Recent advances in LA-ICP-MS for biomedical applications
Abstract
The applications of laser ablation inductively coupled plasma mass spectrometry (LA-ICP-MS) in biomedical research are progressively increasing in the last decade. The main advantage of this powerful analytical technique is the ability to perform multi-elemental analysis directly from biological samples, such as tissues, organs, and single cells, with minimal sample preparation. This feature is particularly important for studies regarding disease biomarkers and mechanism of action of drugs. In this mini-review, the recent advances in the use of LA-ICP-MS for the analysis of biological samples will be discussed, as well as representative biomedical applications.
1.Introduction
Laser ablation inductively coupled plasma mass spectrometry (LA-ICP-MS) is an analytical technique that enables direct multi-elemental analysis and isotope ratio measurements at the trace and ultra trace level in solid samples [3]. Nowadays, it is the most employed technique for mass spectrometry imaging (MSI) of metals, semimetals, and non-metals in biological samples, such as tissues and organs, with a spatial resolution usually in the micrometer range [32].
LA-ICP-MS employs a focused laser beam to ablate material from the surface of a solid sample with a thickness of 5–30 μm [41]. The laser scans the sample line by line, and the material is transported by a continuous flow of argon (or other inert gas, such as helium) to the ICP source [4,14]. The formed ions are continuously extracted from the ion source and further separated according to their mass-to-charge ratio (m/z) in a mass spectrometer, usually equipped with a quadrupole (Q) or double-focusing sector field (SF) mass analyzer. The signal intensities of each ion are thereafter correlated to their concentration. Figure 1 illustrates the major components of a LA-ICP-MS instrument.
Fig. 1.
Schematic diagram of a LA-ICP-MS instrument.
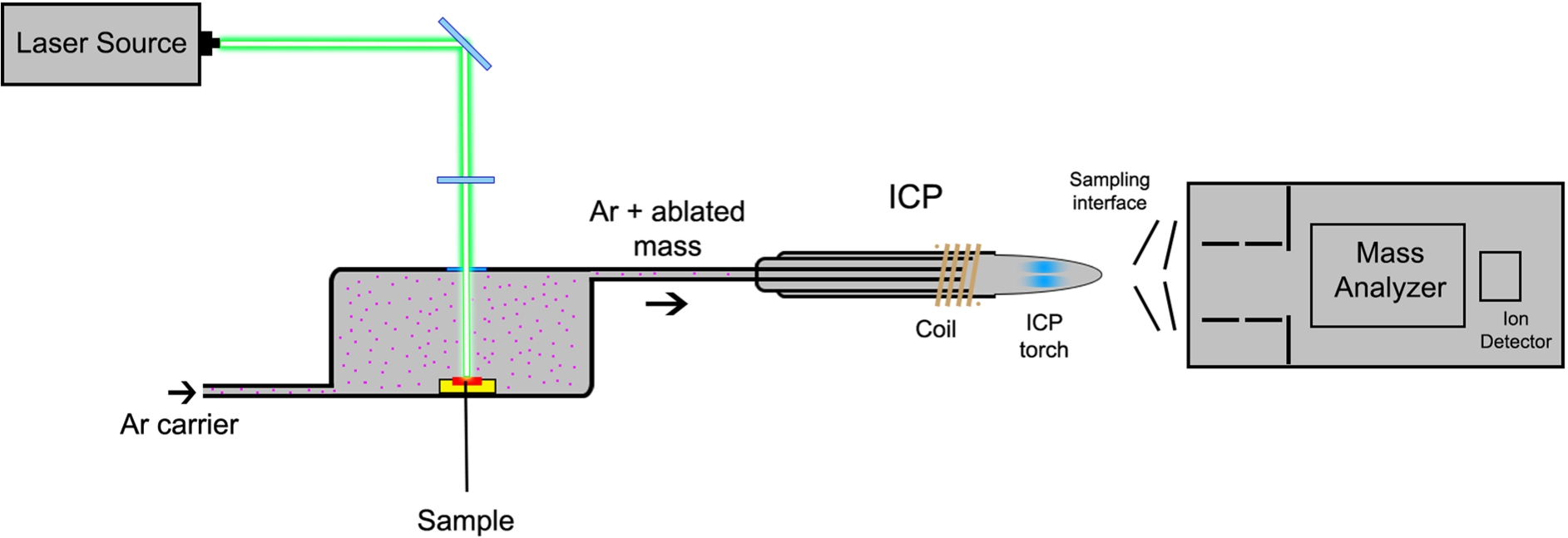
The key parameters that have to be optimized in a LA-ICP-MS experiment are laser spot size, scan speed, and repetition frequency. These parameters influence the ablation efficiency and therefore the sensitivity of the measurements [32].
