Higher Cardiorespiratory Fitness is Associated with Reduced Functional Brain Connectivity During Performance of the Stroop Task
Abstract
Background:
Although higher cardiorespiratory fitness (CRF) has been linked to better executive function, the mechanisms by which this occurs remain a matter of speculation. One hypothesis is that higher CRF is associated with elevated top-down control in which brain regions processing task-relevant information are up-regulated and brain regions processing task-irrelevant information are down-regulated.
Methods:
We tested this top-down hypothesis in 50 young adults (μ age = 25.22 ± 5.17 years) by measuring CRF via a graded maximal exercise test and performing functional Magnetic Resonance Imaging (fMRI) during a color-word Stroop task. We used task-evoked functional connectivity, quantified from a psychophysiological interaction analysis (PPI), to test our hypotheses that (a) higher CRF would be associated with greater connectivity between control centers (i.e., prefrontal and parietal areas) and visual feature centers (i.e., occipital areas) that are involved with processing task-relevant stimulus dimensions (i.e., color), and (b) higher CRF would be associated with lower connectivity between control centers and visual feature centers that are involved with processing task-irrelevant dimensions of the stimuli (i.e., word processing areas).
Results:
Controlling for sex and BMI, we found, consistent with our second hypothesis, that higher CRF was associated with reduced functional connectivity between parietal and occipital areas involved in the task-irrelevant dimension of the task (i.e., word form areas). There were no associations between CRF and functional connectivity with the prefrontal cortex or evidence of heightened connectivity between attentional control and visual feature centers.
Conclusions:
These results suggest that CRF associations with executive functioning might be explained by CRF-mediated differences between brain regions involved with attentional control (parietal regions) and the down-regulation of regions involved with processing task-irrelevant stimulus features (occipital regions).
INTRODUCTION
Higher cardiorespiratory fitness (CRF) has often been associated with better performance on the cognitively-challenging conditions of executive function (EF) tasks, such as the incongruent conditions of the Stroop and Flanker tasks and the dual-task condition in dual-task paradigms [1–6]. Unfortunately, despite this relatively consistent association across the literature, we have a very poor understanding of the mechanisms by which CRF is associated with enhanced EF. Functional MRI studies have demonstrated that higher CRF is associated with greater activation in brain areas that support attentional control processes (i.e., prefrontal and parietal cortices) during more cognitively-demanding conditions (e.g., incongruent condition of the Stroop task) [1, 2, 7]. These prior fMRI studies have been informative for identifying which brain areas differ as a function of CRF during EF tasks, but they do not tell us if these brain areas are working in concert or independently [8]. In addition, there have been few theoretical models describing how these differences in brain activation are linked with better EF.
Despite prior studies focusing on CRF-related differences in activation of the prefrontal and parietal regions, it is possible that brain areas supporting sensory processing (e.g., visual cortex) are also associated with CRF and linked with better EF performance. For example, there is an abundance of data demonstrating that top-down processes are reflected in the brain by both an up-regulation and down-regulation of sensory brain circuits involved in processing stimulus features [9–14]. These top-down processes are characterized by enhancement (i.e., up-regulation) of task-relevant brain regions and suppression (i.e., down-regulation) of task-irrelevant regions to maximize efficiency in cognitive processing. We, and others, have proposed that higher CRF might be linked with elevated EF performance because of an enhancement in top-down control [7, 15–18]. If true, then higher CRF would be associated with the modulation of sensory brain areas involved in processing stimulus features. A test of this hypothesis would be to examine whether CRF explains variability in task-evoked functional connectivity between prefrontal/parietal regions that support top-down attentional control processes and occipital regions involved in bottom-up visual feature processes. Both enhancement of brain regions processing task-relevant features and suppression of regions processing task-irrelevant features could be associated with enhanced task performance.
In the current study, we examined the role of CRF in attentional control in a sample of 50 young adults with a wide range of fitness levels in the context of a Stroop task. We had two primary hypotheses that, if supported, would be consistent with a top-down model as an explanation for the link between CRF and EF. First, we predicted that higher CRF would be associated with greater connectivity between control centers (i.e., prefrontal and parietal areas) and visual feature centers (i.e., occipital areas) that have been previously associated with processing task-relevant stimulus dimensions (i.e., color). Second, we predicted that higher CRF would be associated with reduced connectivity between control centers (i.e., prefrontal and parietal areas) and visual feature centers (i.e., occipital regions) that have been associated with processing task-irrelevant dimensions of the stimuli (i.e., word processing areas).
MATERIALS AND METHODS
Participants
Adults between 18 and 40 years old were recruited from the Pittsburgh community. Participants were eligible if they did not have a history of a neurological or health condition that could affect central nervous system functioning, including a history of psychiatric disease, epilepsy, or metabolic disorder. All participants were screened over the phone prior to enrollment and again during the consenting process to ensure they were healthy enough to engage in a maximal exercise fitness test and were safe for magnetic resonance imaging (MRI). Participants were ineligible if they had a history of: physical conditions that precluded them from engaging in physical activity or the maximal exercise fitness test safely (e.g., current or history of cardiovascular disease, ambulatory problems), color blindness, previous head trauma, previous brain surgery, a history of a neurological disorder or condition (e.g., dementia, stroke), or any preclusions to neuroimaging (e.g., metal that would interfere with MRI). Additional details about study participants and procedures have been previously published [e.g., 19, 20]. All protocols and procedures were approved by the University of Pittsburgh Institutional Review Board, and informed consent was obtained in accordance with the principles set forth in the Declaration of Helsinki.
Procedures
Cardiorespiratory fitness testing
CRF was measured using a graded maximal exercise test on a motor driven treadmill. VO2peak was defined as the highest recorded VO2 value based on the following criteria: either a plateau in VO2 peak between two or more workloads (0.15 L/min or 2.0 ml/kg/min) or meeting two out of three criteria: a respiratory exchange ratio greater than or equal to 1.10, a heart rate within 10 beats of their age-predicted maximum (i.e., 220 – age), or a rating of perceived exertion (RPE) greater than or equal to 17.
