressed as mean ± SEM. Correlations of hippocampal volume (D), FA (E), and MD (F) with the total amount of running at nine weeks were calculated using the two-tailed Pearson correlation test. An individual dot represents one mouse. Data are expressed as mean ± SEM. Asterisks highlight significant correlations (*p < 0.05).
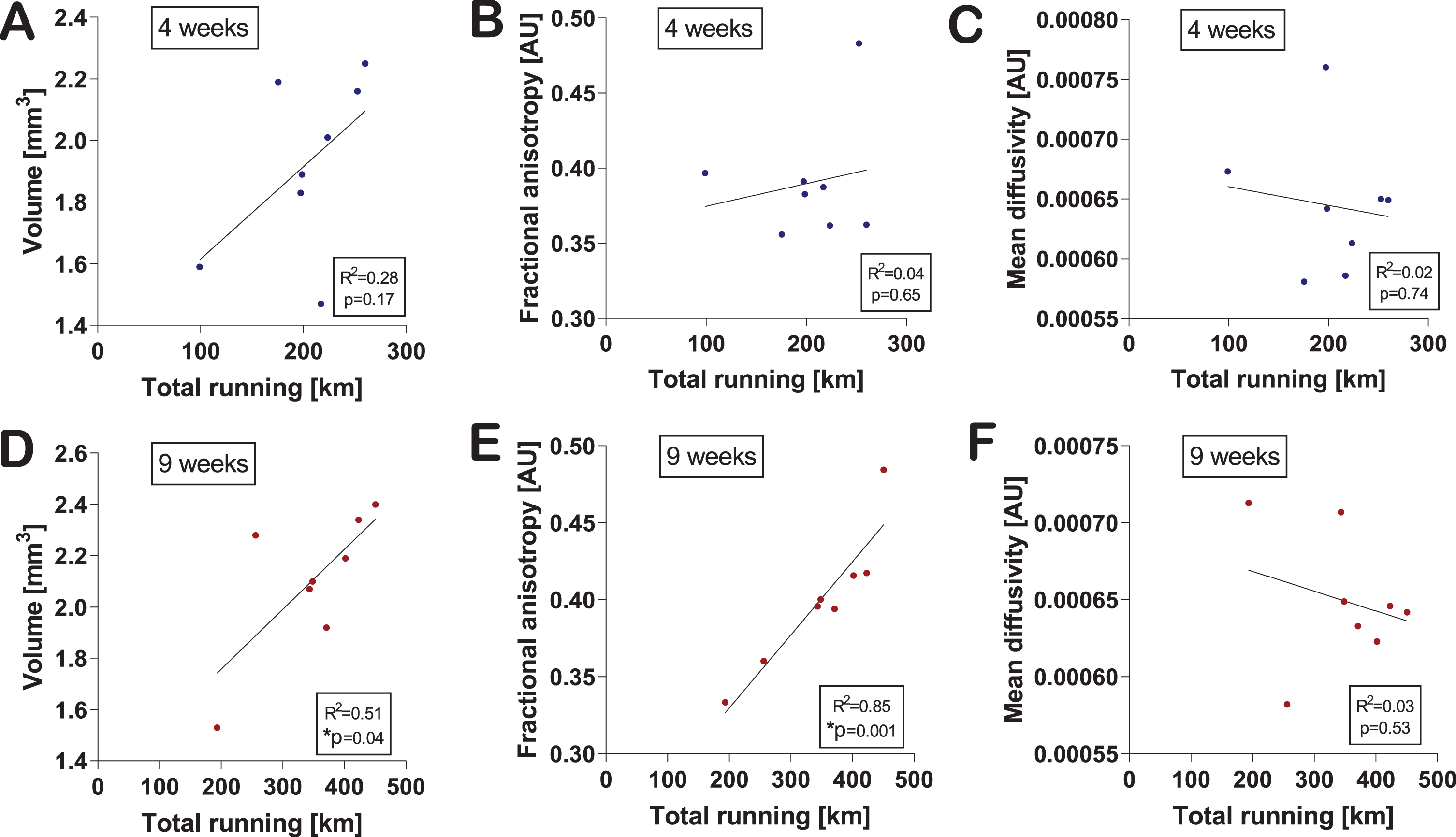
Voluntary running does not affect axial diffusivity or radial diffusivity in the hippocampus
MD is calculated as the mean of the diffusion tensor’s three eigenvalues. Since MD represents an average, it is less sensitive than the individual measurements of axial and radial diffusivity. We therefore decided to analyze hippocampal axial diffusivity (AD) and radial diffusivity (RD) in addition to MD. However, running exercise did not significantly affect either AD or RD (Fig. 4A-B). Similar to the results reported for MD, total running activity (total running in km) after four or nine weeks did not significantly correlate with either AD or RD (Fig. 4C-E). Of note, we tested the data sets for outliers using the ROUT method (Q-value = 1%) as described above, but no outliers were identified.
Fig. 4
Voluntary running does not affect axial diffusivity or radial diffusivity in the hippocampus. Changes in hippocampal axial diffusivity (AD) (A) and radial diffusivity (RD) (B) after four and nine weeks of running. Data are depicted as arbitrary units (AU) and are expressed as mean ± SEM. n.s. = not significant using repeated-measures one-way ANOVA. Correlations of hippocampal AD (C) and RD (D) with the total amount of running at four weeks were calculated using the two-tailed Pearson correlation test. An individual dot represents one mouse. Data are expressed as mean ± SEM. Correlations of hippocampal AD (E) and RD (F) with the total amount of running at nine weeks were calculated using the two-tailed Pearson correlation test. An individual dot represents one mouse. Data are expressed as mean ± SEM.