The precision and accuracy of LA-ICP-MS measurements are directly dependent on the calibration, standardization, and normalization steps. In the case of biological samples, certified reference standards are usually not commercially available, which requires the preparation of laboratory prepared matrix-matched standards (a homogenized tissue spiked with different concentrations of aqueous standards from the analytes) for calibration and standardization [35]. For signal normalization, the measurement of surrogate markers of slice thickness, such as carbon or sulfur, or internal standards is necessary. The most common normalization strategies are based on the measurement of one isotope, the total ion current (TIC), or selected isotopes that represent the ion current related to the sample matrix (extracted ion current, EIC) [17].
LA-ICP-MS can be operated in three different modes: imaging, line scan, and micro local analysis of selected spots [33]. When LA-ICP-MS is used in the imaging mode, the reconstruction of images for visualization of elemental distribution in the analyzed sample is also an important step that will guide the interpretation of the biological question under investigation. Different software tools can be used with this purpose, from commercial to open source ones, such as ELAI [36] and LA-iMageS [27].
LA-ICP-MS has been applied to obtain quantitative images of regionally specific element distributions in sections of different biological tissues, such as mouse brain, kidneys, among others [28,34] as reviewed in the literature [5,6,32]. In the following sections, the recent developments in LA-ICP-MS technique and selected biomedical applications will be discussed.
2.Developments in LA-ICP-MS for bioanalysis
Multimodal imaging, combining LA-ICP-MS with other imaging techniques, is an analytical strategy of growing interest, since it combines the elemental distribution information with structural features, thus improving data that support unraveling complex biological processes.
Recently, Ackerman et al. [1] combined rapid metal profiling by LA-ICP-MS with high-resolution metal imaging by nanoSIMS and with electron and fluorescence confocal microscopies in order to identify tissues and structures with altered copper levels in the Calamitygw71 zebrafish model of Menkes disease. LA-ICP-MS identified reduced copper levels in the brain, neuroretina, and liver of Menkes fish, while high-resolution nanoSIMS imaging of the neuroretina, combined with electron and confocal microscopies, identified the megamitochondria of photoreceptors as loci of copper accumulation in wildtype fish, with lower levels of megamitochondrial copper in the Menkes fish. Niedzwiecki et al. [29] developed another interesting multimodal imaging application, where LA-ICP-MS elemental imaging was combined with multiplexed immunohistochemistry (IHC) to measure element and protein distributions within tissues in order to assess the spatial associations between metals and markers of inflammation in the placenta. These works demonstrate that the complementary information provided by different imaging approaches are important to a broader understanding of the pathophysiology of diseases.
Direct biofluid analysis by LA-ICP-MS is also a topic being continuously explored. A recent approach for serum analysis by LA-ICP-MS using dried serum spot (DSS) sampling, using only 20 μL of serum, was proposed by Chantada-Vázquez et al. [9]. Direct multi-element determination (Al, Be, Ca, Cu, Fe, K, Li, Mg, Mn, Mo, Na, P, Rb, Se, V, and Zn) in DSS sampled in filter paper discs with 2.7 mm diameter and employing acetic acid as an oxidizing agent (used to remove organic matter from the biological matrix) was achieved at low limits of detection levels. Kysenius et al. [25] developed a microdroplet elemental imaging method, which was optimized for the analysis of only 1 μL of mouse spinal cord lysates. This approach for biofluid analysis is particularly relevant for biomedical applications, especially when the samples are rare and obtained in very small volumes.
