Neural Oscillations and Functional Significances for Prioritizing Dual-Task Walking in Parkinson’s Disease
Abstract
Background:
Task prioritization involves allocating brain resources in a dual-task scenario, but the mechanistic details of how prioritization strategies affect dual-task walking performance for Parkinson’s disease (PD) are little understood.
Objective:
We investigated the performance benefits and corresponding neural signatures for people with PD during dual-task walking, using gait-prioritization (GP) and manual-prioritization (MP) strategies.
Methods:
Participants (N = 34) were asked to hold two inter-locking rings while walking and to prioritize either taking big steps (GP strategy) or separating the two rings (MP strategy). Gait parameters and ring-touch time were measured, and scalp electroencephalograph was performed.
Results:
Compared with the MP strategy, the GP strategy yielded faster walking speed and longer step length, whereas ring-touch time did not significantly differ between the two strategies. The MP strategy led to higher alpha (8–12 Hz) power in the posterior cortex and beta (13–35 Hz) power in the left frontal-temporal area, but the GP strategy was associated with stronger network connectivity in the beta band. Changes in walking speed and step length because of prioritization negatively correlated with changes in alpha power. Prioritization-related changes in ring-touch time correlated negatively with changes in beta power but positively with changes in beta network connectivity.
Conclusions:
A GP strategy in dual-task walking for PD can enhance walking speed and step length without compromising performance in a secondary manual task. This strategy augments attentional focus and facilitates compensatory reinforcement of inter-regional information exchange.
INTRODUCTION
Impaired walking ability is prevalent in individuals with Parkinson’s disease (PD), particularly when performing concurrent tasks [1]. Frontostriatal dysfunction in PD leads to compromised executive function, which hinders the ability to process multiple activities simultaneously [2]. The gait performance of individuals with PD is adversely affected when performing concurrent motor or cognitive tasks, a condition known as dual-task walking [3]. This often results in reduced walking speed, shorter step length, increased cadence (to compensate for reduced step length), and heightened gait variability [4]. Additionally, this dual-task interference tends to be more pronounced, particularly in the off-medication state [5]. During dual-task scenarios, patients are susceptible to falling because of their inability to solve task interference and attend to environmental cues [1]. In addition to deficits in balance and gait control, inappropriate allocation of attention has been identified as a critical factor of falls in dual-task conditions, particularly among older adults with balance or gait impairment [4, 6]. Research indicates that in a posture dual-task scenario, where individuals are engaged in dual-task standing, healthy adults can improve stance stability by enhancing automaticity through allocating more attention to the suprapostural task [7–10]. Akin to healthy adults, individuals with PD are recommended to adopt a posture-second strategy, prioritizing attention on the suprapostural task rather than standing balance. This approach aims to enhance stance stability and improve suprapostural accuracy during a posture dual-task [11–13]. In contrast to dual-task standing scenarios, healthy young and older adults tend to prioritize gait control during dual-task walking, which allows them to walk faster without sacrificing the performance of concurrent motor (e.g., tray stability) or cognitive (e.g., verbal fluency) tasks [14, 15]. While individuals with PD are often advised to prioritize gait during dual-task walking [16, 17], such as emphasizing larger steps [18–20], this strategy may lead to a decline in suprapostural performance due to limited functional reserve and attentional capacity. For example, worse performance of concurrent motor (e.g., tray stability) and cognitive (e.g., Stroop task latency and calculation accuracy) tasks were observed when PD focused on walking than focused on the suprapostural task in dual-task walking [18, 21, 22]. Therefore, it remains inconclusive how dual-task walking is controlled when individual with PD adopt different task prioritization strategies. In particular, knowledge is limited about how task prioritization affects the cortical activation in PD.
According to the multiple resource theory, cortical activation can vary based on task priority to manage task interference and resource competition, as these tasks share the processes of perception, cognition, and responding [23]. The prefrontal and frontal cortices are responsible for cognitive resource allocation during dual-task execution [24–26]. In one study of young adults, increasing task difficulty of the alphabetic N-back led to involuntary acceleration of paced ankle movements [27]. Prioritization of the cognitive demands (the N-back task) in that study was associated with reduced regional activities in the left inferior frontal gyrus and superior parietal lobule. In another study of individuals with neuropathic gait abnormalities, performing a cognitive task while walking and shifting attention to walking increased prefrontal activation for gait compensation; however, performance in reciting alternate alphabets worsened [28]. Zhang et al. proposed that the posture-first strategy in dual-task walking with a mathematical calculation subtask led to less left parietal lobe activity in people with PD, despite no significant performance difference compared to the posture-second strategy [29].
In addition to regional activity, cortical oscillations in the theta, alpha and beta bands might be modulated depending on task prioritization because they are influenced by cueing strategies [30, 31] and selective attention [32, 33]. Cortical theta power is associated with cognitive workload [34]. Aligned with increased postural sway, higher theta power signifies an augmented information processing load for postural error detection [35]. Regarding alpha power and beta power, Tosserams et al. (2022) found that both rhythms decreased around the sensorimotor area, when individuals with PD walked with external or internal cueing compared with walked without cueing [31]. The reduction in alpha and beta power may suggest that paying attention to walk could lessen the demands on sensory-cognitive processes and motor attention in individuals with PD [36, 37]. Moreover, the prioritization effect might be mediated by interplays between spatially distinct areas in the brain (e.g., brain connectivity). Huang et al. reported that during a narrow stance with a concurrent motor task, individuals with PD showed attenuation of prioritization-related modulation in EEG-EEG connectivity across the fronto-central-parietal area [12]. However, this finding of a prioritization effect on dual-task standing could not be generalized to dual-task walking, because the prioritization effect in a dual task is highly task-dependent. In conjunction with improved gait, the enhanced beta connectivity in the fronto-centroparietal and fronto-temporal areas suggests that directing attention to regulate gait rhythmicity with auditory stimulations may strengthen the motoric status quo and serve as a controller for walking in individuals with PD [30, 38].
Despite several lines of behavioral evidence, no previous work has investigated cortical mechanisms (both regional and inter-regional activities) and the corresponding functional significance of task prioritization during dual-task walking in PD. Here we compared EEG regional power and EEG-EEG connectivity in the theta, alpha, and beta bands between gait-prioritization (GP) and manual-prioritization (MP) strategies in people with PD during dual-task walking. In addition, we investigated the correlation of changes in EEG spectral variables related to prioritization and dual-task performance. We hypothesized that (1) the GP strategy would lead to better walking performance in individuals with PD, characterized by decreased regional activities in all bands and enhanced beta inter-regional connectivity; and (2) the benefits of dual-task prioritization in PD would be associated with specific changes in EEG regional activity and inter-regional connectivity within each band.
METHODS
Participants
Thirty-four people with PD (mean age: 65.0±5.8 years) participated in this study. The inclusion criteria were (1) a diagnosis of idiopathic PD according to the United Kingdom PD Society Brain Bank clinical diagnostic criteria [39], (2) PD onset at age ≥40 years, (3) a Mini-Mental State Examination score > 25, and (4) ability to walk 10 m without a walking aid or help from another person when off medication. Patients were excluded if they had a history of brain surgery (e.g., deep brain stimulation) or other diseases and conditions that could influence balance ability or had moderate or severe postural tremor or kinetic tremor in the hands (to prevent a possible effect on the manual task). Tremor severity was defined by a score > 2 on item 3.15 (postural tremor) or 3.16 (kinetic tremor) in the Movement Disorder Society-sponsored revision of the Unified Parkinson’s Disease Rating Scale [40]. Table 1 presents the demographic data and clinical characteristics of the participants. All procedures in this study were approved by the National Taiwan University Hospital Research Ethics Committee (Clinical Trial Registration No.: NCT03298503), and all participants gave written informed consent. The sample size was calculated based on data from a previous study [21], which indicated that 16 participants would be sufficient to detect the effect of task prioritization on dual-task walking (effect size d = 0.87, power = 0.9,α= 0.05).
Table 1
Patient demographics and characteristics (N = 34)
Participant details | |
Age (y) | 65.0±5.8 |
Age range (y) | 55.6–74.9 |
Sex, M/F (n) | 17/17 |
Freezer/Non-freezer (n) | 17/17 |
Disease duration (y) | 5.9±2.7 |
Modified Hoehn and Yahr stage (off state) | 2.4±0.5 |
MMSE (off state) | 28.5±1.2 |
MDS-UPDRS motor scores (off state) | 33.3±9.8 |
Data are mean±standard deviation unless otherwise indicated. Freezer/Non-freezer, The participants who ever experienced “freezing of gait episodes” over the past month before recruitment into the study were categorized as freezers. The participants who did not experience “freezing of gait episodes” over the past month before recruitment into the study were categorized as non-freezers. MMSE, Mini-Mental Status Examination; MDS-UPDRS, Movement Disorder Society Unified Parkinson’s Disease Rating Scale.