Participants were first acclimated to the environment and test procedures and then began by warming-up by walking at 3.0 mph at a 0% incline. All participants completed a modified Bruce protocol, beginning with Stage 1 at 3.5 mph and a 2.0% incline. Subsequent stages were reached every two minutes when the speed increased by 0.50 mph and the grade increased by 2% to increase exercise intensity linearly. Participants continued to increase their workload until they reached volitional fatigue or any observed symptoms that required discontinuation of the test. Oxygen uptake, heart rate, and blood pressure were continuously monitored by a trained exercise physiologist. Gas exchange values were measured using a Parvo Medics metabolic cart using expired breath-by-breath air samples averaged at 15-second intervals via a mouthpiece connected to a two-way valve. The mouthpiece was supported with comfort fitted head gear and participants wore nose clips to ensure all expired air was collected. All equipment was worn until VO2peak was attained through symptom limitation and/or self-reported exhaustion. 49 of the 50 participants met the VO2peak criteria listed above; one participant did not meet these criteria and discontinued the test from volitional exhaustion.
Stroop task
Words were presented in an event-related fashion while participants were in an MRI scanner. The Stroop task measures selective attention by utilizing three task conditions: congruent, incongruent, and neutral [21]. In the congruent condition, the color and meaning of the word are identical (e.g., the word “RED” in red ink). In the incongruent condition, the color and meaning of the word are different (e.g., the word “RED” in blue ink). In the neutral condition, non-color words are used (e.g., the word “BOAT” in red ink). Compared to the congruent trials, the incongruent trials require greater attentional demands due to the difference in word meaning and ink color. The Stroop effect was calculated with the following equation: (incongruent – congruent) / congruent. Participants indicated their choice with button presses using a glove on their right hand. Each trial had a maximum window of 1.5 seconds to respond. If a response was not detected in this time, it was recorded as a miss/error. A total of 182 trials were presented (48 incongruent, 54 congruent, 63 neutral).
Neuroimaging
Acquisition
All participants completed an MRI scan that included structural and functional brain images during a separate visit and within two weeks of CRF testing. Images were collected on a 3.0 Tesla, head-only Siemens Allegra scanner. High-resolution T1-weighted brain images were acquired using a 3-dimensional magnetization prepared rapid-acquisition gradient echo (MPRAGE) protocol with 176 contiguous axial slices (slice thickness = 1mm, repetition time = 1.4s, echo time = 2.48ms, field of view [FOV] = 256mm, matrix = 256×256, flip angle = 8). Functional brain images were acquired while participants completed the Stroop task. Functional scan acquisition consisted of 290 blood oxygen level dependent (BOLD) measurements over 8 : 18 minutes for each participant with whole-brain coverage (repetition time = 1.7 s, echo time = 25ms, FOV = 205 mm, matrix = 64×64, flip angle = 90, slice thickness = 3.5 mm, 33 slices).
Preprocessing
All MRI data were processed and analyzed using FMRIB’s software library (FSL) 5.0 (Oxford Center for the fMRI of the Brain; www.fmrib.ox.ac.uk/fsl). Each MPRAGE image was skull-stripped using the brain extraction tool (BET) and spatially normalized into standard space (i.e., using the Montreal Neurological Institute (MNI) image). All functional images were skull-stripped using BET and were motion-corrected to account for any head movement in the scanner by using the middle image in the timeseries as the reference image. The data were highpass filtered using a threshold of 40 seconds and spatially smoothed using a 7.0 mm full width at half maximum (FWHM) Gaussian kernel. FMRI scans were then co-registered to the participant’s corresponding MPRAGE image and MNI space using 7 and 12 degrees-of-freedom, respectively.
Statistical analysis
Behavioral analyses were conducted using IBM SPSS 24. Bivariate correlations examined the relationships between demographic variables (i.e., age, sex, BMI, education) and CRF (i.e., VO2peak). The relationships between CRF and Stroop task performance were assessed with hierarchical multiple regressions that included sex and BMI as covariates. Stroop task performance was evaluated by response times (RT) during each of the three conditions and as an interference score (i.e., Stroop effect).
Task-evoked analyses
All neuroimaging statistical analyses were completed using FEAT in FSL. First, individual, first-level analyses were conducted using FMRIB’s Improved Linear Model (FILM), which uses a nonparametric estimation of time-series autocorrelation to improve estimation efficiency. These analyses model fMRI signal changes for each condition of the Stroop task for each participant. Higher-level analyses were then conducted using FMRIB’s Local Analysis of Mixed Effects (FLAME), which combines the first-level analyses and estimates the random-effects variance of the mixed-effects model at each voxel. Sex and BMI were included as covariates. These group-level analyses tested for differences in activation for each of the Stroop task conditions as a function of CRF. The results were corrected for multiple comparisons by using a voxel-wise threshold of p < .01 and cluster-thresholding of p < .05, which is a method of family-wise error correction based on Gaussian random field theory.
Connectivity analyses
A psychophysiological interaction (PPI) analysis tests for task-evoked functional connectivity between a brain region identified as a ‘seed region’ and all other voxels in the brain [22, 23]. We followed standard procedures for conducting PPI analyses. Specifically, we first identified areas in which the contrast between incongruent and congruent conditions was associated with CRF. This first step is critical because our aim was to examine whether brain areas that are associated with CRF might vary in level of functional connectivity with other brain regions. Thus, using seed regions shown to be sensitive to CRF is not ‘double-dipping’, such that activation patterns or contrasts related to CRF do not bias the results towards finding differences in connectivity as a function of CRF nor do they bias the results in favor of a particular direction of correlation with connectivity patterns. Next, we extracted the average time course from each region for the PPI analysis. We focused our PPI analyses on variation in functional connectivity during the incongruent condition since that is the condition requiring inhibitory control and which performance is associated with CRF (see below). Thus, each region’s time course during the incongruent-fixation contrast was added to the first-level model, along with errors during the incongruent condition and up to six directions of motion if any individual motion parameter exceeded a framewise displacement of 1.7 mm. A PPI regressor was created in this first-level model by calculating an interaction term between the seed time course and the incongruent condition of the Stroop task.
The resulting statistical maps were then entered into higher-level analyses to evaluate whether there were any statistically significant differences in connectivity between the seed regions and other brain areas as a function of CRF. We included sex and BMI as additional regressors in these higher-level analyses. All resulting activation clusters were corrected for multiple comparisons using cluster-thresholding set at p < .05 and a voxel-wise threshold of p < .01. Finally, any resulting activation clusters where functional connectivity was associated with CRF were included in linear regression models to evaluate their relationships with Stroop task performance.
