Age-Related Adaptive Immune Changes in Parkinson’s Disease
Abstract
Ageing is a major risk factor for most neurodegenerative diseases, including Parkinson’s disease (PD). Progressive age-related dysregulation of the immune system is termed immunosenescence and is responsible for the weakened response to novel antigens, increased susceptibility to infections and reduced effectiveness of vaccines seen in the elderly. Immune activation, both within the brain and periphery, is heavily implicated in PD but the role of immunosenescence has not been fully explored. Studies to date provide some evidence for an attenuation in immunosenescence in PD, particularly a reduction in senescent CD8 T lymphocytes in PD cases compared to similarly aged controls. Here, we discuss recent evidence of age-related immune abnormalities in PD with a focus on T cell senescence and explore their potential role in disease pathogenesis and development.
INTRODUCTION
Ageing is characterized by a gradual deterioration of biological functions and is the main risk factor for multiple pathological conditions, such as cardiovascular disease [1], cancer [2], and neurodegenerative disorders [3]. Immunosenescence is an established consequence of ageing and is characterized by a progressive decline in the function of the immune system resulting in a weakened response to novel antigens. Immunosenescence contributes to increased susceptibility to infections, reduced effectiveness of vaccines [4], as well as increased prevalence of autoimmunity and inflammatory conditions in the elderly [5].
Alterations in the peripheral immune system are well-described in Parkinson’s disease (PD), with changes in innate and adaptive immunophenotypes as well as proinflammatory cytokines being linked to a more aggressive disease phenotype [6–8]. The relevance of ageing to the immune component of PD has not been widely explored, but warrants investigation given that PD incidence rises steadily with age such that it is nearly 50-fold higher in the 80–84 age-group versus the 45–49 age-group [9]. In this review, we discuss the current evidence on age-related immune changes in PD and consider how they may play a role in the clinical course of the disease.
IMMUNE CHANGES ASSOCIATED WITH ADVANCING AGE
The term immunosenescence largely describes the reduction in the repertoire of adaptive immune cell receptors and the increased oligoclonal expansion of memory immune cells which occurs with ageing. In particular, prominent hallmarks of immunosenescence include a decrease in the naïve T cell pool with a concomitant accumulation of memory T lymphocytes, especially terminally differentiated effector memory cells (TEMRA). These changes are seen both in helper CD4 and in cytotoxic CD8 T cells, though the shift in the CD8 population is much greater [10]. Senescent T cells are antigen-experienced, providing the host with “antigenic memory” [11], and their age-related increase is primarily due to chronic exposure to different pathogens and the gradual involution of the thymus [10]. TEMRA cells are typically characterised by loss of C–C chemokine receptor type 7 (CCR7) and expression of CD45RA on their cell surface [12]. Senescent CD8 lymphocytes can also be identified by their decreased expression of the activation marker CD28 and upregulation of CD57, a marker of terminal differentiation [13–15]. These senescent cells are highly oligoclonal, have a diminished capacity to proliferate, and are proinflammatory [16, 17]. There is a strong association between the number of senescent T cells and infection with persistent viruses, such as cytomegalovirus (CMV). Chronic stimulation of T cells with CMV in vitro triggers clonal expansion followed by further differentiation; eventually these antigen-experienced T cells lose their ability to proliferate, display an altered phenotype and reach a stage of replicative senescence [18]. Both CD8 TEMRA and CD28loCD57hi T cell populations are increased in elderly CMV seropositive populations [19, 20]. Thus, CMV infection has been proposed as a major driver of the accumulation of senescent CD8 T lymphocytes [21, 22].
Progressive atrophy of the thymus, a lymphoid organ vital for the maturation of T lymphocytes, is another prominent characteristic of the ageing immune system [23]. Thymic involution has been shown to start very early on in life and continue exponentially over time [24, 25]. This results in a reduction in the naïve T cell pool that is able to respond and clonally expand when encountering novel antigens [26]. Thymic involution contributes to the observed shift in the immune profile towards a higher proportion of antigen-experienced memory cells with advancing age.