Experimental conditions and data recording
All clinical assessments and dual-task walking examinations were performed in the morning, at least 12 h after the most recent administration of anti-parkinsonian medications (i.e., medication-off testing). For the dual-task walking, participants were instructed to walk forwardly on an electronic walkway (GAITRite, CIR Systems Inc., Franklin, NJ, USA; sampling rate: 100 Hz) while controlling a pair of inter-locking rings (Fig. 1). To stabilize gait measures with the GAITRite walkway (length: 5.20 m; active area: 4.27 m), the participants walked 2 m before and after the walkway for acceleration and deceleration. For the ring task, the participants flexed elbows at 90°, carefully preventing ring touching while holding two sticks with inter-locking rings (diameter: 4 cm) on the top during walking [20, 37]. The ring task has been proposed as a motor task with relatively low physical demand [37]. If the two rings touched, the computer recorded the event with an A/D card (USB-6221, National Instruments, Austin, TX, USA; sampling rate: 1 kHz). All participants performed the GP condition and MP condition in a random sequence. In the GP condition, the participants were instructed as follows: “The walking task is the primary task. Pay most attention to taking big steps during walking and then to preventing the two rings from touching if possible”. In the MP condition, the participants were instructed as follows: “The ring task is the primary task. Pay most attention to preventing the two rings from touching and then to taking big steps while walking if possible”. To minimize visual feedback from the gait or ring task, the participants were asked to look straight ahead and not at their feet or the rings during walking. Two practice trials were conducted before eight testing trials in each experimental condition. The resting time was 30 s between two testing-trails and was 1 min between the GP and MP conditions for avoiding the effect of muscle fatigue. Immediately following each test trial, the participants were asked to rate the percentage of their attention that they felt had been directed towards “walking with taking big steps” and towards “preventing the two rings from touching”, using an analogue scale (0%–100%) [18].
Fig. 1
Schematic representation of the experimental setup.
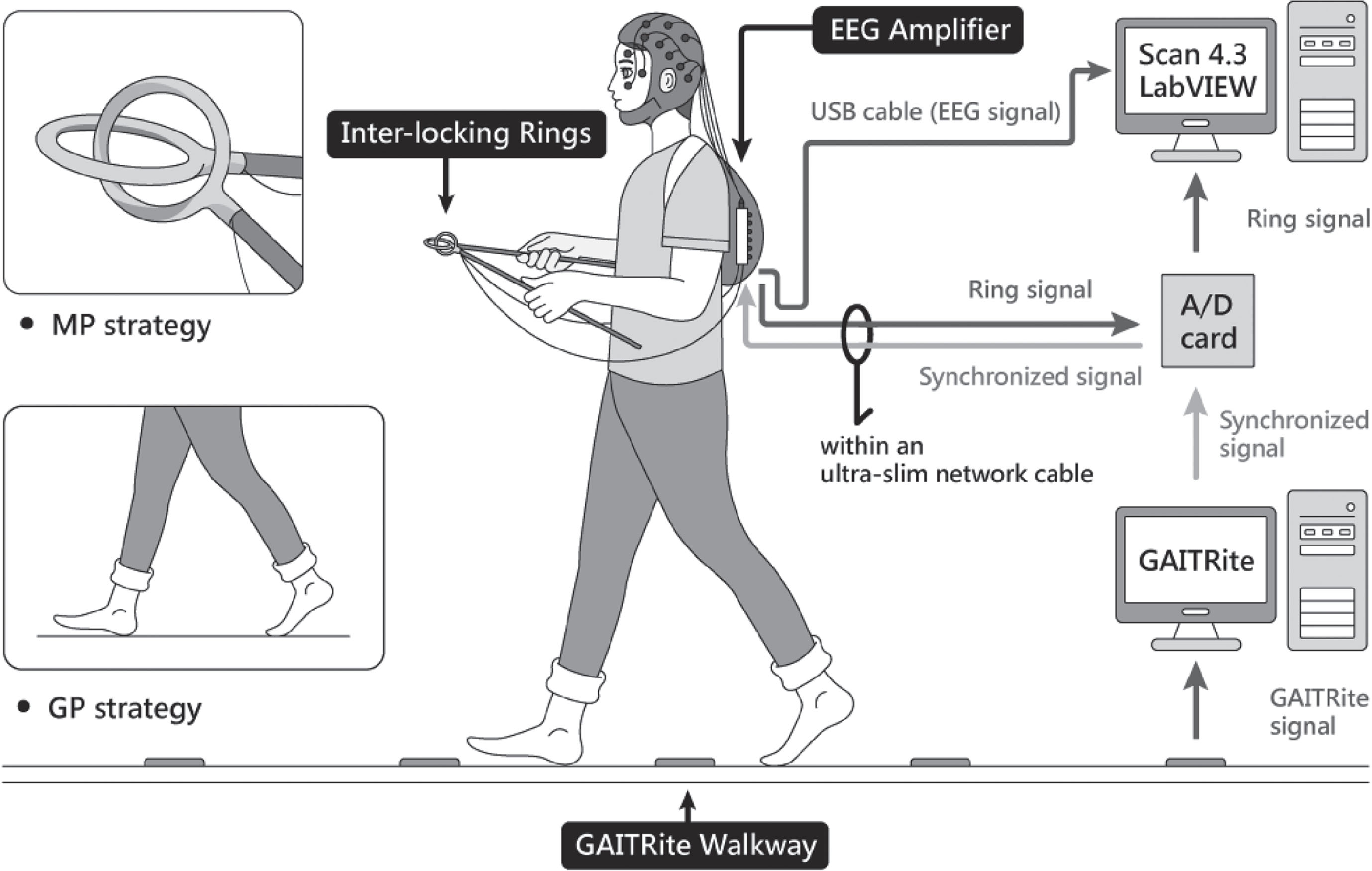
Cortical activities were recorded using a NuAmps 32-channel amplifier (NeuroScan Inc., El Paso, TX, USA). The EEG electrodes (Fp1/2, Fz, F3/4, F7/8, FT7/8, FCz, FC3/4, Cz, C3/4, CPz, CP3/4, Pz, P3/4, T3/4, T5/6, TP7/8, Oz, and O1/2) were placed based on the 10–20 electrode system of the International Federation. The ground electrode was placed along the midline, ahead of Fz. During walking, participants carried the EEG amplifier in a backpack with proper fixation as with a mobile EEG system. The wires between the EEG cap and amplifier were fixed on the upper back by several strips of adhesive tape to avoid artifacts caused by the movement of EEG wires. The cable between the amplifier and computer was suspended to avoid interfering with walking. To monitor vertical and horizontal eye movements and blinks, electrodes were placed above the left eyebrow, below the left eye, and horizontally on the outer canthi of both eyes. The impedances of all electrodes were below 5 kΩ, and all electrodes were referenced to linked mastoids of both sides. EEG data were band-pass filtered at 0.1–100 Hz with a 1 kHz sampling rate. All behavioral data and EEG data were synchronized.
Data analysis
At the behavior level, speed (cm/s), cadence (steps/min), step length (cm), and the coefficient of variation (CV, %) of step length were calculated for the walking task. For the suprapostural task (ring task), ring-touch time (%) was calculated as the percentage of time in which the rings were touching in a walking trial.
At the cortical level, the EEG data were first filtered between 4 and 35 Hz using a zero-phase finite impulse response filter (60 dB/octave) to remove the DC shift and potential contamination from low-frequency movement artifacts < 4 Hz. A zero-phase finite impulse response band-pass filter (60 dB/octave) was selected because the filter setting is phase-invariant (FIR filter) with a sufficiently high slope to exclude EEG signals outside the range of interest (< 4 Hz and > 35 Hz) [41]. Local cortical activity and inter-regional connectivity at theta (4–7 Hz), alpha (8–12 Hz), and beta (13–35 Hz) were characterized. EEG data > 35 Hz (e.g., the gamma band) were not analyzed because of possible contamination from muscle activity of the head and neck [42]. The eye movement or blink artifacts were removed from the EEG using regression analysis with the NeuroScan 4.3 software program (NeuroScan Inc., El Paso, TX, USA), based on bipolar vertical and horizontal electrooculogram channels [43, 44]. Afterwards, the conditioned EEG data were segmented into epochs of a gait cycle (roughly 1–2 s). The EEG epochs were time-locked to single gait cycles, specifically from left heel strike to left heel strike or right heel strike to right heel strike, depending on the foot of the first heel strike recorded by the GAITRite electronic walkway. An experienced researcher visually inspected each epoch of a gait cycle to reject any remaining artifacts.