RESULTS
Participants
50 participants completed the MRI protocol and were used for analyses. On average, participants were 25 years old (M = 25.22, SD = 5.17 years) and 56% were female (see Table 1 for demographic information).
Table 1
Demographic information and bivariate correlations between demographic information and cardiorespiratory fitness
Mean±SD | Pearson’s correlation with CRF (r) | Significance | |
Age (years) | 25.22±5.17 | –0.057 | 0.693 |
# Female (%)** | 28 (56%) | –0.501 | <.001** |
Race (% Caucasian) | 80% | 0.080 | 0.580 |
BMI (kg/m2)** | 26.25±4.83 | –0.373 | 0.008** |
Weight (lbs) | 165.80±35.94 | –0.222 | 0.121 |
Education (years) | 15.98±2.11 | –0.87 | 0.547 |
Peak VO2 ml/kg/min | 40.88±7.92 | – | – |
**p < 0.01 Note: CRF = cardiorespiratory fitness; BMI = body mass index; kg = kilogram; m = meters; lbs = pounds; ml = milliliters; min = minute.
Cardiorespiratory fitness
Participants’ CRF ranged from 25.38 to 60.38 ml/kg/min (within-sample 25th percentile =35.26 ml/kg/min; within-sample 75th percentile =46.67 ml/kg/min) with an average of 40.88 ml/kg/min (SD = 7.92). We identified potential confounding factors by evaluating the relationships between demographic variables (i.e., age, sex, race, BMI, education) and CRF (i.e., peak VO2 ml/kg/min). Only sex and BMI were related to CRF (see Table 2), and were included as covariates in all subsequent analyses.
Table 2
Activation clusters related to cardiorespiratory fitness in the Incongruent > Congruent contrast
# Voxels | % Signal change (mean±SD) | Maximum Z-score | Peak X (mm) | Peak Y (mm) | Peak Z (mm) | |
L dlPFC | 680 | 0.049±0.127 | 3.92 | –36 | 50 | 16 |
R dlPFC | 364 | 0.059 ±0.138 | 3.80 | 26 | 60 | 14 |
L Postcentral Gyrus/Supramarginal Gyrus | 2673 | 0.131±0.228 | 4.39 | –26 | –54 | 46 |
R Supramarginal Gyrus | 639 | 0.072±0.104 | 4.11 | 40 | –42 | 36 |
Caudate Nucleus | 2011 | 0.058±0.110 | 4.15 | 8 | 12 | 4 |
L Angular Gyrus | 348 | 0.087±0.183 | 3.58 | –48 | –48 | 28 |
R Cerebellum | 385 | 0.042±0.142 | 3.43 | 20 | –56 | –24 |
R Middle Temporal Gyrus | 335 | 0.038±0.137 | 3.78 | 64 | –22 | –4 |
Note: L = left; R = right; dlPFC = dorsolateral prefrontal cortex; mm = millimeters.
Stroop task performance
Consistent with previous literature, participants performed slowest in the incongruent condition (mean RT = 740.97 + 85.36ms; 95% accuracy) and faster in the congruent (mean RT = 649.25 + 65.89ms; 97% accuracy) and neutral (mean RT = 675.85 + 69.81ms; 98% accuracy) conditions. There was a significant Stroop effect, with participants performing significantly faster during the congruent relative to the incongruent condition, t(49) = 10.12, p < .001, and faster during the neutral relative to the incongruent condition, t(49) = 7.93, p < .001. Participants also performed significantly faster in the congruent relative to the neutral condition, consistent with a facilitation effect, t(49) =–7.57, p < .001. Due to the high accuracy observed for all conditions, we focused on RT in subsequent analyses.
Bivariate correlations between demographic variables (i.e., age, sex, race, BMI, education) and Stroop task performance did not yield any significant relationships (all p-values>.110). Individuals with higher CRF responded faster during the incongruent (β= –.457, t(46) =–2.425, p = .019) and neutral (β= –.428, t(46) =–2.289, p = .027) conditions of the task relative to those with lower CRF after controlling for sex and BMI. Relationships between the congruent condition or the Stroop effect and CRF were not statistically significant (congruent β= –.290, Stroop Effect β= –.259; all p-values>.137).
CRF and Stroop task activation
We identified eight significant clusters for the incongruent-congruent contrast that were associated with CRF. These clusters were located in areas consistent with prior literature of the Stroop task and included the left and right dorsolateral prefrontal cortices (dlPFC), right supramarginal gyrus, left postcentral gyrus/supramarginal gyrus, bilateral caudate nucleus, right middle temporal gyrus, left angular gyrus, and right cerebellum (see Table 2 and Fig. 1). For each of these clusters, higher CRF was associated with a smaller difference in activity after controlling for sex and BMI. We extracted the time series from these regions for seeds in the PPI analyses.
Fig.1
Activation clusters with greater activity related to lower cardiorespiratory fitness during the Incongruent > Congruent condition contrast. Note: BG = basal ganglia; cereb = cerebellum; MTG = middle temporal gyrus; dlPFC = dorsolateral prefrontal cortex; AG = angular gyrus; PC/SMG = postcentral/supramarginal gyri.