In terms of instrumentation, recent improvements in the development of low dispersion ablation cells have significantly enhanced throughput and sensitivity for high-resolution LA-ICP-MS imaging, thus increasing the scope of LA-ICP-MS applications, mainly for single cell analysis and sub-cellular imaging [37,38]. The development of these LA cells combined with the use of time-of-flight (TOF) mass analyzers, instead of the common quadrupole, are indeed relevant for the advance of high-resolution LA-ICP-MS imaging [8].
Nowadays, the current hot topic in the use of LA-ICP-MS in biomedical applications is the analysis of single cells, which will be detailed in the following section.
2.1.Single cell analysis
LA-ICP-MS is being proposed as an elemental microscopy technique, since it has quantitative features when matrix-matched calibration standards are used, besides the ease of sample preparation and multi-elemental detection capabilities with high sensitivity and spatial resolution. For co-localization of cells and analytes, LA-ICP-MS profiles are usually correlated with a bright field image of the cell sample [20] or other imaging approaches, such as fluorescence confocal microscopy [37]; thus, multimodal imaging is widely applied for single cell elemental analysis.
The elemental analysis of single cells can be performed using the cells in solution (SC-ICP-MS); however, this type of analysis normally requires at least thousands of single cells in a sample, which is different from the analysis of single cells by LA-ICP-MS. Nevertheless, LA-ICP-MS presents a limitation due to the insufficient analytical speed of quadrupole mass analyzers, which separate the ions with basis on the sequential scanning of isotopes, thus impeding the determination of more than two isotopes during a transient signal from a single cell [7].
Currently, microscopic targets can be in situ analyzed by LA-ICP-MS with spatial resolution from 100 micrometers to sub-micrometers, which enables single cell analysis on a substrate [38]. Commercial laser-ablation cells usually produce a signal response to a shot in the range from several hundred milliseconds to one second, which allows the determination of several elements in single cells by modern quadrupole ICP-MS under optimal conditions [43].
A limiting point of using LA-ICP-MS at micrometer scale is the reduced laser spot size, which leads to a reduction of ablated sample material and therefore to a decreased number of detectable ions in the analyzed area, thus resulting in a compromise between sensitivity and spot size [20]. Another limitation of the single cell LA-ICP-MS imaging is the depth resolution, since the laser beam penetrates through the entire cell, and the signal from the cytosol is monitored together with the nucleus [39].
In order to overcome this issue, Herrmann et al. [20] optimized staining procedures for single cell analysis by LA-ICP-MS. An iridium intercalator was utilized to stain the cell nuclei and the whole cell was stained by the use of maleimido-mono-amide-DOTA (mDOTA) complexing lanthanide (III) ions. The determination of introduced metals per cell was performed using a matrix-matched calibration approach based on cellulose membranes.
Recently, van Malderen et al. [39] obtained a three-dimensional elemental distribution profile in human cervical carcinoma cells (HeLa). The suspended cells underwent a series of fixation/embedding protocols and were stained with uranyl acetate and an Ir-based DNA intercalator to identify the nuclei of single cells. Micro-computed tomography (μ-CT) was applied to acquire a reference frame of the morphology of the cells and their spatial distribution, allowing LA-ICP-MS 3D imaging at the intracellular level.
Precision, accuracy, and efficiency of current calibration strategies are also an important point to be considered in a single cell LA-ICP-MS experiment [21]. Van Malderen et al. [40] proposed the use of high-density microarray plates for multiple matrix-matched standards. The system was applied for the quantification at the single cell level of copper in the marine microalgae Scrippsiella trochoidea after exposure to this metal.
Single cell analysis by LA-ICP-MS is being widely used for studies involving the exposure to metal nanoparticles. Zheng et al. [2] performed the analysis of silver nanoparticles (AgNPs) in human bronchial epithelial (16HBE) single cells. In this case, single cells were trapped by a polydimethylsiloxane (PDMS) microwell array, where 60% of the microwells contained one single cell and 4% of the microwells contained two cells or more. Then, the cells in the array were analyzed by LA-ICP-MS.
3.Applications to biomedicine
Table 1 summarizes biomedical applications of LA-ICP-MS technique in the last 5 years. The authors would like to apologize for any relevant work not included in this mini-review. As can be noticed from this table, an important number of applications involves neurodegenerative disorders, the distribution of metallodrugs and exposure to nanoparticles. Therefore, some of these applications will be discussed in the following sections.