In the present study, each test trial contained 4–6 artifact-free epochs (32–48 epochs per condition). The spectral power of an epoch was calculated based on Fast Fourier Transformation with a frequency resolution of 0.2 Hz. The relative power of the epoch was computed as the ratio of the power of each spectral value to the total power, and relative power spectra were averaged across epochs for all channels. The mean value of relative power in each sub-band was obtained from the experimental trials in the GP and MP conditions. For each sub-band EEG, the inter-regional EEG connectivity of the 30 electrode pairs was estimated with the weighted phase-lag index (wPLI). This index is an extended version of the phase-lag index that maximizes the weight of±90-degree phase differences while uniformly driven (such as volume conduction) sources are effectively suppressed [45]. wPLI has been recommended for measuring EEG activity during locomotion because of its immunity to movement artifacts [46]. The wPLI weights each phase difference according to the magnitude of the lag, mathematically formulated as:
A square 30×30 wPLI-adjacent matrix of each epoch in an experimental trial for the GP and MP conditions was obtained and averaged. The wPLI of EEG electrode pairs was calculated with the HERMES function in Matlab [47]. A paired t-test was used to examine differences in the wPLI between the electrode pairs (a cell within a 30×30 matrix) between the GP and MP conditions. The resulting 30×30 t matrix represented prioritization-related differences in inter-regional connectivity between the GP and MP conditions. Supra-threshold connectivity was denoted as a high prioritization-related difference in wPLI for the two prioritization conditions (|t33| > 2.733, p < 0.005). Supra-threshold connectivity was used to highlight topological differences in EEG networks between the GP and MP conditions.
Statistical analysis
Repeated two-way analysis of variance was used to compare the amount of attention directed towards the walking task and ring task between the GP and MP conditions. Paired t-tests were used to examine the effect of prioritization strategy on behavior performance and EEG metrics between the two conditions, including all gait parameters, ring-touch time, and mean relative power of the electrodes in the regions of interest. For EEG relative power, if the number of regions of interest was more than one, the Bonferroni correction would be used for multiple comparisons.
To compare differences in sub-band EEG network properties, a set of prioritization-dependent supra-threshold connectivities was mapped on the scalp. A permutation test was performed 5000 times to examine variations in the null distribution of the supra-threshold connectivity between the GP and MP conditions. Methodological details of corrected network-based statistics with the permutation test have been documented in the work of Zalesky et al. [48]. In case of a prioritization-related difference in the sub-band EEG network, mean wPLI (m-wPLI) with supra-threshold connectivity was obtained for the GP and MP conditions, and the mean strength of wPLI of the two prioritization conditions was compared with the paired t-test. To bridge behavior and EEG linkage with prioritization changes, we calculated the normalized difference (ND) in gait, ring touch, relative power of EEG electrodes in the regions of interest, and m-wPLI between the GP and MP conditions (
RESULTS
All participants completed the experiment without experiencing any adverse effects or falls. The results of repeated two-way analysis of variance revealed that the participants allocated greater attention to the primary task compared with the secondary task in both the GP and MP conditions (F1,33 = 772.258, p < 0.001). There was no significant difference between the conditions in terms of task prioritization (F1,33 < 0.001, p = 1.000). In the GP condition, participants reported allocating 74.0% ±6.0% of their total attention to “walking with taking big steps” and 26.0% ±6.0% to “preventing the two rings from touching”, as indicated by the analogue scale. In the MP condition, participants allocated 73.1% ±5.6% of their total attention to “preventing the two rings from touching” and 26.9% ±5.6% to “walking with taking big steps”.
Table 2 presents the means and standard deviations for the behavioral performance of dual-task walking with GP and MP strategies. Results of paired t-tests indicated that using the GP strategy resulted in significantly faster walking speed (t33 = 3.775, p = 0.001, d = 0.647) and longer step length (t33 = 5.461, p < 0.001, d = 0.937) compared with using the MP strategy. However, the prioritization effect did not significantly influence cadence, step length CV, or ring-touch time (p > 0.05).
Table 2
Means and standard deviations of performance of dual-task walking with gait-prioritization (GP) and manual-prioritization (MP)
GP | MP | Statistics | |
Posture | |||
Speed (cm/s) | 78.53 ± 12.67 | 71.56 ± 12.47 | t33 = 3.775, p = 0.001 |
Cadence (step/min) | 89.55±11.34 | 92.00±13.72 | t33 = –1.517, p = 0.139 |
Step length (cm) | 51.66 ± 8.65 | 45.55 ± 8.77 | t33 = 5.461, p < 0.001 |
Step length CV (%) | 5.67±2.35 | 6.42±3.07 | t33 = –1.506, p = 0.142 |
Supraposture | |||
Ring-touch Time (%) | 3.81±4.19 | 3.09±2.75 | t33 = 1.322, p = 0.192 |
CV, coefficient of variation.
Figure 2 displays the pooled topological distributions of relative powers of theta, alpha, and beta waves from scalp EEG between the GP and MP conditions during dual-task walking. In theta power, prioritization-dependent differences were observed in the prefrontal-frontal (Fp1, Fp2, F3, Fz, and FCz), sensorimotor (C3, Cz, C4, and CP3), and right parietal-occipital (P4 and O2) areas (p < 0.05). Because there were three regions of interest, the significance of the paired-t test for task prioritization effect in theta power was p = 0.017 using the Bonferroni correction. Paired t-tests showed the mean amplitudes of the theta relative power were generally smaller in the GP compared with the MP condition in these regions (prefrontal-frontal: t33 = –3.386, p = 0.002, d = 0.581; sensorimotor: t33 = –2.856, p = 0.007, d = 0.490; right parietal-occipital: t33 = –3.355, p = 0.002, d = 0.575). For alpha relative power, widespread prioritization-dependent differences were found in the posterior cortex (all EEG electrodes except for Fp1, Fp2, F7, F3, and F8; p < 0.05). The mean value of alpha relative power was smaller in the GP compared with the MP condition (t33 = –3.633, p = 0.001, d = 0.623). In the beta band, prioritization-dependent differences were observed in the left frontal-temporal area (F7, F3, FT7, and T3) and O2 electrode (p < 0.05). Because there were two regions of interest, the significance of the paired-t test for task prioritization effect in theta power was p = 0.025 using the Bonferroni correction. The mean value of beta power in the left frontal-temporal area was smaller in the GP than in the MP condition (t33 = –3.201, p = 0.005, d = 0.518). Conversely, the GP condition showed greater beta power at the O2 electrode compared with the MP condition (t33 = 2.514, p = 0.017, d = 0.425).
Fig. 2
Schematic illustration of differences in the relative power of scalp electrodes for the theta (4–7 Hz), alpha (8–12 Hz), and beta (13–35 Hz) bands between gait-prioritization (GP) and manual-prioritization (MP) during dual-task walking. The right subplots of the figure contrast the mean relative powers at various spectral bands in the regions of interest between the GP and MP conditions. Light blue indicates less regional activity in the GP than in the MP condition (p < 0.05), and light red indicates greater regional activity in the GP than in the MP condition. The left plots contrast the mean relative power for the electrodes in the regions of interests (circled in blue) between the two focus conditions.