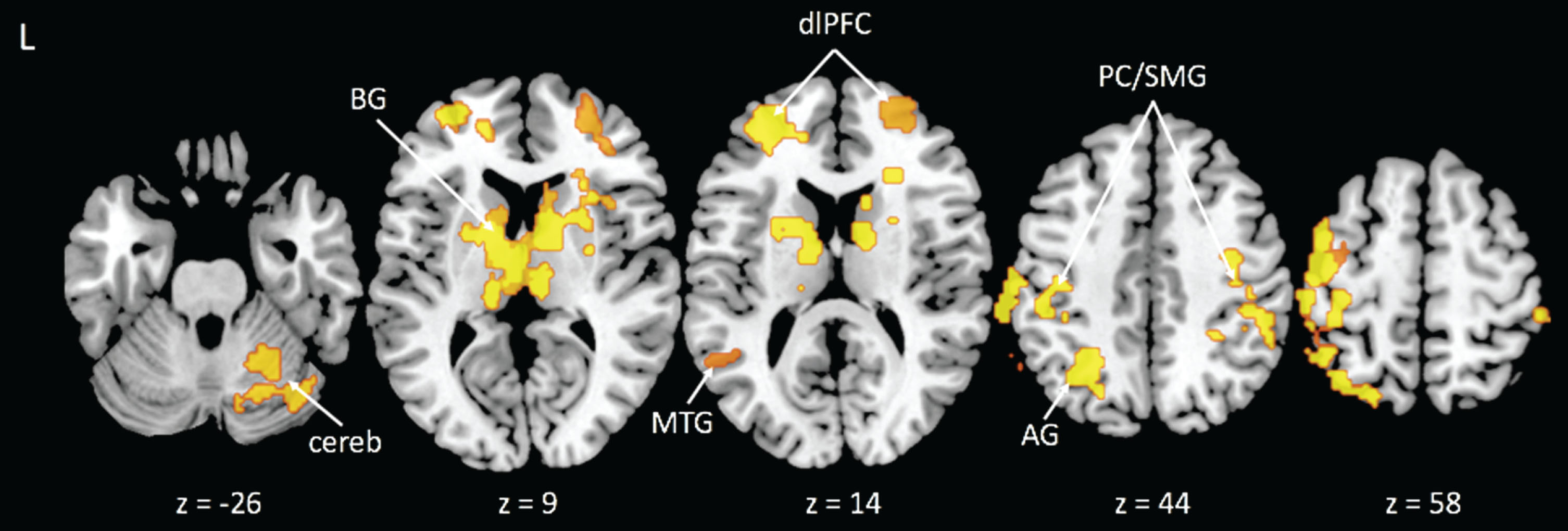
CRF and functional connectivity
Consistent with our second prediction, the PPI analyses revealed that higher CRF was associated with significant differences in connectivity between regions evoked during the task with a visual feature area of the occipital cortex. Specifically, our results showed that higher CRF was associated with reduced connectivity between several brain areas evoked by the incongruent condition of the task (i.e., right supramarginal gyrus, right middle temporal gyrus, and left angular gyrus) and the left occipital fusiform gyrus (see Table 3 and Figs 2, 3). This area of the left occipital fusiform gyrus has been associated with visual word form detection at peak coordinates of approximately –43, –54, –12 [24–26]. Previous work shows that activation in this area is increased during task conditions (i.e., incongruent condition) in which the word is the distractor [11, 15]. Consistent with previous literature, we found that higher CRF was associated with reduced connectivity between attentional control areas and activity near this region with peak coordinates at approximately –34, –74, –10. The left postcentral/supramarginal gyri showed a similar pattern of reduced connectivity to the same left occipital fusiform gyrus cluster, but at subthreshold levels. We also found that higher CRF was associated with reduced connectivity between the right middle temporal gyrus and the lingual gyrus, bilaterally. The lingual gyrus has been implicated in letter and word detection [27], as well as in semantic processing [28].
Table 3
Higher cardiorespiratory fitness is associated with reduced task-evoked functional connectivity between seed regions and activation clusters during the Incongruent condition
Seed region/Activation cluster | # Voxels | % Signal change (mean±SD) | Maximum Z-score | Peak X (mm) | Peak Y (mm) | Peak Z (mm) |
R Supramarginal Gyrus/VWFA | 362 | 0.175±0.251 | 4.03 | –34 | –74 | –12 |
L Angular Gyrus/VWFA | 581 | 0.275±0.278 | 4.20 | –30 | –84 | –10 |
R MTG/VWFA | 425 | 0.256±0.264 | 4.27 | –30 | –84 | –10 |
R MTG/Bilateral Lingual Gyrus | 443 | 0.131±0.218 | 3.61 | 4 | –80 | 2 |
Note: R = right; L = left; mm = millimeters; VWFA = Visual Word Form Area; MTG = middle temporal gyrus.
Fig.2
Reduced task-evoked functional connectivity during the Incongruent condition with greater cardiorespiratory fitness between the left angular gyrus (blue), right supramarginal gyrus (yellow), and right middle temporal gyrus (red) seed regions and the VWFA; overlap shown in pink.
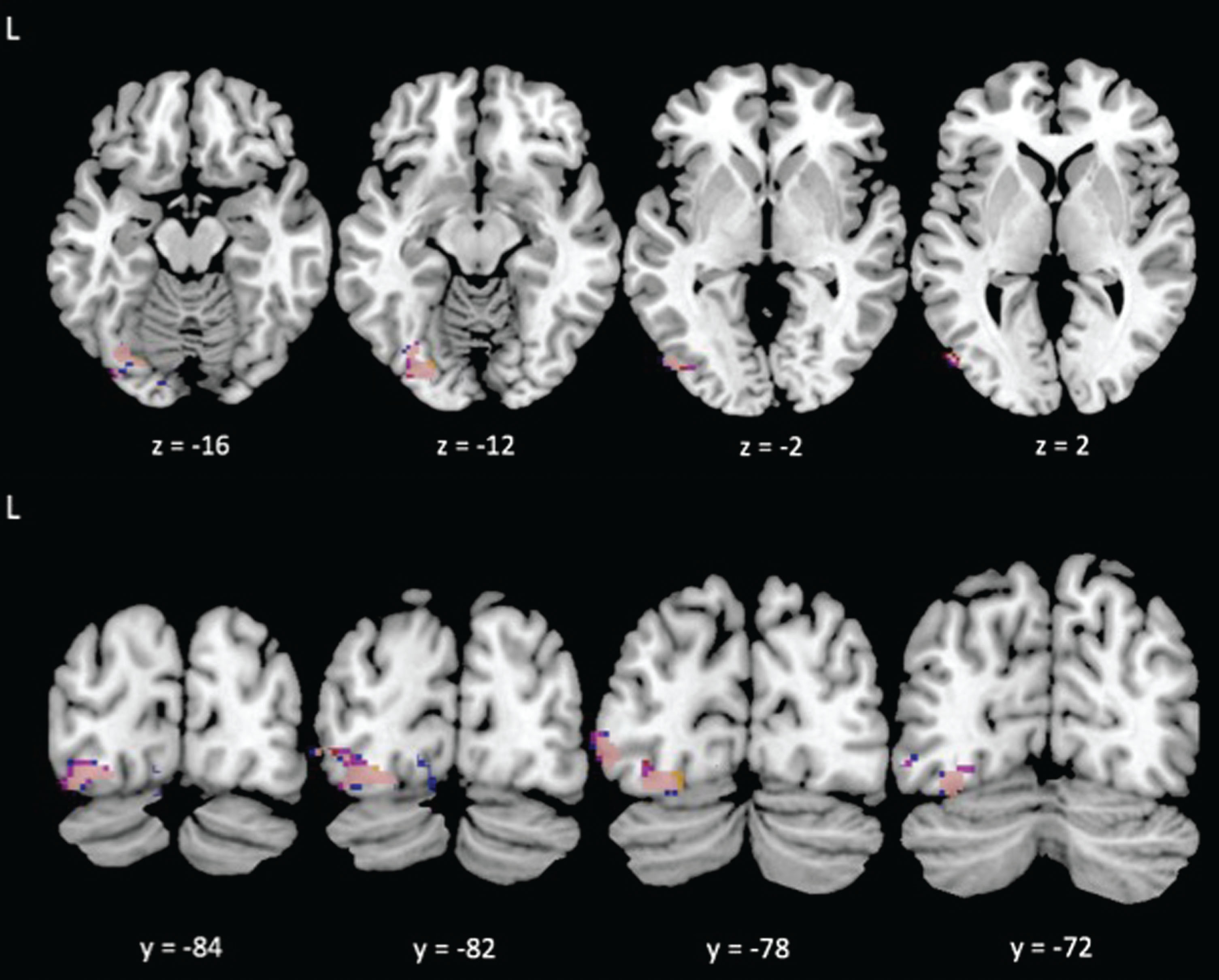
Fig.3
Scatterplots of reduced functional connectivity with higher cardiorespiratory fitness during the incongruent condition. a-c) Connectivity from the left angular gyrus (a), right middle temporal gyrus (b), and right supramarginal gyrus (c) to the VWFA; d) Connectivity from the right middle temporal gyrus to the bilateral lingual gyrus.