Table 1
Recent LA-ICP-MS biomedical applications
Case study | Biological sample | Analyzed elements | Reference |
Biodegradation of magnesium alloys | Bone tissue | Mg, Mn, Zn, Zr, Yb | Draxler et al., 2015 [13] |
Distribution of gold nanoparticles | Mouse spleen, liver, kidney and lung tissue | Au | Elci et al., 2016 [15] |
Exposure to gold nanoparticles | Single cells | Mg and Au | Zhang et al., 2016 [42] |
Fetal metal exposure | Placenta | Fe, Mn, Pb, Zn | Niedzwiecki et al., 2016 [29] |
Posttraumatic stress disorder | Rat hippocampus | Zn | Sela et al., 2017 [30] |
Gadofluorine P distribution in myocardial infarction | Myocardium | Gd | Lohöfer et al., 2018 [26] |
Liver iron overload disease | Liver tissue | Mn, S, Fe, Cu, Zn | Kim et al., 2018 [22] |
Menkes disease | Zebrafish | Cu | Ackerman et al., 2018 [1] |
Gadolinium deposition | Human brain | Gd, P | Fingerhut et al., 2018 [16] |
Exposure to silver nanoparticles | Single cells | Ag | Zheng et al., 2019 [43] |
Amyotrophic lateral sclerosis (ALS) | Mouse embryos | Mg, P, S, Mn, Fe, Cu, Zn | Kysenius et al., 2019 [24] |
Interaction of cisplatin with serum albumin | Human serum | Pt | Sullivan et al., 2019 [31] |
Pt distribution after antitumor administration | Mouse tibia bone tissue | Pt | Crone et al., 2019 [11] |
Determination of membranous receptors | Single cells | Y, Tm, Ho, Ir | Van Acker et al., 2019 [37] |
Alzheimer’s disease | Human brain tissue | Fe, Au | Cruz-Alonso et al., 2019 [12] |
Metallodrug analysis | Dried blood spot | Gd | Kröger et al., 2019 [23] |
3.1.Wilson’s disease
Wilson’s disease (WD) is a rare genetic disorder where copper concentration in body tissues, particularly in liver, brain and corneas, becomes excessive. Some common symptoms associated to this disorder include vomiting, weakness and yellowish skin [2]. The treatment of this disorder includes medications like penicillamine (Cuprimine), which remove copper from the body (chelation therapy) by urinary excretion and zinc salts, which prevent the gut absorbing copper from the diet.
LA-ICP-MS has been applied successfully in studies about Wilson’s disease. Hachmöller et al. [18] have developed a method for imaging analysis of paraffin-embedded liver needle, where copper and iron were quantified using an external calibration based on matrix-matched gelatin standards. This method has offered low limits of quantification of 1 and 5 μg g−1 and the samples could be differentiated by the inhomogeneous copper distribution and high copper concentration in the samples with WD. Hachmöller et al. [19] have studied the concentration level of copper, iron and zinc in rat liver samples treated with d-penicilamine (DPA). This study indicates that LA-ICP-MS analysis is an efficient method to monitor the chelation therapy in WD patients.
3.2.Gadolinium distribution
Gadolinium-based contrast agents are widely used for magnetic resonance imaging (MRI) examinations and are recognized for being rapidly excreted and not metabolized. However, recent studies showed that Gd traces persists in human brain. Fingerhut et al. [16] confirmed these reports by using LA-ICP-MS to analyze a human brain sample from a patient that received an enhanced MRI examination using gadodiamide. Gd was detected in the cerebellum, the dentate nucleus (the region with highest gadolinium concentration), the basal ganglia, and in the frontal lobe and pons. Clases et al. [10] studied Gd deposition in a nephrogenic systemic fibrosis (NSF) skin sample and in a cancerous brain sample by LA-ICP-MS/MS using a triple quadrupole (QqQ) as the mass analyzer, which improved image resolution and the limits of detection.