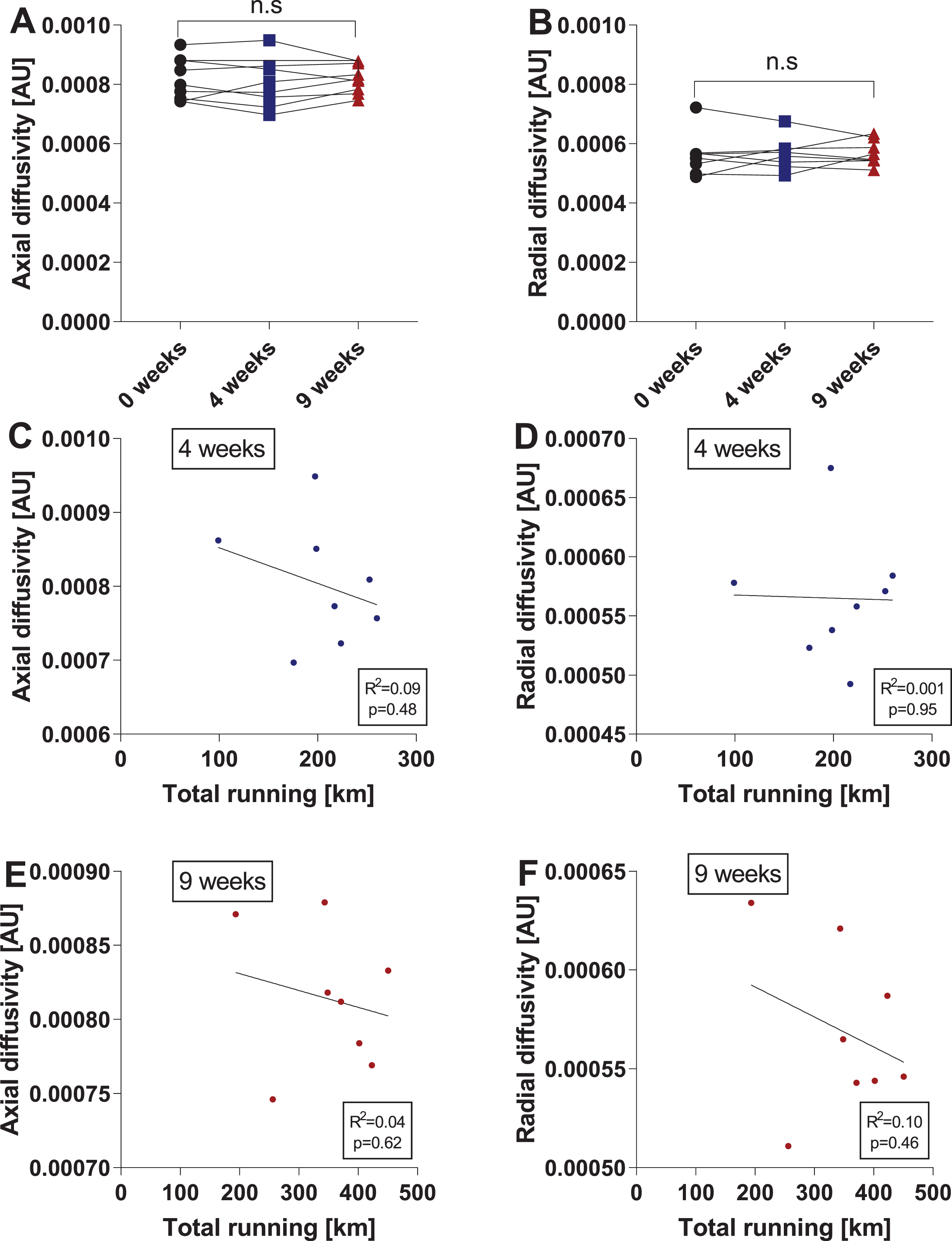
Voluntary running enhances myelination
FA represents diffusion directionality of a population of water molecules. In white matter, restriction in this diffusion has been proposed to depend on axon size and myelination [15]. Accordingly, we performed immunofluorescence (IF) staining for MBP (myelin basic protein), which is a major constituent of the myelin sheath in the brain [19], and for PDGFRα (platelet derived growth factor receptor α), which labels oligodendrocyte precursor cells [27, 28], on hippocampal sections from mice that ran for nine weeks mice and sedentary controls. We first analyzed the corpus callosum because in different disease models, running exercise has been shown to increase myelinization in this brain region [5, 29]. In line with these previous reports, we observed a 23% increase in MBP levels after nine weeks of running, though this was not significant (unpaired two-tailed t-test, p = 0.1616 (Fig. 5A-B), and significantly more PDGFRα+ cells in running mice compared to sedentary controls (unpaired two-tailed t-test, p = 0.0003 (Fig. 5D-E). Both measures were significantly correlated with the total amount of running at nine weeks (R2 = 0.64, p = 0.016 and R2 = 0.80, p = 0.003, respectively) (Fig. 5C, F). These results indicate that our experimental design was sufficient to induce and to detect white matter plasticity. However, the corpus callosum was not part of the ROI for evaluating hippocampal FA (Fig. 2B).Next, we analyzed MBP content in the white matter surrounding the hippocampus (see blue box in Supplemental Figure 1A-B). Running exercise increased MBP content by 14%, though this was not statistically significant (unpaired two-tailed t-test, p = 0.4168) (Supplemental Figure 1C). Interestingly, total running activity after nine weeks was significantly correlated with MBP content (R2 = 0.58, p = 0.03) (Supplemental Figure 1D). The number of PDGFRα+ cells rose by 14% in mice that ran, but this boost was not statistically significant (unpaired two-tailed t-test, p=0.4663) (Supplemental Figure 1E-G). In addition, there was no significant correlation between the changes in histological parameters and total running activity after nine weeks (Supplemental Figure 1H). We also analyzed the dentate gyrus because it displays strong plasticity in response to exercise, including with regard to neurogenesis and angiogenesis (2). Yet neither MBP content nor number of PDGFRα+ cells in the dentate gyrus were significantly affected by running or significantly correlated with running levels (Supplemental Figure 2A-H). Taken together, these data suggest either that our histological analysis did not capture the white matter changes underlying the increase in hippocampal FA produced by running exercise or that the increase in hippocampal FA is a result of changes in hippocampal microstructure directionality caused by something other than enhanced myelination.