Another well-recognised immune alteration in the elderly is chronic low-grade inflammation in the absence of acute infection, termed “inflammageing”. A large study comprising 873 elderly participants between 70–90 years old with no neurodegenerative disease reported that a moderate increase in systemic inflammation was significantly associated with poorer cognitive function, whilst adjusting for multiple confounding factors [27]. This basal systemic inflammation is characterized by a moderate increase in the levels of pro-inflammatory cytokines (TNFα, IL6, and IFN-γ) and other markers of inflammation such as C-reactive protein (CRP) in the blood of old compared to young individuals [28]. The source of this non-specific inflammation with advancing age is not completely clear.
Overall, age-related immune dysregulation contributes to a reduced ability to respond to new pathogens, alongside a chronic low-grade non-specific inflammation. This may play a critical role in neurodegenerative diseases where ageing is a primary risk factor, and immune activation is well described, such as PD.
AGE-RELATED IMMUNE CHANGES IN PARKINSON’S DISEASE
T lymphocyte senescence
Very few studies to date have specifically investigated markers of T cell senescence in PD. Our own study in 2018 investigated naïve, memory and senescent CD4 and CD8 T cell subsets using flow cytometric analysis in a cohort of 41 PD cases (4.3±1.2 years from diagnosis) and 41 age and sex-matched controls, and found a significant reduction in the proportion of “late-differentiated” CD8 CD28loCD57hi as well as CD8 TEMRA lymphocytes [29], but no significant changes in CD4 cell subsets. In a follow-up study, we investigated similar T cell subsets in a larger newly-diagnosed cohort (0.97±0.5 years from diagnosis) of 61 PD cases and 63 age and sex-matched controls and similarly found a reduction in the number of senescent CD8 TEMRA T cells in patients compared to controls, thus confirming our previous finding [30]. There was also a trend towards reduced CD8 CD28loCD57hi cells in PD cases in this second study, which did not reach significance, but CD8 TEMRA and CD8 CD28loCD57hi cell counts were strongly correlated, and it is likely that these represent overlapping populations [12]. In CD8 T lymphocytes, we observed a significant decrease in the mRNA levels of cyclin-dependent kinase inhibitor p16INK4a, a well-established marker of cellular senescence, in PD cases compared to controls [31]. This provided further evidence that the CD8 population is less senescent in PD. Another study by Vavilova and colleagues has similarly reported a reduction in T cell senescence in a cohort of 31 PD cases (7±0.8 years from diagnosis) compared to 25 healthy controls matched for age and CMV status. They found a reduction in the proportion of CD3 TEMRA T cells and CD57+ T cells in PD, although they did not explore CD8 versus CD4 senescent subsets [32]. In contrast, Wang et al, using single cell transcriptomics in a small number of blood and cerebrospinal fluid (CSF) samples, reported an enrichment in the percentage of terminal effector CD8 T cells, as well as a reduction in naïve CD8 subsets in the blood of PD patients compared to controls [33]. The authors also found evidence of increased T cell clonal expansion both in the blood and the CSF of patients. This discrepancy between studies might be explained to an extent by the different markers used to define terminally differentiated CD8 T cells. Whilst we, and Vavilova et al., identified TEMRA CD8 subsets on the basis of their expression of CD45RA and loss of CCR7, Wang and colleagues used a different set of effector markers, namely GZMA, GZMB, PRF1 and NKG7. Similarly, naïve CD8 cells in our work were defined by high CCR7 and CD45RA expression, whereas Wang et al. used SELL, CCR7, TCF7, and LEF1 to define the naïve CD8 T cell population. As such, these studies are not directly comparable and indeed might be identifying distinct subsets of CD8 lymphocytes.
CD4 T cells are more resistant to age-related changes than CD8 T cells, but compositional shifts in CD4 subsets have been described in healthy elderly individuals, albeit at a later stage than changes in the CD8 population. These changes include a reduction in the CD4:CD8 ratio, a decline in antigen-inexperienced naïve T cells and an accumulation of antigen-experienced and late differentiated cells [34]. Baba and colleagues reported a significant decrease in the CD4:CD8 T cell ratio in PD patients compared to age-matched controls [35], while Bas et al. showed a reduction in the proportion and absolute number of naïve CD45RA+ CD4 T cells in PD versus controls [36]. Our own previous work suggested a moderate increase in the proportion of central memory CD4 T cells (defined as CCR7hiCD45RAlo cells) in PD compared to age-matched controls [29], though this was not replicated in our second newly diagnosed PD cohort [30] and we found no changes in CD4 TEMRA cells in either study. In contrast, another recent study in two small independent PD cohorts reported a reduction in the absolute numbers of central memory CD4 T cells in PD patients, but again, no differences were found in the effector memory and TEMRA CD4 T cell subsets between patients and controls [37]. Hence, there are no consistent findings in relation to senescent shifts in the CD4 population in PD.