Other approaches for the study of Gd deposition were developed by Löhofer et al. [26], which combined MRI and LA-ICP-MS for the detection and quantification of Gadofluorine P deposition in scar formation and myocardial remodeling in the heart after myocardial infarction. Kröger et al. [23] employed a dried blood spot method to quantify the metallodrugs gadopentetate and gadoterate.
4.Conclusion
LA-ICP-MS has been extensively used in biomedical research, providing multi-elemental information in a diversity of biological samples. Due to the improvement in the instrumentation, notably in the newly developed low dispersion LA cells and the use of TOF or QqQ as mass analyzers, LA-ICP-MS is able to enhance the information regarding the mechanisms of diseases, as well as the biological effects of metallodrugs and metal nanoparticles in a variety of tissues and also in single cells. Multimodal imaging, direct biofluid analysis, and single cell analysis by LA-ICP-MS have been improved in the last few years, which is particularly interesting for biomedical studies.
Acknowledgements
A.S. would like to thank FAPESP (grant number 2018/01525-3) for financial support.
Conflict of interest
The authors have no conflict of interest to report.
References
[1] | C.M. Ackerman, P.K. Weber, T. Xiao, B. Thai, T.J. Kuo, E. Zhang, J. Pett-Ridge and C.J. Chang, Multimodal LA-ICP-MS and nanoSIMS imaging enables copper imaging within photoreceptor megamitochondria in a zebrafish model of Menkes disease, Metallomics 10: ((2018) ), 474–485. doi:10.1039/C7MT00349H. |
[2] | O. Bandmann, K.H. Weiss and S.G. Kaler, Wilson’s disease and other neurological copper disorders, Lancet 14: ((2015) ), 103–113. doi:10.1016/S1474-4422(14)70190-5. |
[3] | J.S. Becker, Inorganic Mass Spectrometry: Principles and Applications, Wiley, Chichester, (2007) . |
[4] | J.S. Becker and N. Jakubowski, The synergy of elemental and biomolecular mass spectrometry: New analytical strategies in life sciences, Chem. Soc. 38: ((2009) ), 1969–1983. doi:10.1039/b618635c. |
[5] | J.S. Becker, A. Matusch and B. Wu, Bioimaging mass spectrometry of trace elements – recent advance and applications of LA-ICP-MS: A review, Anal. Chim. Acta. 835: ((2014) ), 1–18. doi:10.1016/j.aca.2014.04.048. |
[6] | J.S. Becker and D. Salber, BrainMet – bioimaging of metals in brain and metallomics: New mass spectrometric tools in brain research, Trends Anal. Chem. 29: ((2010) ), 966–978. doi:10.1016/j.trac.2010.06.009. |
[7] | O. Borovinskaya, B. Hattendorf, M. Tanner, S. Gschwind and D. Günther, A prototype of a new inductively coupled plasma time-of-flight mass spectrometer providing temporally resolved, multi-element detection of short signals generated by single particles and droplets, J. Anal. At. Spectrom. 28: ((2013) ), 226–233. doi:10.1039/C2JA30227F. |
[8] | M. Burger, G. Schwarz, A. Gundlach-Graham, D. Käser, B. Hattendorf and D. Günther, Capabilities of laser ablation inductively coupled plasma time-of-flight mass spectrometry, J. Anal. At. Spectrom. 32: ((2017) ), 1946–1959. doi:10.1039/C7JA00236J. |
[9] | M.P. Chantada-Vázquez, J. Moreda-Piñeiro, A. Cantarero-Roldán, P. Bermejo-Barrera and A. Moreda-Piñeiro, Development of dried serum spot sampling techniques for the assessment of trace elements in serum samples by LA-ICP-MS, Talanta 186: ((2018) ), 169–175. doi:10.1016/j.talanta.2018.04.049. |