Fig. 5
Voluntary running enhances myelination in the corpus callosum. Myelin immunostaining for MBP (myelin basic protein) and PDGFRα (platelet derived growth factor receptor α) in the hippocampus (HC). Representative images of MBP staining of hippocampal sections (A). Scale bar is 500μm. Relative myelin intensity in the corpus callosum (CC) (B) and correlation of nine weeks of total running with myelin intensity in the CC (C). Representative images of PDGFRα staining, a marker for oligodendrocyte precursors, of hippocampal sections (D). Insert depicts 40x magnification with a PDGFRα+ cells. Scale bar is 100μm. Total number of PDGFRα+cell count/mm2 of CC area (G) and correlation of the total amount running in 9 weeks with PDGFRα+cell count/mm2 (H). Data are expressed as mean ± SEM. Asterisks highlight significance (*p < 0.05), n.s. = not significant. Correlations were calculated using the two-tailed Pearson correlation test. An individual dot represents one mouse. The boxes in (A) and (D) mark the regions of interest for image analysis. Cg = cingulate gyrus, cc = corpus callosum, f = fornix.
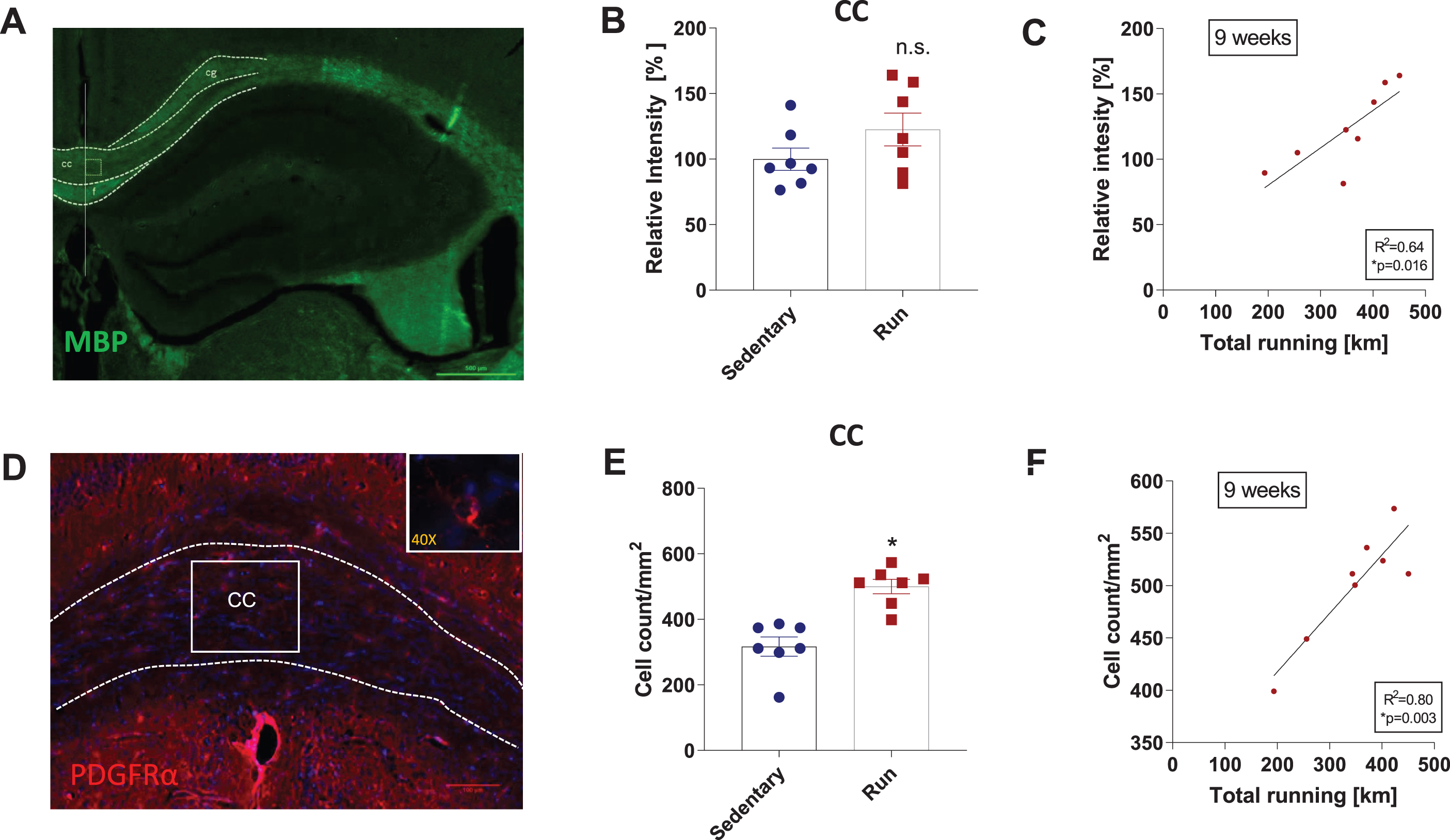
DISCUSSION
Exercise-induced increases in hippocampal volume, measured by MRI, have been previously described in mice [30, 31] and humans [11, 32-35]. In addition, such increases have been correlated with exercise levels in humans [12, 13, 36], suggesting a dose-response effect. Our findings on hippocampal volume are in line with these results, indicating that our study design was sufficient to produce and evaluate exercise-induced neuroplasticity in the hippocampus. DTI studies in humans have shown that exercise raises FA in the brain [37-40] and that a higher-percentile change in fitness was associated with significantly increased temporal FA [41]. Although, to the best of our knowledge, no study has yet assessed exercise-induced changes in hippocampal FA in humans. Our study is the first to use (in vivo) DTI to investigate the effects of running – i.e. aerobic exercise – on hippocampal microstructure in mice. In accordance with the human data, we observed that exercised mice had elevated microstructural directionality (FA) and that this increase was positively correlated with exercise levels. These result are important because a.) it demonstrates that we can use a longitudinal/interventional study design to investigate exercise-induced hippocampal microstructural plasticity in mice and b.) it confirms the relevance our model to the human condition. Therefore, we can perform the necessary mechanistic studies that cannot be executed in humans in this model.Two studies have used ex vivo DTI to evaluate running exercise’s effects on rat disease models. One such study using a short-term (4 weeks) treadmill exercise protocol in Disc1 svΔ2 rats, a genetic model of schizophrenia, reported significant differences in the neural microstructures – specifically the neocortex, basal ganglia, corpus callosum, and external capsule, not the hippocampus – of sedentary versus exercised Disc1 sv Δ2 animals but observed no such differences between sedentary and exercised control animals [42]. The other study employed ex vivo DTI to investigate the neuroprotective effect of running exercise in a cranial radiation therapy rat model and found that exercise increased whole brain volume, the number of fibers in the entire brain, and global connectomics. However, this study did not demonstrate such effects on hippocampal volume or hippocampal FA [43]. There are several differences in the study design that could explain the difference in observed outcomes: the exercise regimen (forced-treadmill running vs free-wheel running), the models organism (mice vs rats), the use of in vivo vs ex vivo DTI, and, lastly, the time-point of performing the DTI. In both studies, DTI was performed not immediately after the exercise intervention, as it was in our study, but rather 9–12 days and twelve months later, respectively. This is important since it is unknow for how long after ending the exercise intervention, the microstructural changes