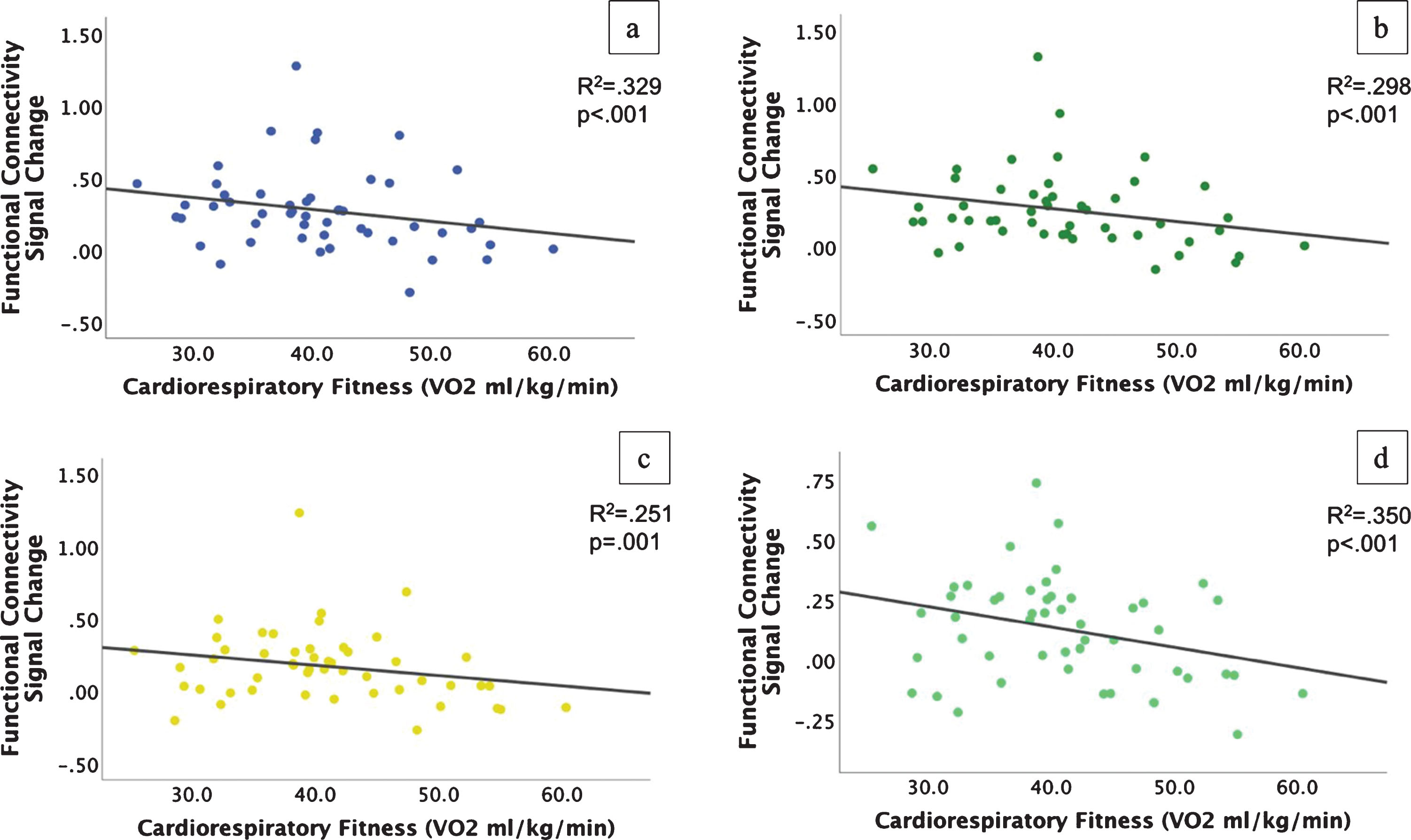
Contrary to our first prediction, we did not identify areas where heightened connectivity between regions evoked during the task and attentional control/visual feature centers were associated with CRF. There were no significant relationships between either dlPFC seed and connectivity in any area as a function of CRF.
Functional connectivity and Stroop task performance
Given that our functional connectivity analyses were conducted for the incongruent task condition, we examined whether the relationships between CRF and connectivity were related to RT in the incongruent condition. We found that greater functional connectivity between the left angular gyrus and left occipital fusiform gyrus was associated with longer RTs (poorer performance) during the incongruent condition, β= 0.314, p = 0.026. This is consistent with our finding that higher CRF was associated with reduced connectivity between these regions. All other relationships between functional connectivity and RT were trending toward statistical significance (all p-values < 0.088). For all of these regions, the relationships matched the direction of the relationships between CRF and functional connectivity, i.e., faster RT was associated with reduced connectivity between the right supramarginal gyrus/right middle temporal gyrus and left occipital fusiform gyrus, and reduced connectivity between the right middle temporal gyrus and bilateral lingual gyri.