[10] | D. Clases, S. Fingerhut, A. Jeibmann, M. Sperling, P. Doble and U. Karst, LA-ICP-MS/MS improves limits of detection in elemental bioimaging of gadolinium deposition originating from MRI contrast agents in skin and brain tissues, J. Trace. Elem. Med. Biol. 51: ((2019) ), 212–218. doi:10.1016/j.jtemb.2018.10.021. |
[11] | B. Crone, L. Schlatt, R.A. Nadar, N.W.M. van Dijk, N. Margiotta, M. Sperling, S. Leeuwenburgh and U. Karst, Quantitative imaging of platinum-based antitumor complexes in bone tissue samples using LA-ICP-MS, J. Trace Elem. Med. Biol. 54: ((2019) ), 98–102. doi:10.1016/j.jtemb.2019.04.011. |
[12] | M. Cruz-Alonso, B. Fernandez, A. Navarro, S. Junceda, A. Astudillo and R. Pereiro, Laser ablation ICP-MS for simultaneous quantitative imaging of iron and ferroportin in hippocampus of human brain tissues with Alzheimer’s disease, Talanta 197: ((2019) ), 413–421. doi:10.1016/j.talanta.2019.01.056. |
[13] | J. Draxler, A. Zitek, M. Meischel, S.E. Stranzl-Tschegg, B. Mingler, E. Martinelli, A.M. Weinberg and T. Prohaska, Regionalized quantitative LA-ICP-MS imaging of the biodegradation of magnesium alloys in bone tissue, J. Anal. At. Spectrom. 30: ((2015) ), 2459–2468. doi:10.1039/C5JA00354G. |
[14] | S.F. Durrant and N.I. Ward, Recent biological and environmental applications of laser ablation inductively coupled plasma mass spectrometry (LA-ICP-MS), J. Anal. At. Spectrom. 20: ((2005) ), 821–829. doi:10.1039/b502206a. |
[15] | S.G. Elci, B. Yan, S.T. Kim, K. Saha, Y. Jiang, G.A. Klemmer, D.F. Moyano, G.Y. Tonga, V.M. Rotello and R.W. Vachet, Quantitative imaging of 2 nm monolayer-protected gold nanoparticle distributions in tissues using laser ablation inductively-coupled, Analyst 141: ((2016) ), 2418–2425. doi:10.1039/C6AN00123H. |
[16] | S. Fingerhut, A.C. Niehoff, M. Sperling, A. Jeibmann, W. Paulus, T. Niederstadt, T. Allkemper, W. Heindel, M. Holling and U. Karst, Spatially resolved quantification of gadolinium deposited in the brain of a patient treated with gadolinium-based contrast agents, J. Trace Elem. Med. Biol. 45: ((2018) ), 125–130. doi:10.1016/j.jtemb.2017.10.004. |
[17] | J.M. Fonville, C. Carter, O. Cloarec, J.K. Nicholson, J.C. Lindon, J. Bunch and E. Holmes, Robust data processing and normalization strategy for MALDI mass spectrometric imaging, Anal. Chem. 84: ((2012) ), 1310–1319. doi:10.1021/ac201767g. |
[18] | O. Hachmöller, M. Aichler, K. Schwamborn, L. Lutz, M. Werner, M. Sperling, A. Walch and U. Karst, Element bioimaging of liver needle biopsy specimens from patients with Wilson’s disease by laser ablation-inductively coupled plasma-mass spectrometry, J. Trace Elem. Med. Biol. 35: ((2016) ), 97–102. doi:10.1016/j.jtemb.2016.02.001. |
[19] | O. Hachmöller, A. Zibert, H. Zischka, M. Sperling, S.R. Groba, I. Grünewald, E. Wandelmann, H.H.J. Schmidt and U. Karst, Spatial investigation of the elemental distribution in Wilson’ s disease liver after D-penicillamine treatment by LA-ICP-MS, J. Trace Elem. Med. Biol. 44: ((2017) ), 26–31. doi:10.1016/j.jtemb.2017.05.008. |
[20] | A.J. Herrmann, S. Techritz, N. Jakubowski, A. Haase, A. Luch, U. Panne and L. Mueller, A simple metal staining procedure for identification and visualization of single cells by LA-ICP-MS, Analyst 142: ((2017) ), 1703–1710. doi:10.1039/C6AN02638A. |