persists.White matter plasticity is important for learning but its role in running excise-induced neuroplasticity is less well-explored. DTI has been successfully used to show white matter neuroplasticity in complex motor learning in human behaviors such as juggling [18], piano playing [44], or visuomotor skill training [20]. Similar motor learning effects have been observed in rodents using rotarod [45], a prehension task [19], and a unilateral reaching movement task [21]. In addition, spatial learning and memory can induce white matter neuroplasticity in rodents and humans [46, 47]. However, while both motor learning and free-wheel running are forms of exercise and therefore induce neuroplasticity, these exercise types and their neurobiological effects are distinct. Motor learning comprises the processes aimed at learning and refining new skills by practicing them and induces neuroplasticity in areas important for motor function and learning/memory [48]. Though running on an evenly spaced wheel is not very challenging to the motor system, running elicits major adaptive responses in the musculoskeletal, cardiovascular, and metabolic systems [49]. Interestingly, while it does not present a (major) cognitive challenge or learning task, running does improve learning and memory [1–3]. Running has also been shown to be the neurogenic and neurotrophic stimulus in environmental enrichment [50, 51]. Therefore, additional investigations into the basic biology of running-exercise induced-neuroplasticity are important.We observed no changes in hippocampal MD, AD, or RD with running exercise. These results are similar to human studies reporting no significant post-exercise shifts in MD either globally [37], related to specific white matter tracts [39, 52], or in hippocampal MD levels [53]. In contrast to FA, which solely measures directional anisotropy and is affected by axon myelination, MD also measures unrestricted diffusivity, which is mainly changed with severe neuropathology associated with demyelination or axonal injury, such as multiple sclerosis or stroke [54–56]. Of note, one recent study in older adults connected changes in fitness to changes in hippocampal MD [57]. Therefore, it would be interesting to test whether in aged mice running exercise would modify hippocampal MD.Whereas previous studies have provided evidence that elevated perfusion/angiogenesis and neurogenesis in the hippocampus contribute to the increase in volume [7, 58, 59], identifying the underlying cellular and molecular correlates to changes in FA will be much more challenging. FA represents a water molecule population’s diffusion directionality. In white matter, on axon size and myelination have been proposed to cause restriction in this diffusion [15]. Myelination and oligodendrogenesis play well understood roles in sensory-motor learning, and oligodendrogenesis (and therefore myelination) was recently revealed to be an important contributor to spatial memory consolidation [28, 60–63] (for more in-depth reviews, please see [64, 65]). Some studies indicating that learning elevates hippocampal FA also noted effects on MBP, a main component of the myelin sheath, and GAP-43, a marker for axon remodeling [19, 46]. Exercise has also been shown to raise oligodendrocyte precursor proliferation in mouse models [4, 5], and a clinical study suggested myelination of the right parahippocampal cingulum is associated with physical activity in young healthy adults [66]. These results align with our findings, namely that running exercise increased the number of PDGFRα+ oligodendroctyte precursor cells in the corpus callosum. However, none of these studies addresses changes in axon directionality, a task that would require very sophisticated 3D imaging techniques, nor did they demonstrate causality. In the present work, the corpus callosum was not part of the ROI for evaluating hippocampal FA, and, interestingly, in the areas that were part of the ROI in which we investigated MBP levels and PDGFRα+ oligodendrocyte precursor numbers, we did not detect any significant changes after nine