DISCUSSION
This was the first study to examine whether CRF could explain variability in task-evoked functional connectivity and be a possible explanation for how CRF is associated with enhanced EF. In line with prior literature, higher CRF was associated with better performance (i.e., faster RT) on the incongruent condition of the Stroop task. Higher CRF was also associated with activation in areas of the PFC, anterior and posterior parietal cortex, caudate nucleus, and occipital cortex that are consistent with previous literature on Stroop task performance [9, 11, 29, 30]. Consistent with one of our predictions, higher CRF was associated with reduced task-evoked functional connectivity between areas that support attentional control (i.e., parietal cortex) and word detection (i.e., left occipital fusiform gyrus), suggesting that higher CRF is associated with top-down control operations that down-regulate activity in areas that process task-irrelevant information. However, higher CRF was not associated with heightened task-evoked functional connectivity between attentional control and task-relevant visual feature regions. Together, our findings suggest that enhanced down-regulation of brain regions involved in task-irrelevant sensory processing may be one mechanism by which CRF is associated with elevated executive function.
Higher fit young adults showed reduced functional connectivity between brain regions associated with attentional control and regions involved in reading words. Word detection is a highly automatized process that must be inhibited for successful task performance in the incongruent condition. We found that higher fit participants showed reduced connectivity between areas within the visual attentional control network (i.e., right supramarginal gyrus, right middle temporal gyrus, left angular gyrus) and the left occipital fusiform gyrus, a brain region commonly associated with a visual word form area (VWFA) [24, 26]. Reduced connectivity between these regions as a function of increasing CRF might indicate active inhibition or suppression of the brain regions associated with processing task-irrelevant information [12, 31]. Consistent with our suppression hypothesis, this is likely related to the exertion of top-down control operations within visual attentional circuitry [10, 32] acting on bottom-up processing centers involved in reading words during the Stroop task [11, 12]. When individuals are asked to inhibit the automatic response set of reading, it may be that those with higher CRF are more effectively able to use top-down control operations [4], partly supported by parietal regions, to suppress the areas of the striate and extrastriate cortices that detect word features [7, 15]. This heightened suppression of brain areas associated with automatic responses (i.e., reading) may indicate that individuals with higher CRF perform executive functions, such as attentional and inhibitory control, more efficiently than those with lower CRF because of enhanced top-down control of brain areas responsible for processing task-irrelevant dimensions of stimuli.
Better behavioral Stroop task performance, i.e., faster RT in the incongruent condition, was associated with reduced connectivity between the left angular gyrus and VWFA. This result supports our suppression hypothesis that higher CRF is associated with elevated performance on measures of EF by down-regulating regions involved in processing visual features. Other brain areas associated with lower functional connectivity with higher CRF were not significantly associated with performance, but the associations were all trending in the same direction. Although not statistically significant, the direction of these relationships suggest that associations between these brain areas might have direct relevance to the CRF link to better EF. Given that CRF is influenced by modifiable lifestyle factors, such as physical activity engagement, our evidence suggests that brain function might be malleable throughout the lifespan.
Surprisingly, we did not find significant associations between CRF and connectivity of the dorsolateral prefrontal regions. Given the importance of the prefrontal cortex with supporting EF and top-down attentional control operations [9, 30, 33–37], we hypothesized that CRF would influence the connectivity between the dorsolateral prefrontal cortex and posterior brain areas. It is possible that the patterns of connectivity between prefrontal areas and other brain regions differ across the lifespan [38, 39]. Younger adults rely more on posterior regions, including the fusiform gyrus, for completion of selective attention tasks [40], and less on prefrontal regions compared to older adults [1, 2, 7]. While the PFC is a key region for top-down processing, its contributions are likely strongly connected to other regions, such as the anterior cingulate cortex or superior parietal cortex [41–43]. We also did not find any heightened connectivity between attentional control and task-relevant visual feature centers relative to CRF. It is possible that CRF does not influence the up-regulation of task-relevant processing and that the only effects on attentional control are due to the suppression of irrelevant stimuli. However, it is also possible that the lack of significant associations in our sample may be due to the limitations of our study, which are described below.
We did not perform any type of retinotopic mapping procedures to determine whether the area of the visual cortex is clearly associated with word processing, which may limit the interpretations of our findings. Another limitation is that the participants were in an age range when the prefrontal cortex may be less affected by variability in CRF compared to other times in the lifespan (i.e., childhood or late life). As such, the participants might be in their ‘prime’ of cognitive functioning and variability in CRF might have a smaller impact on brain health in this age range. However, our results provide evidence that CRF is related to top-down modulation of a visual cortex area in a younger sample that is less frequently studied and suggests that at least some aspects of brain health in younger adults are influenced by markers of physical health such as CRF.
Despite these limitations, the results of the current study suggest that down-regulation of task-irrelevant brain regions might be one mechanism by which CRF is associated with elevated EF. Increasing exercise engagement is capable of increasing CRF and has also been shown to enhance brain plasticity in animal models [44, 45] and in humans [46–49]. Higher CRF is also associated with better cognitive performance [50–52] and resting state functional connectivity [53–56] in cross-sectional studies and following exercise interventions.
We found that higher CRF was associated with reduced connectivity between attentional control regions and areas linked with word or letter processing (i.e., task-irrelevant information) during the incongruent condition of the Stroop task. Our results also provide evidence that CRF is associated with task-evoked functional connectivity during a cognitively-demanding task and that this relationship can be detected in younger adults, an age range less frequently studied in the context of CRF and brain function, indicating that CRF is associated with heightened brain function throughout the lifespan. Future work should replicate these results in larger samples and expand it to other executively-demanding tasks, and could consider expanding into measuring cerebrovascular reactivity beyond task-evoked BOLD signal change.
CONFLICTS OF INTEREST
The authors have no conflicts of interest to report.
ACKNOWLEDGMENTS
This research was supported by funding to K.I.E. from the National Institute of Diabetes and Digestive and Kidney Diseases grant R01 DK095172.