Interestingly, no association has been observed in any of the above studies between markers of T cell senescence and measures of disease progression (such as disease stage and severity of motor/cognitive symptoms), but similar reductions in TEMRA cells have been observed in PD cohorts at varying disease stages including in newly diagnosed cases [29, 30, 32]. This suggests that age-related immune dysregulation might be involved in disease pathogenesis rather than progression [29, 30].
Prior exposure to chronic viral infections such as cytomegalovirus (CMV) has been strongly associated with CD8 T cell senescence in the elderly [11]. However, our previous data in two independent PD patient cohorts suggest that the relationship between CMV infection and CD8 T cell senescence is attenuated in PD compared to controls [29, 30]. Prior CMV infection was associated with an increase in senescent CD8 CD28loCD57hi cells in healthy aged controls but this senescent shift in the T cell population was not observed in CMV positive PD cases. In the study by Vavilova and colleagues, reductions in CD3 TEMRA and CD3 CD57+ cells in PD cases were observed in the context of prior CMV infection (with 100% of PD cases being CMV positive, and comparisons made with CMV positive controls) [32]. This raises the question of whether there are intrinsic differences in the T cell response to chronic infections in PD cases versus controls.
The T cell response might also be affected by changes in dendritic cells which play a critical role in T cell priming. Therefore, investigating age-related changes in dendritic cells is highly relevant in PD. In the healthy population, advancing age is associated with a decrease in the numbers of plasmacytoid dendritic cells, whilst the population of myeloid dendritic cells remains largely unchanged [38]. Both cell types, however, have shown an attenuated secretion of pro-inflammatory cytokines upon Toll-like receptors stimulation in the elderly [39], suggesting immunosenescence of dendritic cells occurs with ageing. In PD, conventional myeloid dendritic cells (cDC2) responsible for priming CD4 T cells [40, 41] as well as, total myeloid dendritic cells have been found to be significantly decreased in PD compared to age-matched controls [42]. Furthermore, a lower proportion of myeloid dendritic cells was associated with worse motor scores (measured by the UPDRS III), suggesting that altered T cell priming might be linked with disease progression [42]. Recent work in a small cohort of PD patients has also shown a reduction in PD-L1+ tolerogenic dendritic cells in patients compared to controls [43]. A reduction in immune tolerance may lead to an increased responsiveness to autoantigens, such as alpha-synuclein. Hence, age-related dysfunction of dendritic cells warrants further research in PD.
There is very limited data on age-related B lymphocyte changes in PD. Frasca and colleagues have demonstrated an increase in the proportion of late memory “exhausted” B cells (CD27–IgD–) in the elderly compared to young controls. These B cells were found to express multiple senescence-associated markers, such as p16INK4 [44]. In PD, a recent study found a decrease in the absolute count of these mature late memory (CD27–IgD–) B cells in patients versus controls, further supporting our findings of reduced immunosenescence in PD [37]. No other studies to date have specifically explored senescent B cell subsets in the context of PD.