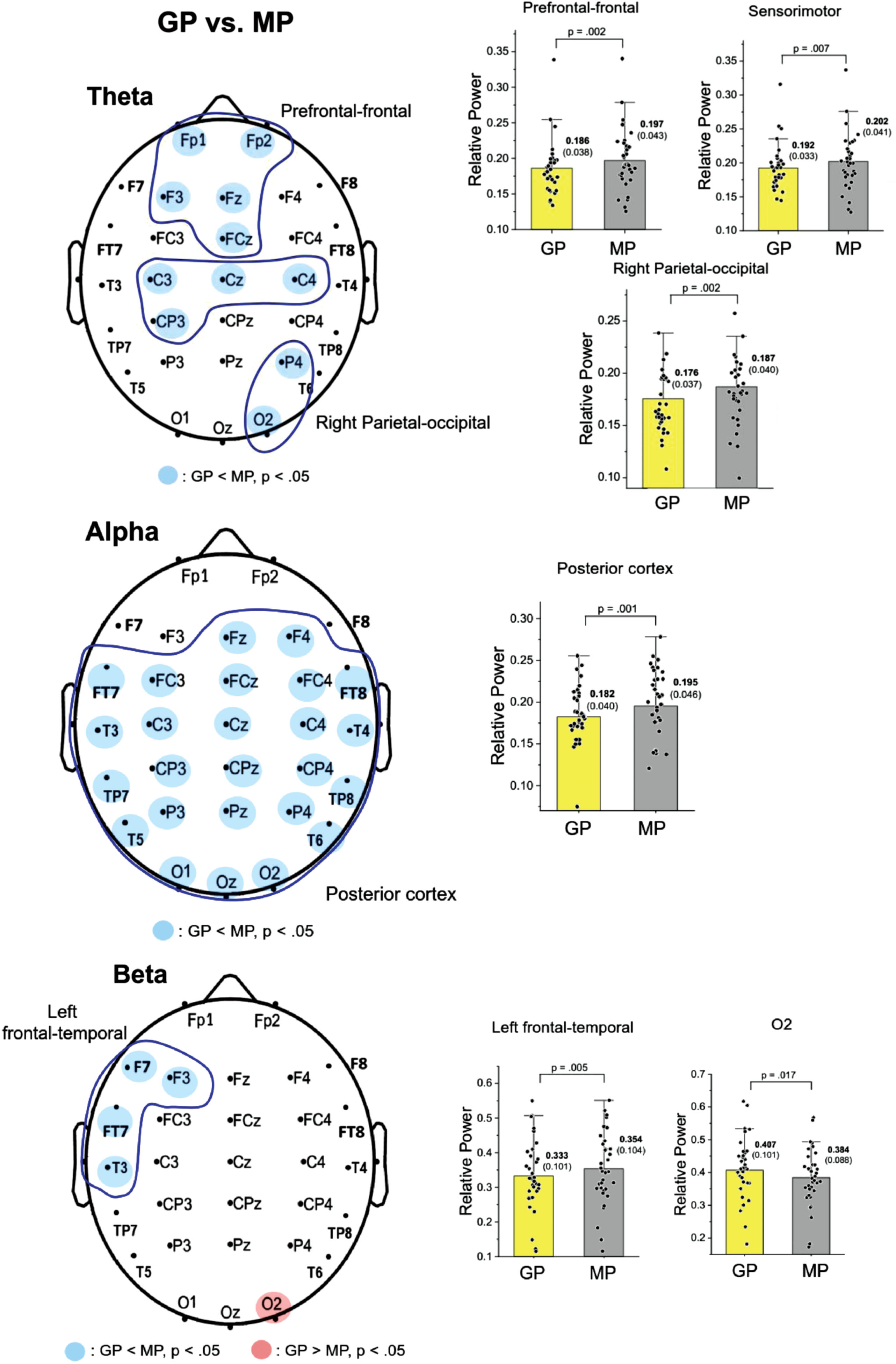
Figure 3 shows the contrast of pooled wPLI of EEG-EEG electrode pairs in the theta, alpha, and beta waves between the GP and MP conditions during dual-task walking. Significant connectivity differences between the GP and MP conditions were identified for the scalp map based on t-values (|t33| > 2.733, p < 0.005) (Fig. 3, right plots). The supra-threshold connectivity highlights the most pronounced changes in functional connectivity resulting from prioritization strategies. Corrected network-based statistics revealed that prioritization strategy significantly influenced the EEG-EEG network in the beta (p = 0.047, corrected), but not in the theta (p = 0.815, corrected) and alpha (p = 0.999, corrected) bands during dual-task walking. The mean wPLI of all supra-threshold beta connectivity was significantly greater in the GP condition (0.499±0.033) compared with the MP condition (0.456±0.035; t33 = 6.622, p < 0.001, d = 1.136). Of note, the T6 electrode exhibited stronger beta connectivity with the mid fronto-centro-parietal area (Fz, FCz, Cz, CPz, and Pz) in the GP condition.
Fig. 3
The adjacent matrices of t values contrasting the weighted phase-lag index (wPLI) values of all electrode pairs in the theta (4–7 Hz), alpha (8–12 Hz), and beta (13–35 Hz) bands between gait-prioritization (GP) and manual-prioritization (MP) during dual-task walking. A contrasting wiring diagram on the scalp shows the topological distributions of the supra-threshold connectivity tuned to the focus paradigm (GP vs. MP) (|t33| > 2.733, p < 0.005). The results of permutation tests for network-based supra-threshold connectivity are showed at the bottom of each contrasting wiring diagram (red line: GP >MP, p < 0.005; blue line: GP <MP, p < 0.005).
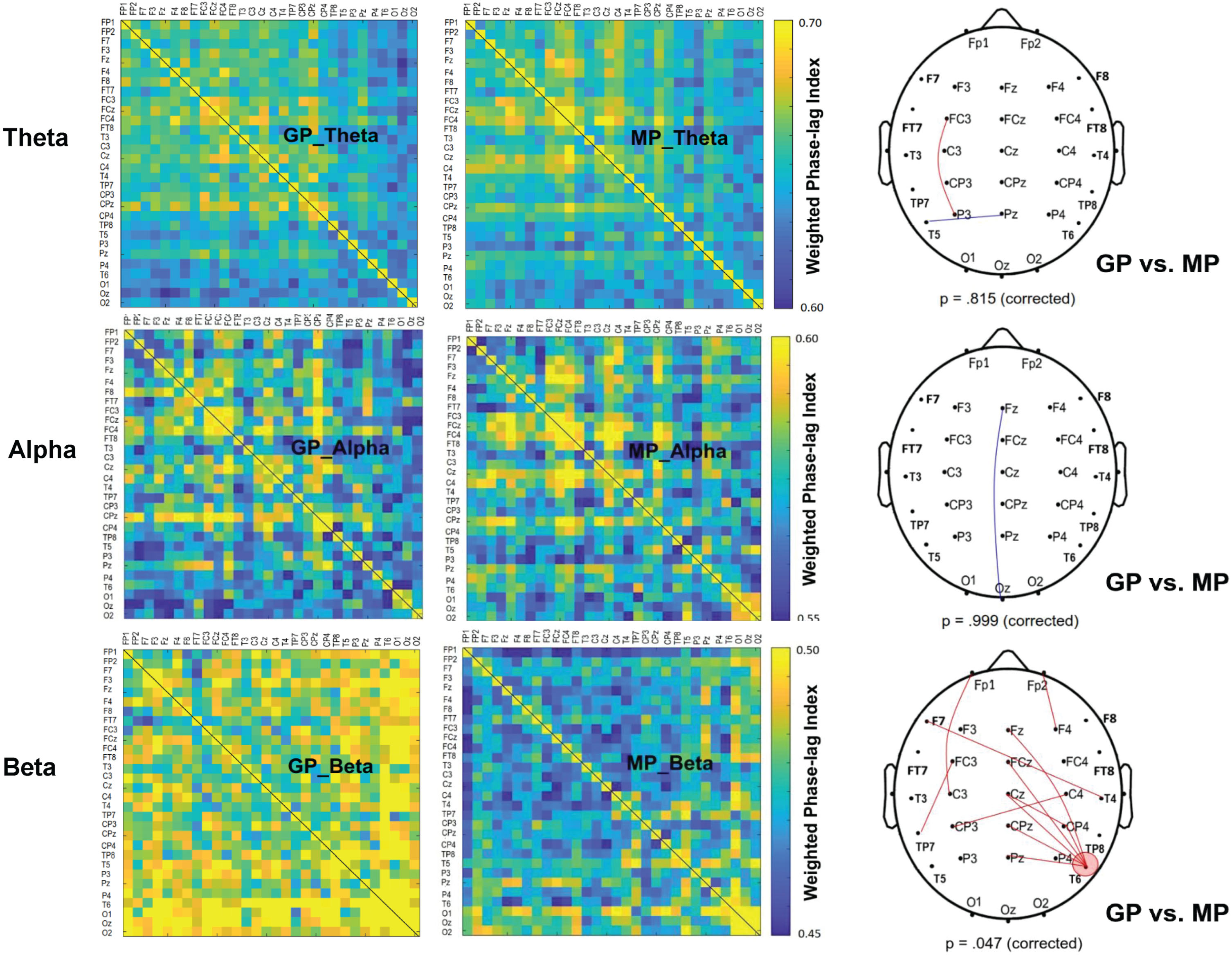
In Fig. 4, the Fig. 4a illustrates a scatter plot between the normalized differences in relative power of the alpha band in the posterior cortex (ND_Post-Alpha) and the normalized differences in gait speed (ND_Speed) resulting from prioritization change. ND_Speed showed a negative correlation with ND_Post-Alpha (r = –0.357, p = 0.038). Similarly, the Fig. 4b demonstrates a significant negative correlation between ND_Post-Alpha and the normalized difference in step length (ND_Step Length) resulting from prioritization change (r = –0.383, p = 0.025). However, there were no significant correlations between the normalized differences in relative power of the theta and beta bands in the regions of interest and the normalized differences in the gait variables in this study (r = –0.208 to 0.247, p > 0.05). Furthermore, the normalized differences in mean w-PLI of all supra-threshold connectivity in the beta band (ND_m-wPLI-Beta) did not correlate with any of the gait variables (r = –0.079 to 0.196, p > 0.05). Figure 4c shows a scatter plot between the normalized difference in relative beta power of the left frontal-temporal area (ND_LFT-Beta) and the normalized difference in ring-touch time (ND_Ring-touch Time) resulting from prioritization change. A significant negative correlation was observed between ND_Ring-touch Time and ND_LFT-Beta (r = –0.346, p = 0.045). Additionally, Fig. 4d shows the scatter plot for ND_m-wPLI-Beta and ND_Ring-touch Time resulting from a prioritization change. The normalized differences in mean w-PLI of all supra-threshold beta connectivity correlated significantly with ring-touch time (r = 0.863, p < 0.001), but no other EEG variables showed a significant correlation with ND_Ring-touch Time (r = –0.007 to 0.247, p > 0.05).