REFERENCES
[1] | Colcombe S , Kramer AF , Erickson KI , Scalf P , McAuley E , Cohen NJ , et al. Cardiovascular fitness, cortical plasticity, and aging. Proceedings of the National Academy of Sciences. (2004) ;101: (9):3316–21. |
[2] | Wong C , Chaddock-Heyman L , Voss M , Burzynska A , Basak C , Erickson KI , et al. Brain activation during dual-task processing is associated with cardiorespiratory fitness and performance in older adults. Frontiers in Aging Neuroscience. (2014) ;7. |
[3] | Pontiflex M , Raine L , Johnson C , Chaddock L , Voss M , Cohen NJ , et al. Cardiorespiratory fitness and the flexible modulation of cognitive control in preadolescent children. Journal of Cognitive Neuroscience. (2011) ;23: (6):1332–45. |
[4] | Wengaard E , Kristoffersen M , Harris A , Gundersen H . Cardiorespiratory fitness is associated with selective attention in healthy male high-school students. Front Hum Neurosci. (2017) ;11. |
[5] | Bauermeister S , Bunce D . Aerobic fitness and intraindividual reaction time variability in middle and old age. J Gerontol B Psychol Sci Soc Sci. (2016) ;71: (3):431–8. |
[6] | Pentikainen H , Savonen K , Ngandu T , Solomon A , Komulainen P , Paajanen T , et al. Cardiorespiratory Fitness and Cognition: Longitudinal Associations in the FINGER Study. J Alzheimers Dis. (2019) ;68: (3):961–8. |
[7] | Prakash R , Voss M , Erickson KI , Lewis J , Chaddock L , Malkowski E , et al. Cardiorespiratory fitness and attentional control in the aging brain. Frontiers in Human Neuroscience. (2011) ;4. |
[8] | Voss M , Erickson KI , Prakash R , Chaddock L , Malkowski E , Alves H , et al. Functional connectivity: a source of variance in the association between cardiorespiratory fitness and cognition? Neuropsychologia. (2010) ;48: (5):1394–406. |
[9] | Banich MT , Milham MP , Atchley R , Cohen NJ , Webb A , Wszalek T , et al. fMRI studies of Stroop tasks reveal unique roles of anterior and posterior brain systems in attentional selection. J Cogn Neurosci. (2000) ;12: (6):988–1000. |
[10] | Corbetta M , Shulman G . Control of goal-directed and stimulus-driven attention in the brain. Nature Reviews Neuroscience. (2002) ;3: (3). |
[11] | Milham MP , Erickson KI , Banich MT , Kramer AF , Webb A , Wszalek T , et al. Attentional control in the aging brain: insights from an fMRI study of the stroop task. Brain Cogn. (2002) ;49: (3):277–96. |
[12] | Polk T , Drake R , Jonides J , Smith M , Smith E . Attention enhances the neural processing of relevant features and suppresses the processing of irrelevant features in humans: a functional magnetic resonance imaging study of the Stroop task. Journal of Neuroscience. (2008) ;28: (51):13786–92. |
[13] | Zanto T , Rissman J . Top-Down Suppression. Brain Mapping: An Encyclopedic Reference. (2015) ;3: :261–7. |
[14] | Noonan M , Crittenden B , Jensen O , Stokes M . Selective inhibition of distracting input. Behav Brain Res. (2018) ;355: :36–47. |
[15] | Erickson KI , Prakash R , Kim J , Sutton B , Colcombe S , Kramer AF . Top-down attentional control in spatially coincident stimuli enhances activity in both task-relevant and task-irrelevant regions of cortex. Behavioural Brain Research. (2009) ;197: (1):186–97. |
[16] | Dupuy O , Gauthier CJ , Fraser SA , Desjardins-Crepeau L , Desjardins M , Mekary S , et al. Higher levels of cardiovascular fitness are associated with better executive function and prefrontal oxygenation in younger and older women. Front Hum Neurosci. (2015) ;9: :66. |
[17] | Voss M , Prakash R , Erickson K , Basak C , Chaddock L , Kim J , et al. Plasticity of brain networks in a randomized intervention trial of exercise training in older adults. Frontiers in Aging Neuroscience. (2010) ;2. |
[18] | Wang CH , Moreau D , Yang CT , Lin JT , Tsai YY , Tsai CL . The influence of aerobic fitness on top-down and bottom-up mechanisms of interference control. Neuropsychology. (2019) ;33: (2):245–55. |
[19] | Bento-Torres J , Bento-Torres NVO , Stillman CM , Grove GA Jr , Huang H , Uyar F , et al. Associations between cardiorespiratory fitness, physical activity, intraindividual variability in behavior, and cingulate cortex in younger adults. Journal of sport and health science. (2019) ;8: (4):315–24. |
[20] | Stillman C , Uyar F , Huang H , Grove G , Watt J , Wollam M , et al. Cardiorespiratory fitness is associated with enhanced hippocampal functional connectivity in healthy young adults. Hippocampus. (2018) ;28: (3):239–47. |
[21] | Stroop J . Studies of interference in serial verbal reactions. Journal of Experimental Psychology. (1935) ;18: (6):643–62. |
[22] | O’Reilly J , Woolrich M , Behrens T , Smith S , Johansen-Berg H . Tools of the Trade: Psychophysiological Interactions and Functional Connectivity. Social Cognitive and Affective Neuroscience. (2012) ;7: (5):604–9. |
[23] | Friston K , Buechel C , Fink G , Morris J , Rolls E , Dolan RJ . Psychophysiological and modulatory interactions in neuroimaging. NeuroImage. (1997) ;3: :218–29. |
[24] | McCandliss B , Cohen L , Dehaene S . The visual word form area: expertise for reading in the fusiform gyrus. Trends in Cognitive Sciences. (2003) ;7: (7):293–9. |
[25] | Cohen L , Dehaene S . Specialization within the ventral stream: the case for the visual word form area. NeuroImage. (2004) ;22: (1):466–76. |
[26] | Cohen L , Dehaene S , Naccache L , Lehericy S , Dehaene-Lambertz G , Henaff M , et al. The visual word form area: spatial and temporal characterization of an initial stage of reading in normal subjects and posterior split-brain patients. Brain. (2000) ;123: (2):291–307. |
[27] | Price C , Wise J , Frackowiak R . Demonstrating the implicit processing of visually presented words and pseudowords. Cerebral Cortex. (1996) ;6: :62–70. |
[28] | Luke K-K , Liu H-L , Wai Y-Y , Wan Y-L , Tan L . Functional anatomy of syntactic and semantic processing in language comprehension. Human Brain Mapping. (2002) ;16: :133–45. |