[21] | J. Jiménez-Lamana, J. Szpunar and R. Łobinski, New frontiers of metallomics: Elemental and species-specific analysis and imaging of single cells, Adv. Exp. Med. Biol. 1055: ((2018) ), 245–270. doi:10.1007/978-3-319-90143-5_10. |
[22] | P. Kim, S. Weiskirchen, R. Uerlings, A. Kueppers, F. Stellmacher, A. Viveiros, H. Zoller and R. Weiskirchen, Quantification of liver iron overload disease with laser ablation inductively coupled plasma mass spectrometry, BMC Med. Imag. 18: ((2018) ), 1–11. doi:10.1186/s12880-018-0245-9. |
[23] | S. Kröger, M. Sperling and U. Karst, Quantitative dried blood spot analysis for metallodrugs by laser ablation- inductively coupled plasma-mass spectrometry, J. Trace Elem. Med. Biol. 51: ((2019) ), 50–56. doi:10.1016/j.jtemb.2018.09.009. |
[24] | K. Kysenius, J.B. Hilton, B. Paul, D.J. Hare and P.J. Crouch, Anatomical redistribution of endogenous copper in embryonic mice overexpressing SOD1, Metallomics 11: ((2019) ), 141–150. doi:10.1039/C8MT00242H. |
[25] | K. Kysenius, B. Paul, J.B. Hilton, J.R. Liddell, D.J. Hare and P.J. Crouch, A versatile quantitative microdroplet elemental imaging method optimised for integration in biochemical workflows for low-volume samples, Anal. Bioanal. Chem. 3: ((2019) ), 603–616. doi:10.1007/s00216-018-1362-6. |
[26] | F. Lohöfer, L. Hoffmann, R. Buchholz, K. Huber, A. Glinzer, K. Kosanke, A. Feuchtinger, M. Aichler, B. Feuerecker, G. Kaissis, E.J. Rummeny, C. Höltke, C. Faber, F. Schilling, R.M. Botnar, A.K. Walch, U. Karst and M. Wildgruber, Molecular imaging of myocardial infarction with Gadofluorine P – A combined magnetic resonance and mass spectrometry imaging approach, Heliyon 4: ((2018) ), e00606. doi:10.1016/j.heliyon.2018.e00606. |
[27] | H. López-Fernández, G.S. Pessôa, M.A.Z. Arruda, J.L. Capelo-Martínez, F. Fdez-Riverola, D. Glez-Peña and M. Reboiro-Jato, LA-iMageS: A software for elemental distribution bioimaging using LA-ICP-MS data, J. Cheminform. 8: ((2016) ), 65. doi:10.1186/s13321-016-0178-7. |
[28] | P. M-M, R. Weiskirchen, N. Gassler, A.K. Bosserhoff and J.S. Becker, Novel bioimaging techniques of metals by laser ablation inductively coupled plasma mass spectrometry for diagnosis of fibrotic and cirrhotic liver disorders, PLoS One 8: ((2013) ), e58702. doi:10.1371/journal.pone.0058702. |
[29] | M.M. Niedzwiecki, C. Austin, R. Remark, M. Merad, S. Gnjatic, G. Estrada-Gutierrez, A. Espejel-Nuñez, H. Borboa-Olivares, M. Guzman-Huerta, R.J. Wright, R.O. Wright and M. Arora, A multimodal imaging workflow to visualize metal mixtures in the human placenta and explore colocalization with biological response markers, Metallomics 8: ((2016) ), 444–452. doi:10.1039/C6MT00010J. |
[30] | H. Sela, H. Cohen, Z. Karpas and Y. Zeiri, Distinctive hippocampal zinc distribution patterns following stress exposure in an animal model of PTSD, Metallomics 9: ((2017) ), 323–333. doi:10.1039/C6MT00207B. |
[31] | M.P. Sullivan, S.J. Morrow, D.C. Goldstone and C.G. Hartinger, Gel electrophoresis in combination with laser ablation – inductively coupled plasma mass spectrometry to quantify the interaction of cisplatin with human, Electrophoresis, in press. doi:10.1002/elps.201900070. |
[32] | A. Sussulini, J.S. Becker and J.S. Becker, Laser ablation ICP-MS: Application in biomedical research, Mass Spectrom. Rev. 36: ((2017) ), 47–57. doi:10.1002/mas.21481. |