Telomere shortening
Telomeres are repetitive sequences of non-coding DNA at the end of chromosomes that protect the chromosome from degradation and intrachromosomal fusion. They shorten with each cell division, and therefore represent a marker of biological ageing of the cell. Thus, telomere length within immune cells may provide an alternative marker of immunosenescence. Indeed replicative CD8 T cell senescence, as defined by the loss of CD28 expression, has been shown to be associated with shortened telomeres [45, 46]. Telomere length measured in total leucocytes has been proposed as a prognostic biomarker in the elderly. In a longitudinal study involving 143 individuals older than 60 years old, Cawthon et al. found a significantly higher mortality rate in people whose leucocytes had the greatest degree of telomere erosion [47]. Leucocyte telomere length has been implicated as a risk factor for many age-associated neurodegenerative conditions including PD, though there is conflicting data across studies. Hudson et al., compared telomere lengths in peripheral blood mononuclear cells of 109 PD cases and 99 controls and reported significantly longer telomeres in PD patients versus controls, consistent with reduced leucocyte senescence [48]. However, a subsequent meta-analysis of 956 PD cases and 1284 controls across 8 studies showed no significant differences between PD and controls. Longitudinal studies in PD cohorts also report contrasting findings. Degerman et al. found no difference in telomere length in PD (n = 136) versus controls (n = 30) at baseline or 3 years follow-up, but reported a significantly higher dementia risk within 3 years of diagnosis in PD patients with longer leucocyte telomere length at baseline [49]. In contrast, in an incident cohort of 154 early-stage PD patients and 99 controls (ICICLE-PD) we found shorter telomeres in the peripheral blood of patients both at baseline and at 18 months compared to controls. PD cases who went on to develop dementia within 3 years had significantly shorter telomeres than those who remained cognitively intact [50]. A limitation of these studies is that they have examined telomere length in the total leucocyte population, whilst it may be more relevant to measure this marker within specific cell subsets which demonstrate replicative senescence with ageing, such as the CD8 population. We recently compared both telomere length as well as the expression of hTERT (telomerase reverse transcriptase; the catalytic subunit of the enzyme telomerase, responsible for the maintenance of telomere ends) specifically in the CD8 T cell population of 61 PD patients compared to 63 age-matched controls but found no difference between the groups [30]. Hence to date, there is no strong evidence to support the use of telomere length as an immunosenescence-related biomarker in PD.
Thymic involution
Atrophy of the thymus is a well described feature of ageing which results in a reduction in the output of naïve T cells and a more restricted ability to respond to novel antigens. [24, 25]. Thymic output has not been extensively explored in PD. An indirect and non-invasive method of quantifying thymic function and T cell output is by measuring the number of naïve CD4 and CD8 recent thymic emigrant T cells using flow cytometry [51, 52]. In our recent study, we quantified the number of PTK7+ CD4 and CD103+ CD8 recent thymic emigrants in the peripheral blood of 30 PD patients and 30 age and sex-matched controls and found no differences between the groups. This suggests that the decrease seen in T cell replicative senescence in PD patients that we have observed in our cohorts is not directly related to preservation of thymic function, but further studies are needed to adequately investigate this.
Inflammageing
Several studies have reported an increase in the levels of peripheral proinflammatory mediators in PD patients. In a meta-analysis of 25 studies involving a total of 1547 PD patients and 1107 controls, Qin et al. reported significantly elevated concentrations of the serum cytokines IL-6, TNFα, IL-1β, CRP, IL-10, and IL-2 and the chemokine RANTES in patients versus controls [53]. Our own study has demonstrated that inflammation is present at the earliest stages of the disease, with a significant but low-grade increase in TNFα, IL-1β, IL-10, and IL-2 in an incident cohort of 262 PD patients compared to 99 age-matched controls. Using a principal component analysis approach, we identified a proinflammatory immune marker profile characterised by high levels of IL-6, IFNγ, TNFα, IL-2, IL-10, and CRP and low levels of IL-4 and IL-13, with prognostic significance. Specifically, this proinflammatory profile at baseline was predictive of a more rapid deterioration in motor function (measured by UPDRS motor scores) over 3 years of follow-up, as well as persistently lower cognitive scores (measured by the Mini-Mental State Examination) [6]. A subsequent study reported a similar increase in cytokine levels (IL-1β, IL-10, IL-4, and IL-2) in patients with mild cognitive impairment (MCI) with a Lewy body profile and in patients with MCI with an Alzheimer’s disease (AD) profile, as compared to controls [54]. This suggests that inflammageing could be a shared feature in both AD and PD. Interestingly, a proteomic study in a small group of patients with REM sleep behaviour disorder (who have a 60–81% likelihood of developing parkinsonism/dementia within 10 years) [55, 56] has reported a significant upregulation of CRP as well as other immune-related molecules in patients versus controls, thus implicating peripheral inflammation in prodromal PD [57].