[29] | Leung H , Skudlarski P , Gatenby J , Peterson B , Gore J . An event-related functional MRI study of the Stroop color word interference task. Cerebral Cortex. (2000) ;10: (6):552–60. |
[30] | Mead L , Mayer A , Bobholz J , Woodley S , Cunningham J , Hammeke T , et al. Neural basis of the Stroop interference task: response competition or selective attention? Journal of the International Neuropsychological Society. (2002) ;8: (6):735–42. |
[31] | Melcher T , Gruber O . Oddball and incongruity effects during Stroop task performance: a comparative fMRI study on selective attention. Brain Res. (2006) ;1121: (1):136–49. |
[32] | Corbetta M , Akbudak E , Conturo T , Snyder A , Ollinger J , Drury H , et al. A common network of functional areas for attention and eye movements. Neuron. (1998) ;21: (4):761–73. |
[33] | Voss M , Chaddock L , Kim J , VanPatter M , Pontiflex M , Raine L , et al. Aerobic fitness is associated with greater efficiency of the network underlying cognitive control in preadolescent children. Neuroscience. (2011) ;199: :166–76. |
[34] | Kim C , Chung C , Kim J . Multiple cognitive control mechanisms associated with the nature of conflict. Neurosci Lett. (2010) ;476: (3):156–60. |
[35] | Prabhakaran V , Rypma B , Narayanan NS , Meier TB , Austin BP , Nair VA , et al. Capacity-speed relationships in prefrontal cortex. PLoS One. (2011) ;6: (11):e27504. |
[36] | Rypma B , Berger JS , Prabhakaran V , Bly BM , Kimberg DY , Biswal BB , et al. Neural correlates of cognitive efficiency. Neuroimage. (2006) ;33: (3):969–79. |
[37] | Rypma B , Prabhakaran V . When less is more and when more is more: The mediating roles of capacity and speed in brain-behavior efficiency. Intelligence. (2009) ;37: (2):207–22. |
[38] | Geerligs L , Saliasi E , Maurits N , Lorist M . Compensation through increased functional connectivity: neural correlates of inhibition in old and young. J Cogn Neurosci. (2012) ;24: (10):2057–69. |
[39] | Geerligs L , Rubinov M , Henson R . State and trait components of functional connectivity: individual differences vary with mental state. Journal of Neuroscience. (2015) ;35: (41):13949–61. |
[40] | Solbakk A , Alpert G , Furst A , Hale L , Oga T , Chetty S , et al. Altered prefrontal function with aging: Insights into age-associated performance decline. Brain Res. (2008) ;1232: :30–47. |
[41] | Miller B , D’Esposito M . Searching for “the top” in top-down control. Neuron. (2005) ;48: (4):535–8. |
[42] | Egner T , Delano M , Hirsch J . Separate conflict-specific cognitive control mechanisms in the human brain. NeuroImage. (2007) ;35: (2):940–8. |
[43] | Milham MP , Banich MT , Webb A , Barad V , Cohen NJ , Wszalek T , et al. The relative involvement of anterior cingulate and prefrontal cortex in attentional control depends on nature of conflict. Cognitive Brain Research. (2001) ;12: (3):467–73. |
[44] | Cotman C , Berchtold N . Exercise: A behavioral intervention to enhance brain health and plasticity. Trends in Neurosciences. (2002) ;25: (6):295–301. |
[45] | Patten AR , Yau SY , Fontaine CJ , Meconi A , Wortman RC , Christie BR . The Benefits of Exercise on Structural and Functional Plasticity in the Rodent Hippocampus of Different Disease Models. Brain plasticity (Amsterdam, Netherlands). (2015) ;1: (1):97–127. |
[46] | Hayes SM , Hayes JP , Cadden M , Verfaellie M . A review of cardiorespiratory fitness-related neuroplasticity in the aging brain. Front Aging Neurosci. (2013) ;5: :31. |
[47] | Reiter K , Nielson K , Smith T , Weiss L , Alfini A , Smith J . Improved cardiorespiratory fitness is associated with increased cortical thickness in Mild Cognitive Impairment. Journal of the International Neuropsychological Society. (2015) ;21: (10):757–67. |
[48] | Erickson KI , Voss M , Prakash R , Basak C , Szabo A , Chaddock L , et al. Exercise training increases size of hippocampus and improves memory. Proceedings of the National Academy of Sciences. (2011) ;108: (7):3017–22. |
[49] | Voss MW , Vivar C , Kramer AF , van Praag H . Bridging animal and human models of exercise-induced brain plasticity. Trends Cogn Sci. (2013) ;17: (10):525–44. |
[50] | Smith PJ , Blumenthal JA , Hoffman BM , Cooper H , Strauman TA , Welsh-Bohmer K , et al. Aerobic exercise and neurocognitive performance: a meta-analytic review of randomized controlled trials. Psychosom Med. (2010) ;72: (3):239–52. |
[51] | Northey JM , Cherbuin N , Pumpa KL , Smee DJ , Rattray B . Exercise interventions for cognitive function in adults older than 50: a systematic review with meta-analysis. Br J Sports Med. (2018) ;52: (3):154–60. |
[52] | Barha CK , Davis JC , Falck RS , Nagamatsu LS , Liu-Ambrose T . Sex differences in exercise efficacy to improve cognition: A systematic review and meta-analysis of randomized controlled trials in older humans. Frontiers in neuroendocrinology. (2017) ;46: :71–85. |
[53] | Chirles TJ , Reiter K , Weiss LR , Alfini AJ , Nielson KA , Smith JC . Exercise Training and Functional Connectivity Changes in Mild Cognitive Impairment and Healthy Elders. J Alzheimers Dis. (2017) ;57: (3):845–56. |
[54] | Flodin P , Jonasson LS , Riklund K , Nyberg L , Boraxbekk CJ . Does Aerobic Exercise Influence Intrinsic Brain Activity? An Aerobic Exercise Intervention among Healthy Old Adults. Front Aging Neurosci. (2017) ;9: :267. |
[55] | Li MY , Huang MM , Li SZ , Tao J , Zheng GH , Chen LD . The effects of aerobic exercise on the structure and function of DMN-related brain regions: a systematic review. The International journal of neuroscience. (2017) ;127: (7):634–49. |
[56] | Voss M , Weng T , Burzynska A , Wong C , Cooke G , Clark R , et al. Fitness, but not physical activity, is related to functional integrity of brain networks associated with aging. NeuroImage. (2016) ;131: :113–25. |