[33] | A. Sussulini, H. Kratzin, O. Jahn, C.E.M. Banzato, M.A.Z. Arruda and J.S. Becker, Metallomics studies of human blood serum from treated bipolar disorder patients, Anal. Chem. 82: ((2010) ), 5859–5864. doi:10.1021/ac101063t. |
[34] | A. Sussulini, A. Matusch, M. Klietz, A. Bauer, C. Depboylu and J.S. Becker, Quantitative imaging of Cu, Fe, Mn and Zn in the L-DOPA-treated unilateral 6-hydroxydopamine Parkinson’s disease mouse model by LA-ICP-MS, Biomed. Spectroscopy Imaging 1: ((2012) ), 125–136. doi:10.3233/BSI-2012-0011. |
[35] | R. Uerlings, A. Matusch and R. Weiskirchen, Standardization and normalization of data from laser ablation inductively coupled plasma mass spectrometry, in: Applications of Laser Ablation – Thin Film Deposition, Nanomaterial Synthesis and Surface Modification, D. Yang, ed., Intech Pub., (2016) , pp. 399–415. |
[36] | R. Uerlings, A. Matusch and R. Weiskirchen, Reconstruction of laser ablation inductively coupled plasma mass spectrometry (LA-ICP-MS) spatial distribution images in Microsoft Excel 2007, Int. J. Mass. Spectrom. 395: ((2016) ), 27–35. doi:10.1016/j.ijms.2015.11.010. |
[37] | T. van Acker, T. Buckle, S.J.M. van Malderen, D.M. van Willigen, V. van Unen, F.W.B. van Leeuwen and F. Vanhaecke, High-resolution imaging and single-cell analysis via laser ablation inductively coupled plasma-mass spectrometry for the determination of membranous receptor expression levels in breast cancer cell lines using receptor-specific hybrid tracers, Anal. Chim. Acta 1074: ((2019) ), 43–53. doi:10.1016/j.aca.2019.04.064. |
[38] | S.J.M. van Malderen, A.J. Managh, B.L. Sharp and F. Vanhaecke, Recent developments in the design of rapid response cells for laser ablation-inductively coupled plasma-mass spectrometry and their impact on bioimaging applications, J. Anal. At. Spectrom. 31: ((2016) ), 423–439. doi:10.1039/C5JA00430F. |
[39] | S.J.M. van Malderen, T. van Acker, B. Laforce, M. De Bruyne, R. de Rycke, T. Asaoka, L. Vincze and F. Vanhaecke, Three-dimensional reconstruction of the distribution of elemental tags in single cells using laser ablation ICP-mass spectrometry via registration approaches, Anal. Bioanal. Chem. 411: ((2019) ), 4849–4859. doi:10.1007/s00216-019-01677-6. |
[40] | S.J.M. van Malderen, E. Vergucht, M. De Rijcke, C. Janssen, L. Vincze and F. Vanhaecke, Quantitative determination and subcellular imaging of Cu in single cells via laser ablation-ICP-mass spectrometry using high-density microarray gelatin standards, Anal. Chem. 88: ((2016) ), 5783–5789. doi:10.1021/acs.analchem.6b00334. |
[41] | S. Weiskirchen, P. Kim and R. Weiskirchen, Determination of copper poisoning in Wilson’s disease using laser ablation inductively coupled plasma mass spectrometry, Ann. Transl. Med. 7: ((2019) ), S72. doi:10.21037/atm.2018.10.67. |
[42] | Z. Xin-Ying, Z. Ling-Na, W. Hai-Long, S. Jun-Wen, F. Wei-Yue, L. Liang and W. Meng, Elemental bio-imaging of biological samples by laser ablation – inductively coupled plasma – mass spectrometry, Chin. J. Anal. Chem. 44: ((2016) ), 1646–1651. doi:10.1016/S1872-2040(16)60969-6. |
[43] | L.N. Zheng, Y.B. Sang, R.P. Luo, B. Wang, F.T. Yi, M. Wang and W.Y. Feng, Determination of silver nanoparticles in single cells by microwell trapping and laser ablation ICP-MS determination, J. Anal. At. Spectrom. 34: ((2019) ), 915–921. doi:10.1039/C8JA00438B. |