weeks of running exercise. It may be that the white matter changes underlying the exercise-induced hippocampal FA increase evaded our histological analysis. Indeed, electron microscopy would be required to measure axon size, lightsheet miscroscopy to assess myelinated fiber directionality. Alternatively, it is possible that elevated hippocampal FA after running exercise is the result of directional variations/alterations that are not due to enhanced myelination, especially since DTI is only an indirect (and non-specific) measure for myelin [16, 67]. Research in humans often employs DWI as a measure of “white matter integrity” and “white matter microstructure” because water diffusion is/can be restricted by microstructures such as the myelin sheaths around axons. Generally, this approach is an accepted way to investigate/characterize large fiber bundles, although the literature on humans also includes substantial misinterpretation, as since myelination only provides indirect interpretation, and some studies present alternate interpretations [16, 17]. Additionally, recent work indicates that grey matter changes, such as gliosis, can also affect DWI measurements [17]. The underlying cellular and molecular correlates of the exercised-induced changes in hippocampal FA will therefore be identified in future studies.
Study limitations
While there is reasonable evidence that exercise mainly affects the dentate gyrus within the hippocampal formation, our study’s spatial resolution precludes subfield analysis. To achieve such spatial resolution with conventional methods, we would need 0.1 mm isotopic resolution to resolve the dentate gyrus, which is 250 um thick, and at least ∼30 times longer scan time [68]. This limited spatial resolution also prevented evaluation of connections between the hippocampus and the cortex that are modulated by exercise [9, 69]. To obtain a data set with sufficient resolution will require a separate study with either much longer scan times per animal (>2 hrs) or an ex vivo protocol.This proof-of-concept study provides strong in vivo evidence that similar adaptive microstructural changes occur in the hippocampus of mice and humans in response to running exercise. In addition, our study adds to the existing literature on the role of new myelin and white matter microstructural adaptations in neuroplasticity [18–21].
FUNDING
C.D.W. was supported by the NIH (NS087096, AG062904-01); the Cure Alzheimer’s Fund (CAF); an Alzheimer’s Association Research Grant (AARG); the Hassenfeld Clinical Scholar Award; and the Claflin Distinguished Scholar Award. M.R.I. was supported by a Harvard Brain Science Initiative Young Scientist Travel Award. C.T.N. was supported by the NIH (EB024701), the MGH Spark Award, and the Hassenfeld Family Foundation.
CONFLICT OF INTEREST STATEMENT
The authors have no conflicts to report.
AUTHOR CONTRIBUTIONS
M.R.I., C.T.N., and C.D.W. planned the experiments and wrote the paper. M.R.I., K.S., B.C.D., C.T.N., and C.D.W. analyzed and interpreted the data. M.R.I., S.V., and Y.I.C. performed the running and MRI experiments. M.R.I., R.L., H.T., E.H., and K.A. performed and analyzed the histological experiments.
ACKNOWLEDGMENTS
We would to thank the MGH-Harvard Catalyst Biostatistics Program and the Division of Clinical Research at MGH, and specifically Dr. Hang Lee, for their support with the biostatistical analysis. This work was conducted with support from Harvard Catalyst | The Harvard Clinical and Translational Science Center (National Center for Advancing Translational Sciences, National Institutes of Health Award UL 1TR002541) and financial contributions from Harvard University and its affiliated academic healthcare centers. The content is solely the responsibility of the authors and does not necessarily represent the official views of Harvard Catalyst, Harvard University and its affiliated academic healthcare centers, or the National Institutes of Health.
SUPPLEMENTARY MATERIAL
[1] The supplementary material is available in the electronic version of this article: http://dx.doi.org/10.3233/BPL-190090.