Fig. 4
Pearson correlation between normalized differences (NDs) in EEG variables and dual-task performance for gait-prioritization (GP) and manual-prioritization (MP) during dual-task walking (Post_Alpha: relative alpha power of the posterior cortex; LFT-Beta: relative beta power of the left-frontal-temporal area; m-wPLI-Beta: mean weighted phase-lag index of beta band with significant focus-related difference).
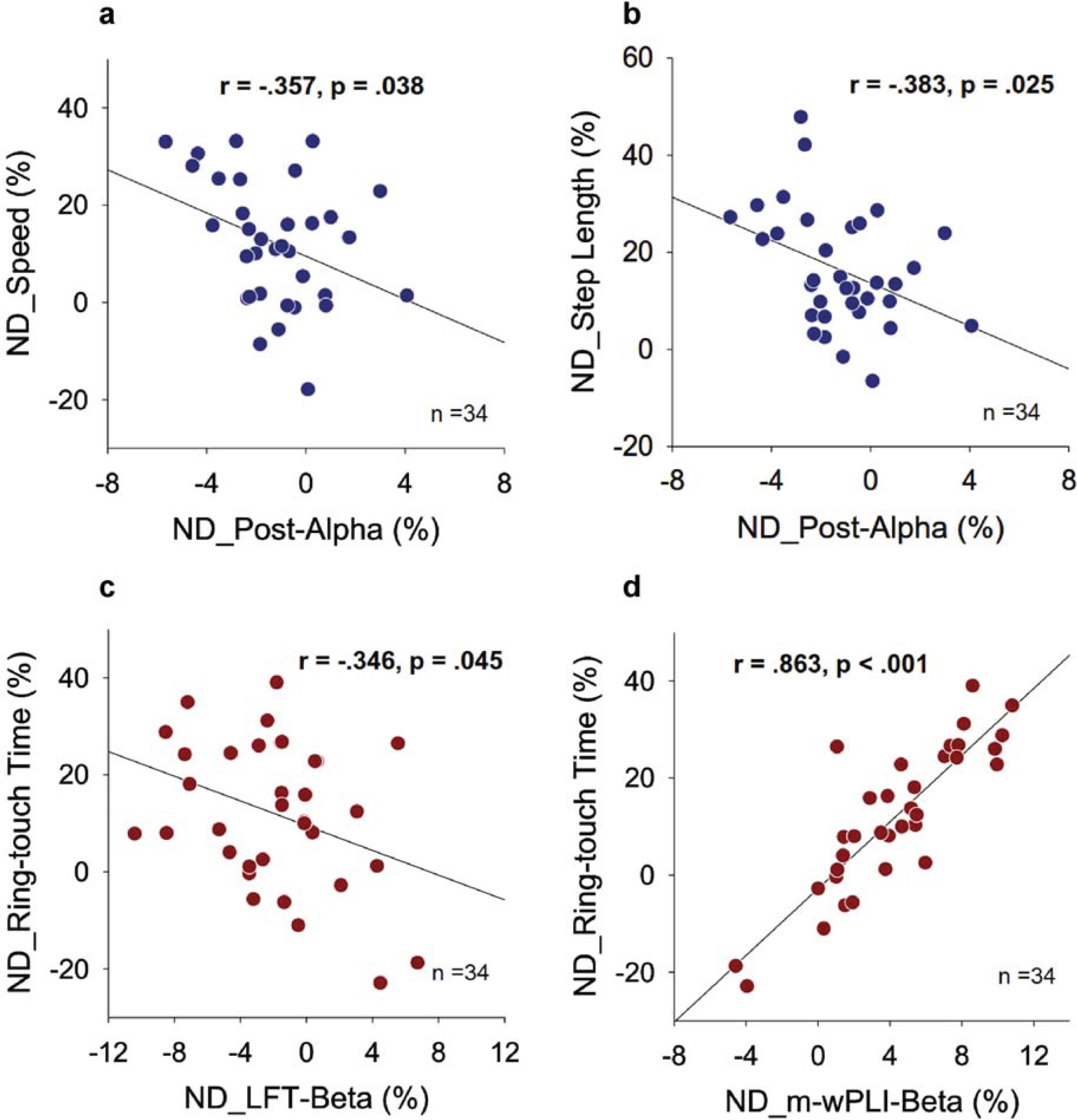
DISCUSSION
The present study demonstrates that individuals with PD could achieve faster walking speed and larger step length while maintaining secondary task performance by employing a GP strategy during dual-task walking. These performance benefits were accompanied by specific modulations in scalp EEG, including regional activity changes in the theta, alpha, and beta bands, as well as inter-regional connectivity changes in the beta band.
Task prioritization effect on theta power
During dual-task walking in this study, the relative theta power differed between prioritization strategies, with lower power observed in the prefrontal-frontal-center area in the GP compared with the MP condition (Fig. 2). Prefrontal/frontal theta oscillation reflects the demands of concurrent task scheduling and sustained attention [49, 50], and prefrontal/frontal theta power amplitude can be used as an indicator of cognitive load during dual-task interference. For example, compared with walking alone, walking while engaging in conversation leads to increased theta power in the frontal region [51]. In addition, increased relative theta power in the frontal or fronto-parietal area has been observed in high-demand concurrent tasks during dual-task walking or when processing high conflict information [34, 50]. Accordingly, the observed decrease in frontal theta power with the GP strategy suggests a lower cognitive load for people with PD [49]. Thus, adopting a GP strategy might effectively enhance gait performance in terms of increased walking speed and longer step length (Table 2) by promoting superior neuralefficiency.
Our findings of decreased theta power in the prefrontal-frontal and sensorimotor areas during dual-task walking with a concurrent manual task are consistent with those of Zhang et al. [29]. Using functional near infrared spectroscopy, their group also observed reduced activity in the frontal and parietal areas of individuals with PD prioritizing gait over cognition during dual-task walking. The frontocentral regions, particularly the supplementary motor area, is the primary area for regulating cognitive-motor interactions and supporting action plans for complex movements [52]. The observed decrease in sensorimotor theta power during dual-task walking with the GP strategy theoretically aligns with the extended Bland’s theta sensorimotor integration model [53]. This concordance suggests that the GP strategy reduces the cognitive effort required for movement planning and execution, possibly because of a high level of sensorimotor integration [54].