REFERENCES
[1] | Düzel E , van Praag H , Sendtner M . Can physical exercise in old age improve memory and hippocampal function? Brain: A Journal of Neurology. (2016) ;139: (Pt 3):662–73. |
[2] | Cooper C , Moon HY , van Praag H . On the Run for Hippocampal Plasticity. Cold Spring Harbor Perspectives in Medicine. (2018) ;8: (4). |
[3] | Bherer L , Erickson KI , Liu-Ambrose T . A review of the effects of physical activity and exercise on cognitive and brain functions in older adults. Journal of Aging Research. (2013) ;2013: :657508. |
[4] | Matsumoto Y , Tsunekawa Y , Nomura T , Suto F , Matsumata M , Tsuchiya S , et al. Differential proliferation rhythm of neural progenitor and oligodendrocyte precursor cells in the young adult hippocampus. PloS One. (2011) ;6: (11):e27628–e. |
[5] | Ohtomo R , Kinoshita K , Ohtomo G , Takase H , Hamanaka G , Washida K , et al. Treadmill Exercise Suppresses Cognitive Decline and Increases White Matter Oligodendrocyte Precursor Cells in a Mouse Model of Prolonged Cerebral Hypoperfusion. Translational stroke research. 2019. |
[6] | van Praag H , Christie BR , Sejnowski TJ , Gage FH . Running enhances neurogenesis, learning, and long-term potentiation in mice. Proceedings of the National Academy of Sciences of the United States of America. (1999) ;96: (23):13427–31. |
[7] | Pereira AC , Huddleston DE , Brickman AM , Sosunov AA , Hen R , McKhann GM , et al. An in vivo correlate of exercise-induced neurogenesis in the adult dentate gyrus. Proceedings of the National Academy of Sciences. (2007) ;104: (13):5638–43. |
[8] | Eadie BD , Redila VA , Christie BR . Voluntary exercise alters the cytoarchitecture of the adult dentate gyrus by increasing cellular proliferation, dendritic complexity, and spine density. The Journal of Comparative Neurology. (2005) ;486: (1):39–47. |
[9] | Stranahan AM , Khalil D , Gould E . Running induces widespread structural alterations in the hippocampus and entorhinal cortex. Hippocampus. (2007) ;17: (11):1017–22. |
[10] | Creer DJ , Romberg C , Saksida LM , van Praag H , Bussey TJ . Running enhances spatial pattern separation in mice. Proceedings of the National Academy of Sciences. (2010) ;107: (5):2367–72. |
[11] | Erickson KI , Voss MW , Prakash RS , Basak C , Szabo A , Chaddock L , et al. Exercise training increases size of hippocampus and improves memory. Proceedings of the National Academy of Sciences of the United States of America. (2011) ;108: (7):3017–22. |
[12] | Maass A , Duzel S , Goerke M , Becke A , Sobieray U , Neumann K , et al. Vascular hippocampal plasticity after aerobic exercise in older adults. Mol Psychiatry. (2015) ;20: (5):585–93. |
[13] | Nauer RK , Dunned MF , Sterna CE , Storere TW , K. S. Improving fitness increases dentate gyrus/CA3 volume in the hippocampal head and enhances memory in young adults. Hippocampus. 2019; In press. |
[14] | Sexton CE , Betts JF , Demnitz N , Dawes H , Ebmeier KP , Johansen-Berg H . A systematic review of MRI studies examining the relationship between physical fitness and activity and the white matter of the ageing brain. NeuroImage. (2016) ;131: :81–90. |
[15] | Le Bihan D . Looking into the functional architecture of the brain with diffusion MRI. Nature Reviews Neuroscience. (2003) ;4: (6):469–80. |
[16] | Jones DK , Knösche TR , Turner R . White matter integrity, fiber count, and other fallacies: The do’s and don’ts of diffusion MRI. NeuroImage. (2013) ;73: :239–54. |
[17] | Weston PSJ , Simpson IJA , Ryan NS , Ourselin S , Fox NC . Diffusion imaging changes in grey matter in Alzheimer’s disease: a potential marker of early neurodegeneration. Alzheimer’s Research & Therapy. (2015) ;7: (1):47. |
[18] | Scholz J , Klein MC , Behrens TEJ , Johansen-Berg H . Training induces changes in white-matter architecture. Nature Neuroscience. (2009) ;12: (11):1370–1. |
[19] | Sampaio-Baptista C , Khrapitchev AA , Foxley S , Schlagheck T , Scholz J , Jbabdi S , et al. Motor Skill Learning Induces Changes in White Matter Microstructure and Myelination. The Journal of Neuroscience. (2013) ;33: (50):19499–503. |
[20] | Lakhani B , Borich MR , Jackson JN , Wadden KP , Peters S , Villamayor A , et al. Motor Skill Acquisition Promotes Human Brain Myelin Plasticity. Neural plasticity. (2016) ;2016: :7. |
[21] | Badea A , Ng KL , Anderson RJ , Zhang J , Miller MI , O’Brien RJ . Magnetic resonance imaging of mouse brain networks plasticity following motor learning. PloS One. (2019) ;14: (5):e0216596–e. |
[22] | Jenkinson M , Smith S . A global optimisation method for robust affine registration of brain images. Medical Image Analysis. (2001) ;5: (2):143–56. |
[23] | Basser PJ , Pajevic S , Pierpaoli C , Duda J , Aldroubi A . In vivo fiber tractography using DT-MRI data. Magnetic Resonance in Medicine. (2000) ;44: (4):625–32. |
[24] | Eichenbaum H , Yonelinas AP , Ranganath C . The medial temporal lobe and recognition memory. Annual review of neuroscience. (2007) ;30: :123–52. |