Task prioritization modulation and walking association in alpha oscillation
During dual-task walking with the GP strategy, individuals with PD showed a widespread decrease in alpha power across the frontal, sensorimotor, temporal, and occipital areas (Fig. 2). This reduction in regional alpha activity is likely associated with functional benefits for locomotion. A study investigating the effects of external auditory guidance on gait in people with PD found that during guided walking with a metronome, alpha oscillations in the parieto-occipital area were lower compared with uncued walking. This decrease in alpha activity was associated with subjective gait improvement [31]. Whittier et al. also found that increasing stride rate during running in individuals with a history of tibial injury led to suppression of alpha power in the parietal cortex [55]. In another study, training healthy young adults to decrease central alpha power using EEG neurofeedback resulted in improved performance on both the single walking task and cognitive dual-task walking [56]. In keeping with the cortical idling hypothesis [57] and gating by inhibition hypothesis [58, 59], the decreased alpha synchronization observed in the GP condition over the posterior cortical area of individuals with PD suggests an increase in conscious control and a reduction in task-switching processes during dual-task walking [60, 61]. When individuals adopt an optimal strategy, they may effectively manage the demands of both walking and manual tasks by reducing interference, inhibiting unnecessary or noisy processes, and facilitating well-integrated information processing [62]. The significant negative correlation between gait performance and alpha power in the posterior cortex (Fig. 4a, b) further supports the interpretation that alpha suppression with the GP strategy reflects enhanced attentive control over locomotion. This enhanced control leads to improve gait performance, including faster gait speed and longer step length, during dual-task walking in individuals with PD.
Task prioritization modulation and ring-task association in beta oscillation
In the GP condition, beta band activity in the left fronto-temporal area was less than in the MP condition (Fig. 2), which is in keeping with the left hemisphere’s involvement in skilled movement [63]. Deficits in force coordination of the bimanual force-matching task have been observed when the fronto-temporal cortex is excised, indicating its role in monitoring proprioceptive feedback and programming the appropriate amount of force output [64]. Individuals with early-stage PD often exhibit greater cortical atrophy of the left hemisphere, particularly in the left frontal-temporal region [63, 65]. Greater EEG beta power in the left fronto-temporal area reflects sustained attention required for response accuracy [66]. Beta oscillation in the fronto-temporal area also is associated with conflict resolution for goal-directed voluntary actions in people with PD and in unaffected adults [67]. In a hand-controlled joystick task, trials with larger tracking errors were associated with smaller beta power [68]. Of interest, with the GP strategy in the current study, participants who had smaller beta power exhibited a longer ring-touch time (Fig. 4c). A decrease in beta power at the specific site in the GP condition could represent an adaptive action selection due to gait prioritization, as it led the brain to compromise and dedicate less attentional resources to executing a concurrent manual task.
The GP strategy, however, also resulted in enhancing beta connectivity strength between the midline electrodes of Fz, FCz, Cz, CPz, and Pz to T6 (Fig. 3). This increase in circuit-specific functional connectivity due to gait prioritization may have led to an increase in ring-touch time (or poorer performance) for the manual task (Fig. 4d). To our knowledge, no previous study has reported a similar finding for dual-task situations. Our observation of greater beta oscillations suggests a potential role in maintaining the current sensorimotor set during dual-task walking with GP [69]. The enhanced beta connectivity observed in the GP condition may indicate top-down processing aimed at maintaining the coordination of big steps with the current brain state. The shift of attention away from monitoring manual performance in favor of prioritizing gait led to longer ring-touch time. The involvement of cortical midline structures in this process is believed to be related to error detection, particularly in situations with high response-conflict [70, 71]. On the other hand, the right temporo-parietal junction (T6) is responsible for spatial selective attention and self-body location in space, according to the body schema theory of the brain [72–74]. The positive relationship between ring-touch time and beta connectivity suggests that individuals with PD who devote more cognitive process to controlling locomotion in the GP condition may exhibit poorer manual performance, potentially because of reduced attention allocated to the manual task. In such a scenario, attentional resources would be directed more towards gait, leading to a trade-off in manual performance.
Overall, the decline in left regional beta activity and enhancement of the right inter-regional beta connectivity may help prevent excessive cortical resource recruitment during dual-task walking with GP. Fortunately, in behavior contexts, GP did not lead to a significant increase in ring-touch time (Table 2), despite a potential negative impact on the manual task.
Methodological concerns and study limitations
First, we analyzed power and weighted phase-lag index (wPLI) to represent average cortical activation and connectivity within a gait cycle during dual-task walking. However, it was not possible to uncover phase-dependent variations in EEG-EEG connectivity over a short time within a gait cycle. The sliding window method could be a potential solution; however, there is no consensus on the window length in the literature [75, 76], especially given its limitation in achieving high temporal resolution metrics of functional connectivity within a short gait cycle. Although this study simply assessed average connectivity and regional power within a gait cycle, we successfully demonstrated the prioritization effect on EEG dynamics for people with PD during dual-task walking. Future studies could explore variations in the prioritization effect on EEG dynamics within a gait cycle during dual-task walking. Next, the prioritization effect in PD might involve subcortical structures such as the striatum and hippocampus [77]. The activities of subcortical structures cannot be adequately characterized with scalp EEG. Third, this study lacked an age-matched control group, limiting the comparison of task prioritization effects on dual-task walking between individuals with and without PD. However, examining the prioritization of gait in healthy adults is of limited clinical significance, as they can well accomplish dual-task walking without obvious functional constraints and safety concerns. Clinically, only people with PD are necessarily benefited from a gait prioritization strategy, as reported in this study. Finally, considering different brain regions involved and the variability on dual-task paradigms, it would be prudent to extend our conclusions on prioritization effects to dual-task walking with concurrent cognitive tasks or in PD with different executive dysfunction or gait disorder (e.g., freezers vs. non-freezers) [78, 79]. The use of a dual-task paradigm involving a concurrent motor task during walking in this study is to emphasize set-shifting for the coordination of physical movements, which is believed to introduce greater task interference in limited motor resources for people with PD [23]. Further research is needed to explore the task-dependent prioritization effects on people with PD.
CONCLUSION
In dual-task walking for people with PD, a posture-first strategy focusing on walking improved gait speed and step length without compromising manual performance. Improvements in walking speed (or step length) were correlated with prioritization-related changes in alpha power in the posterior cortex. Concurrent manual performance, on the other hand, was linked to regional beta activity in the left fronto-temporal area and inter-regional connectivity in the beta band, extending from the midline to the right temporo-parietal region.
ACKNOWLEDGMENTS
The authors have no acknowledgments to report.
FUNDING
This work was supported by a grant from the National Science and Technology Council, Taiwan (grant no. NSTC 112-2314-B-002-136-MY3).
CONFLICT OF INTEREST
The authors have no conflict of interest to report.
DATA AVAILABILITY
The data sets generated and analyzed in this study are available from the corresponding author upon reasonable request.
REFERENCES
[1] | Yogev-Seligmann G , Hausdorff JM , Giladi N ((2008) ) The role of executive function and attention in gait. Mov Disord 23: , 329–342. |
[2] | Zgaljardic DJ , Borod JC , Foldi NS , Mattis PJ , Gordon MF , Feigin A , Eidelberg D ((2006) ) An examination of executive dysfunction associated with frontostriatal circuitry in Parkinson’s disease. J Clin Exp Neuropsychol 28: , 1127–1144. |
[3] | Raffegeau TE , Krehbiel LM , Kang N , Thijs FJ , Altmann LJP , Cauraugh JH , Hass CJ ((2019) ) A meta-analysis: Parkinson’s disease and dual-task walking. Parkinsonism Relat Disord 62: , 28–35. |
[4] | Belghali M , Chastan N , Cignetti F , Davenne D , Decker LM ((2017) ) Loss of gait control assessed by cognitive-motor dual-tasks: Pros and cons in detecting people at risk of developing Alzheimer’s and Parkinson’s diseases. Geroscience 39: , 305–329. |
[5] | Kelly VE , Eusterbrock AJ , Shumway-Cook A ((2012) ) A review of dual-task walking deficits in people with Parkinson’s disease: Motor and cognitive contributions, mechanisms, and clinical implications. Parkinsons Dis 2012: , 918719. |
[6] | Woollacott M , Shumway-Cook A ((2002) ) Attention and the control of posture and gait: A review of an emerging area of research. Gait Posture 16: , 1–14. |
[7] | Deviterne D , Gauchard GC , Jamet M , Vançon G , Perrin PP ((2005) ) Added cognitive load through rotary auditory stimulation can improve the quality of postural control in the elderly. Brain Res Bull 64: , 487–492. |
[8] | Prado JM , Stoffregen TA , Duarte M ((2007) ) Postural sway during dual tasks in young and elderly adults. Gerontology 53: , 274–281. |
[9] | Jehu DA , Desponts A , Paquet N , Lajoie Y ((2015) ) Prioritizing attention on a reaction time task improves postural control and reaction time. Int J Neurosci 125: , 100–106. |
[10] | Yu SH , Huang CY ((2017) ) Improving posture-motor dual-task with a supraposture-focus strategy in young and elderly adults. PLoS One 12: , e0170687. |
[11] | Huang CY , Chen YA , Hwang IS , Wu RM ((2018) ) Improving dual-task control with a posture-second strategy in early-stage Parkinson disease. Arch Phys Med Rehabil 99: , 1540–1546.e2. |
[12] | Huang CY , Chen LC , Wu RM , Hwang IS ((2022) ) Effects of task prioritization on a postural-motor task in early-stage Parkinson’s disease: EEG connectivity and clinical implication. Geroscience 44: , 2061–2075. |
[13] | Hung YT , Chen LC , Wu RM , Huang CY ((2020) ) The Effects of task prioritization on dual-tasking postural control in patients with Parkinson disease who have different postural impairments. Arch Phys Med Rehabil 101: , 1212–1219. |
[14] | Oh-Park M , Holtzer R , Mahoney J , Wang C , Raghavan P , Verghese J ((2013) ) Motor dual-task effect on gait and task of upper limbs in older adults under specific task prioritization: Pilot study. Aging Clin Exp Res 25: , 99–106. |
[15] | Yogev-Seligmann G , Rotem-Galili Y , Mirelman A , Dickstein R , Giladi N , Hausdorff JM ((2010) ) How does explicit prioritization alter walking during dual-task performance? Effects of age and sex on gait speed and variability. Phys Ther 90: , 177–186. |
[16] | Bloem BR , Grimbergen YA , van Dijk JG , Munneke M ((2006) ) The “posture second” strategy: A review of wrong priorities in Parkinson’s disease. J Neurol Sci 248: , 196–204. |
[17] | Yogev-Seligmann G , Rotem-Galili Y , Dickstein R , Giladi N , Hausdorff JM ((2012) ) Effects of explicit prioritization on dual task walking in patients with Parkinson’s disease. Gait Posture 35: , 641–646. |
[18] | Canning CG ((2005) ) The effect of directing attention during walking under dual-task conditions in Parkinson’s disease. Parkinsonism Relat Disord 11: , 95–99. |
[19] | Baker K , Rochester L , Nieuwboer A ((2007) ) The immediate effect of attentional, auditory, and a combined cue strategy on gait during single and dual tasks in Parkinson’s disease. Arch Phys Med Rehabil 88: , 1593–1600. |
[20] | Huang CY , Chen YA , Wu RM , Hwang IS ((2022) ) Dual-task walking improvement with enhanced kinesthetic awareness in Parkinson’s disease with mild gait impairment: EEG connectivity and clinical implication. Front Aging Neurosci 14: , 1041378. |
[21] | Kelly VE , Eusterbrock AJ , Shumway-Cook A ((2012) ) The effects of instructions on dual-task walking and cognitive task performance in people with Parkinson’s disease. Parkinsons Dis 2012: , 671261. |
[22] | Lin YP , Lin II , Chiou WD , Chang HC , Chen RS , Lu CS , Chang YJ ((2023) ) The executive-function-related cognitive-motor dual task walking performance and task prioritizing effect on people with Parkinson’s disease. Healthcare (Basel) 11: , 567. |
[23] | Wickens CD ((2008) ) Multiple resources and mental workload. Hum Factors 50: , 449–455. |
[24] | Wrightson JG , Twomey R , Ross EZ , Smeeton NJ ((2015) ) The effect of transcranial direct current stimulation on task processing and prioritisation during dual-task gait. Exp Brain Res 233: , 1575–1583. |
[25] | Mori T , Takeuchi N , Izumi SI ((2018) ) Prefrontal cortex activation during a dual task in patients with stroke. Gait Posture 59: , 193–198. |
[26] | Collett J , Fleming MK , Meester D , Al-Yahya E , Wade DT , Dennis A , Salvan P , Meaney A , Cockburn J , Dawes J , Johansen-Berg H , Dawes H ((2021) ) Dual-task walking and automaticity after Stroke: Insights from a secondary analysis and imaging sub-study of a randomised controlled trial. Clin Rehabil 35: , 1599–1610. |
[27] | Johannsen L , Li KZ , Chechlacz M , Bibi A , Kourtzi Z , Wing AM ((2013) ) Functional neuroimaging of the interferencebetween working memory and the control of periodic ankle movement timing. Neuropsychologia 51: , 2142–2153. |
[28] | Holtzer R , Verghese J , Allali G , Izzetoglu M , Wang C , Mahoney JR ((2016) ) Neurological gait abnormalities moderate the functional brain signature of the posture first hypothesis. Brain Topogr 29: , 334–343. |
[29] | Zhang X , Wang Y , Lu J , Wang J , Shu Z , Cheng Y , Zhu Z , Liu P , Yu Y , Yu N , Han J , Wu J ((2022) ) Fronto-parietal cortex activation during walking in patients with Parkinson’s disease adopting different postural strategies. Front Neurol 13: , 998243. |
[30] | Calabrò RS , Naro A , Filoni S , Pullia M , Billeri L , Tomasello P , Portaro S , Di Lorenzo G , Tomaino C , Bramanti P ((2019) ) Walking to your right music: A randomized controlled trial on the novel use of treadmill plus music in Parkinson’s disease. J Neuroeng Rehabil 16: , 68. |
[31] | Tosserams A , Weerdesteyn V , Bal T , Bloem BR , Solis-Escalante T , Nonnekes J ((2022) ) Cortical correlates of gait compensation strategies in Parkinson disease. Ann Neurol 91: , 329–341. |
[32] | Foxe JJ , Snyder AC ((2011) ) The role of alpha-band brain oscillations as a sensory suppression mechanism during selective attention. Front Psychol 2,: , 154. |
[33] | Possti D , Fahoum F , Sosnik R , Giladi N , Hausdorff JM , Mirelman A , Maidan I ((2021) ) Changes in the EEG spectral power during dual-task walking with aging and Parkinson’s disease: Initial findings using event-related spectral perturbation analysis. J Neurol 268: , 161–168. |
[34] | Pruziner AL , Shaw EP , Rietschel JC , Hendershot BD , Miller MW , Wolf EJ , Hatfield BD , Dearth CL , Gentili RJ ((2019) ) Biomechanical and neurocognitive performance outcomes of walking with transtibial limb loss while challenged by a concurrent task. Exp Brain Res 237: , 477–491. |
[35] | Hülsdünker T , Mierau A , Neeb C , Kleinöder H , Strüder HK ((2015) ) Cortical processes associated with continuous balance control as revealed by EEG spectral power. Neurosci Lett 592: , 1–5. |
[36] | Başar E ((2012) ) A review of alpha activity in integrative brain function: Fundamental physiology, sensory coding, cognition and pathology. Int J Psychophysiol 86: , 1–24. |
[37] | Beurskens R , Steinberg F , Antoniewicz F , Wolff W , Granacher U ((2016) ) Neural correlates of dual-task walking: Effects of cognitive versus motor interference in young adults. Neural Plast 2016: , 8032180. |
[38] | Jenkinson N , Brown P ((2011) ) New insights into the relationship between dopamine, beta oscillations and motor function. Trends Neurosci 34,: , 611–618. |
[39] | Hughes AJ , Daniel SE , Kilford L , Lees AJ ((1992) ) Accuracy of clinical diagnosis of idiopathic Parkinson’s disease: A clinico-pathological study of 100 cases. J Neurol Neurosurg Psychiatry 55: , 181–184. |