[25] | Braak H , Braak E . Frequency of stages of Alzheimer-related lesions in different age categories. Neurobiology of aging. (1997) ;18: (4):351–7. |
[26] | Tan RH , Wong S , Kril JJ , Piguet O , Hornberger M , Hodges JR , et al. Beyond the temporal pole: limbic memory circuit in the semantic variant of primary progressive aphasia. Brain: a journal of neurology. (2014) ;137: (Pt 7):2065–76. |
[27] | Maki T , Choi YK , Miyamoto N , Shindo A , Liang AC , Ahn BJ , et al. A-Kinase Anchor Protein 12 Is Required for Oligodendrocyte Differentiation in Adult White Matter. Stem Cells (Dayton, Ohio). (2018) ;36: (5):751–60. |
[28] | 28. Xiao L , Ohayon D , McKenzie IA , Sinclair-Wilson A , Wright JL , Fudge AD , et al Rapid production of new oligodendrocytes is required in the earliest stages of motor-skill learning. Nature Neuroscience. (2016) ;19: (9):1210–7. |
[29] | Mandolesi G , Bullitta S , Fresegna D , De Vito F , Rizzo FR , Musella A , et al. Voluntary running wheel attenuates motor deterioration and brain damage in cuprizone-induced demyelination. Neurobiology of Disease. (2019) ;129: :102–17. |
[30] | Cahill LS , Steadman PE , Jones CE , Laliberte CL , Dazai J , Lerch JP , et al. MRI-detectable changes in mouse brain structure induced by voluntary exercise. NeuroImage. (2015) ;113: :175–83. |
[31] | Biedermann S , Fuss J , Zheng L , Sartorius A , Falfan-Melgoza C , Demirakca T , et al. In vivo voxel based morphometry: detection of increased hippocampal volume and decreased glutamate levels in exercising mice. NeuroImage. (2012) ;61: (4):1206–12. |
[32] | ten Brinke LF , Bolandzadeh N , Nagamatsu LS , Hsu CL , Davis JC , Miran-Khan K , et al. Aerobic exercise increases hippocampal volume in older women with probable mild cognitive impairment: a 6-month randomised controlled trial. British journal of sports medicine. (2015) ;49: (4):248–54. |
[33] | Rosano C , Guralnik J , Pahor M , Glynn NW , Newman AB , Ibrahim TS , et al. Hippocampal Response to a 24-Month Physical Activity Intervention in Sedentary Older Adults. The American journal of geriatric psychiatry: official journal of the American Association for Geriatric Psychiatry. (2017) ;25: (3):209–17. |
[34] | Chaddock L , Erickson KI , Prakash RS , Kim JS , Voss MW , Vanpatter M , et al. A neuroimaging investigation of the association between aerobic fitness, hippocampal volume, and memory performance in preadolescent children. Brain research. (2010) ;1358: :172–83. |
[35] | Thomas AG , Dennis A , Rawlings NB , Stagg CJ , Matthews L , Morris M , et al. Multi-modal characterization of rapid anterior hippocampal volume increase associated with aerobic exercise. NeuroImage. (2016) ;131: :162–70. |
[36] | Benedict C , Brooks SJ , Kullberg J , Nordenskjold R , Burgos J , Le Greves M , et al. Association between physical activity and brain health in older adults. Neurobiology of aging. (2013) ;34: (1):83–90. |
[37] | Gow AJ , Bastin ME , Muñoz Maniega S , Valdés Hernández MC , Morris Z , Murray C , et al. Neuroprotective lifestyles and the aging brain. Activity, atrophy, and white matter integrity. (2012) ;79: (17):1802–8. |
[38] | Chaddock-Heyman L , Erickson KI , Kienzler C , Drollette ES , Raine LB , Kao SC , et al. Physical Activity Increases White Matter Microstructure in Children. Frontiers in neuroscience. (2018) ;12: :950. |
[39] | Johnson NF , Kim C , Clasey JL , Bailey A , Gold BT . Cardiorespiratory fitness is positively correlated with cerebral white matter integrity in healthy seniors. NeuroImage. (2012) ;59: (2):1514–23. |
[40] | Oberlin LE , Verstynen TD , Burzynska AZ , Voss MW , Prakash RS , Chaddock-Heyman L , et al. White matter microstructure mediates the relationship between cardiorespiratory fitness and spatial working memory in older adults. NeuroImage. (2016) ;131: :91–101. |
[41] | Voss MW , Heo S , Prakash RS , Erickson KI , Alves H , Chaddock L , et al. The influence of aerobic fitness on cerebral white matter integrity and cognitive function in older adults: results of a one-year exercise intervention. Hum Brain Mapp. (2013) ;34: (11):2972–85. |
[42] | Barnett BR , Anderson JM , Torres-Velázquez M , Yi SY , Rowley PA , Yu J-PJ . Exercise ameliorates deficits in neural microstructure in a Disc1 model of psychiatric illness. Magnetic Resonance Imaging. (2019) ;61: :90–6. |
[43] | Sahnoune I , Inoue T , Kesler SR , Rodgers SP , Sabek OM , Pedersen SE , et al. Exercise ameliorates neurocognitive impairments in a translational model of pediatric radiotherapy. Neuro Oncol. (2018) ;20: (5):695–704. |
[44] | Engel A , Hijmans BS , Cerliani L , Bangert M , Nanetti L , Keller PE , et al. Inter-individual differences in audio-motor learning of piano melodies and white matter fiber tract architecture. Hum Brain Mapp. (2014) ;35: (5):2483–97. |
[45] | Scholz J , Niibori Y , W Frankland P , P Lerch J . Rotarod training in mice is associated with changes in brain structure observable with multimodal MRI. NeuroImage. (2015) ;107: :182–9. |