[40] | Goetz CG , Tilley BC , Shaftman SR , Stebbins GT , Fahn S , Martinez-Martin P , Poewe W , Sampaio C , Stern MB , Dodel R , Dubois B , Holloway R , Jankovic J , Kulisevsky J , Lang AE , Lees A , Leurgans S , LeWitt PA , Nyenhuis D , Olanow CW , Rascol O , Schrag A , Teresi JA , van Hilten JJ , LaPelle N ((2008) ) Movement Disorder Society-sponsored revision of the Unified Parkinson’s Disease Rating Scale (MDS-UPDRS): Scale presentation and clinimetric testing results. Mov Disord 23: , 2129–2170. |
[41] | Alarcon G , Guy CN , Binnie CD ((2020) ) A simple algorithm for a digital three-pole Butterworth filter of arbitrary cut-off frequency: Application to digital electroencephalography. J Neurosci Methods 104: , 35–44. |
[42] | Whitham EM , Pope KJ , Fitzgibbon SP , Lewis T , Clark CR , Loveless S , Broberg M , Wallace A , DeLosAngeles D , Lillie P , Hardy A , Fronsko R , Pulbrook A , Willoughby JO ((2007) ) Scalp electrical recording during paralysis: Quantitative evidence that EEG frequencies above 20 Hz are contaminated by EMG. Clin Neurophysiol 118: , 1877–1888. |
[43] | Semlitsch HV , Anderer P , Schuster P , Presslich O ((1986) ) A solution for reliable and valid reduction of ocularartifacts, applied to the P300 ERP. Psychophysiology 23: , 695–703. |
[44] | Croft RJ , Barry RJ ((2000) ) Removal of ocular artifact from the EEG: A review. Neurophysiol Clin 30: , 5–19. |
[45] | Vinck M , Oostenveld R , van Wingerden M , Battaglia F , Pennartz CM ((2011) ) An improved index of phase-synchronization for electrophysiological data in the presence of volume-conduction, noise and sample-size bias. Neuroimage 55: , 1548–1565. |
[46] | Lau TM , Gwin JT , McDowell KG , Ferris DP ((2012) ) Weighted phase lag index stability as an artifact resistant measure to detect cognitive EEG activity during locomotion. J Neuroeng Rehabil 9: , 47. |
[47] | Niso G , Bruña R , Pereda E , Gutiérrez R , Bajo R , Maestú F , del-Pozo F ((2013) ) HERMES: Towards an integrated toolbox to characterize functional and effective brain connectivity. Neuroinformatics 11: , 405–434. |
[48] | Zalesky A , Fornito A , Bullmore ET ((2010) ) Network-based statistic: Identifying differences in brain networks. Neuroimage 53: , 1197–1207. |
[49] | Eschmann KCJ , Bader R , Mecklinger A ((2018) ) Topographical differences of frontal-midline theta activity reflect functional differences in cognitive control abilities. Brain Cogn 123: , 57–64. |
[50] | Duprez J , Gulbinaite R , Cohen MX ((2020) ) Midfrontal theta phase coordinates behaviorally relevant brain computations during cognitive control. Neuroimage 207: , 116340. |
[51] | Pizzamiglio S , Naeem U , Abdalla H , Turner DL ((2017) ) Neural correlates of single- and dual-task walking in the real world. Front Hum Neurosci 11: , 460. |
[52] | Leisman G , Moustafa AA , Shafir T ((2016) ) Thinking, walking, talking: Integratory motor and cognitive brain function. Front Public Health 4,: , 94. |
[53] | Bland BH , Oddie SD ((2001) ) Theta band oscillation and synchrony in the hippocampal formation and associated structures: The case for its role in sensorimotor integration. Behav Brain Res 127: , 119–136. |
[54] | Cruikshank LC , Singhal A , Hueppelsheuser M , Caplan JB ((2012) ) Theta oscillations reflect a putative neural mechanism for human sensorimotor integration. J Neurophysiol 107: , 65–77. |
[55] | Whittier T , Willy RW , Sandri Heidner G , Niland S , Melton C , Mizelle JC , Murray NP ((2020) ) The Cognitive Demands of Gait Retraining in Runners: An EEG Study. J Mot Behav 52: , 360–371. |
[56] | Sidhu A , Cooke A ((2021) ) Electroencephalographic neurofeedback training can decrease conscious motor control and increase single and dual-task psychomotor performance. Exp Brain Res 239: , 301–313. |
[57] | Pfurtscheller G , Stancák A Jr , Neuper C ((1996) ) Event-related synchronization (ERS) in the alpha band–an electrophysiological correlate of cortical idling: A review. Int J Psychophysiol 24: , 39–46. |
[58] | Freunberger R , Werkle-Bergner M , Griesmayr B , Lindenberger U , Klimesch W ((2011) ) Brain oscillatory correlates of working memory constraints. Brain Res 1375: , 93–102. |
[59] | Roberts BM , Hsieh LT , Ranganath C ((2013) ) . Oscillatory activity during maintenance of spatial and temporal information in working memory. Neuropsychologia 51: , 349–357. |
[60] | Klimesch W , Doppelmayr M , Röhm D , Pöllhuber D , Stadler W ((2000) ) Simultaneous desynchronization and synchronization of different alpha responses in the human electroencephalograph: A neglected paradox? Neurosci Lett 284: , 97–100. |
[61] | Mastria S , Agnoli S , Zanon M , Acar S , Runco MA , Corazza GE ((2021) ) Clustering and switching in divergent thinking: Neurophysiological correlates underlying flexibility during idea generation. Neuropsychologia 158: , 107890. |
[62] | Klimesch W ((2012) ) α-band oscillations, attention, and controlled access to stored information. Trends Cogn Sci 16: , 606–617. |
[63] | Claassen DO , McDonell KE , Donahue M , Rawal S , Wylie SA , Neimat JS , Kang H , Hedera P , Zald D , Landman B , Dawant B , Rane S ((2016) ) Cortical asymmetry in Parkinson’s disease: Early susceptibility of the left hemisphere. Brain Behav 6: , e00573. |
[64] | Jones L ((1989) ) Force matching by patients with unilateral focal cerebral lesions. Neuropsychologia. Neuropsychologia 27: , 1153–1163. |
[65] | Mostile G , Giuliano L , Dibilio V , Luca A , Cicero CE , Sofia V , Nicoletti A , Zappia M ((2019) ) Complexity of electrocortical activity as potential biomarker in untreated Parkinson’s disease. J Neural Transm (Vienna) 126: , 167–172. |
[66] | Valentino DA , Arruda JE , Gold SM ((1993) ) Comparison of QEEG and response accuracy in good vs poorer performers during a vigilance task. Int J Psychophysiol 15: , 123–133. |
[67] | Duprez J , Tabbal J , Hassan M , Modolo J , Kabbara A , Mheich A , Drapier S , Vérin M , Sauleau P , Wendling F , Benquet P , Houvenaghel JF ((2022) ) Spatio-temporal dynamics of large-scale electrophysiological networks during cognitive action control in healthy controls and Parkinson’s disease patients. Neuroimage 258: , 119331. |
[68] | Tan H , Jenkinson N , Brown P ((2014) ) Dynamic neural correlates of motor error monitoring and adaptation during trial-to-trial learning. J Neurosci 34: , 5678–5688. |
[69] | Engel AK , Fries P ((2010) ) Beta-band oscillations–signalling the status quo? Curr Opin Neurobiol 20: , 156–165. |
[70] | Fassbender C , Hester R , Murphy K , Foxe JJ , Foxe DM , Garavan H ((2009) ) Prefrontal and midline interactions mediating behavioural control. Eur J Neurosci 29: , 181–187. |
[71] | Dali G , Brosnan M , Tiego J , Johnson BP , Fornito A , Bellgrove MA , Hester R ((2022) ) Examining the neural correlates of error awareness in a large fMRI study. Cereb Cortex 33: , 458–468. |
[72] | Blanke O , Mohr C , Michel CM , Pascual-Leone A , Brugger P , Seeck M , Landis T , Thut G ((2005) ) Linking out-of-body experience and self processing to mental own-body imagery at the temporoparietal junction. J Neurosci 25: , 550–507. |
[73] | Shulman GL , Pope DL , Astafiev SV , McAvoy MP , Snyder AZ , Corbetta M ((2010) ) Right hemisphere dominance during spatial selective attention and target detection occurs outside the dorsal frontoparietal network. J Neurosci 30: , 3640–3651. |
[74] | Graziano MS , Kastner S ((2011) ) Human consciousness and its relationship to social neuroscience: A novel hypothesis. Cogn Neurosci 2: , 98–113. |
[75] | O’Neill GC , Tewarie P , Vidaurre D , Liuzzi L , Woolrich MW , Brookes MJ ((2018) ) Dynamics of large-scale electrophysiological networks: A technical review. Neuroimage 180: , 559–576. |
[76] | Long Z , Liu X , Niu Y , Shang H , Lu H , Zhang J , Yao L ((2023) ) Improved dynamic functional connectivity estimation with an alternating hidden Markov model. Cogn Neurodyn 17: , 1381–1398. |
[77] | Kim H , Fraser S ((2022) ) Neural correlates of dual-task walking in people with central neurological disorders: A systematic review. J Neurol 269: , 2378–2402. |
[78] | Giladi N , Huber-Mahlin V , Herman T , Hausdorff JM ((2007) ) Freezing of gait in older adults with high level gait disorders: Association with impaired executive function. J Neural Transm (Vienna) 114: , 1349–1353. |
[79] | Nutt JG , Bloem BR , Giladi N , Hallett M , Horak FB , Nieuwboer A ((2011) ) Freezing of gait: Moving forward on a mysterious clinical phenomenon. Lancet Neurol 10: , 734–744. |