[46] | Lerch JP , Yiu AP , Martinez-Canabal A , Pekar T , Bohbot VD , Frankland PW , et al. Maze training in mice induces MRI-detectable brain shape changes specific to the type of learning. NeuroImage. (2011) ;54: (3):2086–95. |
[47] | Sagi Y , Tavor I , Hofstetter S , Tzur-Moryosef S , Blumenfeld-Katzir T , Assaf Y . Learning in the Fast Lane: New Insights into Neuroplasticity. Neuron. (2012) ;73: (6):1195–203. |
[48] | Nieuwboer A , Rochester L , Müncks L , Swinnen SP . Motor learning in Parkinson’s disease: limitations and potential for rehabilitation. Parkinsonism & Related Disorders. (2009) ;15: :S53–S8. |
[49] | Mann N , Rosenzweig A . Can exercise teach us how to treat heart disease? Circulation. (2012) ;126: (22):2625–35. |
[50] | Mustroph ML , Chen S , Desai SC , Cay EB , DeYoung EK , Rhodes JS . Aerobic exercise is the critical variable in an enriched environment that increases hippocampal neurogenesis and water maze learning in male C57BL/6J mice. Neuroscience. (2012) ;219: :62–71. |
[51] | Kobilo T , Liu QR , Gandhi K , Mughal M , Shaham Y , van Praag H . Running is the neurogenic and neurotrophic stimulus in environmental enrichment. Learning & memory (Cold Spring Harbor, NY). (2011) ;18: (9):605–9. |
[52] | Marks B , Katz L , Styner M , Smith J . Aerobic fitness and obesity: relationship to cerebral white matter integrity in the brain of active and sedentary older adults. British Journal of Sports Medicine. (2011) ;45: (15):1208–15. |
[53] | Best JR , Rosano C , Aizenstein HJ , Tian Q , Boudreau RM , Ayonayon HN , et al. Long-term changes in time spent walking and subsequent cognitive and structural brain changes in older adults. Neurobiology of Aging. (2017) ;57: :153–61. |
[54] | Moccia M , de Stefano N , Barkhof F . Imaging outcome measures for progressive multiple sclerosis trials. Multiple sclerosis (Houndmills, Basingstoke, England). (2017) ;23: (12):1614–26. |
[55] | Mastropietro A , Rizzo G , Fontana L , Figini M , Bernardini B , Straffi L , et al. Microstructural characterization of corticospinal tract in subacute and chronic stroke patients with distal lesions by means of advanced diffusion MRI. Neuroradiology. (2019) ;61: (9):1033–45. |
[56] | Song S-K , Yoshino J , Le TQ , Lin S-J , Sun S-W , Cross AH , et al. Demyelination increases radial diffusivity in corpus callosum of mouse brain. NeuroImage. (2005) ;26: (1):132–40. |
[57] | Kleemeyer MM , Kuhn S , Prindle J , Bodammer NC , Brechtel L , Garthe A , et al. Changes in fitness are associated with changes in hippocampal microstructure and hippocampal volume among older adults. NeuroImage. (2016) ;131: :155–61. |
[58] | Biedermann SV , Fuss J , Steinle J , Auer MK , Dormann C , Falfán-Melgoza C , et al. The hippocampus and exercise: histological correlates of MR-detected volume changes. Brain Structure and Function. (2016) ;221: (3):1353–63. |
[59] | Fuss J , Biedermann SV , Falfan-Melgoza C , Auer MK , Zheng L , Steinle J , et al. Exercise boosts hippocampal volume by preventing early age-related gray matter loss. Hippocampus. (2014) ;24: (2):131–4. |
[60] | Steadman PE , Xia F , Ahmed M , Mocle AJ , Penning ARA , Geraghty AC , et al. Disruption of Oligodendrogenesis Impairs Memory Consolidation in Adult Mice. Neuron. 2019. |
[61] | Kato D , Wake H , Lee PR , Tachibana Y , Ono R , Sugio S , et al. Motor learning requires myelination to reduce asynchrony and spontaneity in neural activity. Glia. (2020) ;68: (1):193–210. |
[62] | McKenzie IA , Ohayon D , Li H , de Faria JP , Emery B , Tohyama K , et al. Motor skill learning requires active central myelination. Science (New York, NY). (2014) ;346: (6207):318–22. |
[63] | Zheng J , Sun X , Ma C , Li BM , Luo F . Voluntary wheel running promotes myelination in the motor cortex through Wnt signaling in mice. Molecular Brain. (2019) ;12: (1):85. |
[64] | Chapman TW , Hill RA . Myelin plasticity in adulthood and aging. Neuroscience Letters. 2019:134645. |
[65] | Bergles DE , Richardson WD . Oligodendrocyte Development and Plasticity. Cold Spring Harbor Perspectives in Biology. (2015) ;8: (2):a020453. |
[66] | Bracht T , Jones DK , Bells S , Walther S , Drakesmith M , Linden D . Myelination of the right parahippocampal cingulum is associated with physical activity in young healthy adults. Brain Structure and Function. (2016) ;221: (9):4537–48. |
[67] | Chang EH , Argyelan M , Aggarwal M , Chandon T-SS , Karlsgodt KH , Mori S , et al. The role of myelination in measures of white matter integrity: Combination of diffusion tensor imaging and two-photon microscopy of CLARITY intact brains. NeuroImage. (2017) ;147: :253–61. |
[68] | Amaral DG , Scharfman HE , Lavenex P . The dentate gyrus: fundamental neuroanatomical organization (dentate gyrus for dummies). Prog Brain Res. (2007) ;163: :3–22. |
[69] | Vivar C , Peterson BD , van Praag H . Running rewires the neuronal network of adult-born dentate granule cells. NeuroImage. (2016) ;131: :29–41. |