In the ageing population, an increase in the concentration of proinflammatory molecules including TNFα, IL-8, and IFNγ-induced protein 10 has also been observed in the CSF [58]. Studies examining cytokine levels in the CSF of PD patients have found this inflammatory shift to be heightened in demented, but not in cognitively intact patients. Specifically, increased levels of IL-6 [59], IL-8 [60], and CRP [61] have been observed in the CSF of demented versus non-demented PD patients. Higher levels of CRP and monocyte chemoattractant protein-1 have also been reported to be closely linked to the development of cognitive impairment, depression and fatigue in PD [62]. Similarly, Hall and colleagues showed correlations between worse motor function (as measured by the Hoehn & Yahr and UPDRS motor scores) in PD and elevated CRP and serum amyloid A (an acute phase reactant) in the CSF. The authors also found significant correlations between depression and the same CSF inflammatory markers, as well as an association between lower cognitive scores and higher serum amyloid A in the CSF [61]. Proteomic studies in CSF have also shown an increase of HLA proteins (HLA-DRA, HLA-DRB1 and HLA-DPA1) [63] in familial PD patients (LRRK2 G2019S carriers) versus controls, providing further evidence of increased inflammation in PD.
Taken together, this data suggests that low grade inflammation in both the blood and the CSF plays a detrimental role in PD and may be implicated in the development of dementia. It is, however, unclear whether these inflammatory changes seen in PD are due to age-related dysregulation of the immune system or rather due to disease-related processes. Alpha-synuclein aggregates, which are known to circulate in peripheral blood in PD [64], can induce a peripheral inflammatory response via Toll-like receptor activation [65]. Both alpha-synuclein pathology and microbial changes in the gut have also been implicated in driving a proinflammatory state in PD [66, 67]. Dissecting out the contribution of immune ageing to this inflammatory state in PD represents a significant challenge.
AGE-RELATED IMMUNE CHANGES IN ALZHEIMER’S DISEASE
Ageing is a major risk factor for most neurodegenerative conditions including Alzheimer’s disease, thus immune ageing may have a common relevance in these diseases. The immune system (primarily the innate component) has been heavily implicated in the pathogenesis and progression of AD, but less is known about the role of immunosenescence in the disease process. Similar to PD, an overall reduction in the proportion of total T and B lymphocytes has been reported in AD patients compared to matched controls [68]. Larbi et al. further demonstrated a marked reduction in naïve T cells with a parallel increase in both effector memory and TEMRA T cells in a small cohort of AD patients compared to age-matched controls. The same study also showed a pronounced decrease in the expression of the activation marker CD28. These changes were observed in CD4 but not CD8 subsets [69]. A more recent study demonstrated increased proportions of CD8 TEMRAs (identified as CD45RA+CD27–) in the blood of AD patients compared to controls. The authors showed that a higher percentage of senescent CD8 TEMRAs in the blood of patients was significantly associated with worse cognitive function. Importantly, single-cell RNA sequencing revealed for the first time a significant increase of clonally expanded CD8 TEMRAs in the CSF of patients versus controls [70]. Taken together, this data suggests an increase in T cell immunosenescence in AD compared to controls, which is in contrast to the current evidence indicating reduced CD8 immunosenescence in PD. Hence immunosenescence may be contributing in differing ways to the pathogenesis of PD and AD.
However, there may be some commonality in terms of alternations in the CD8 T cell response to CMV in AD and PD. As previously discussed, CMV infection is considered a major driver of immunosenescence. Westman et al. showed that latent CMV infection was associated with a decreased proportion of CMV-specific CD8 T lymphocytes in AD patients compared to controls, [71] mirroring our findings of a reduced senescent shift in the T cell population in response to CMV infection in PD [29, 30]. The same authors later showed that upon stimulation with T cell activating stimuli (anti-CD3/CD28 beads), PBMCs of CMV seropositive AD patients have a more inflammatory cytokine secretion profile compared to seropositive controls [72] providing further evidence of reduced immunosenescence.
Whilst there is contradicting data on telomere shortening in PD, the majority of studies in AD are in agreement, consistently showing shortening of telomeres in patients compared to age-matched controls. This was demonstrated in a meta-analysis including 860 patients and 2022 healthy subjects across 13 studies (9 of which were done in leucocytes) [73]. The same authors performed a separate meta-analysis across 8 PD studies, concluding that there is no consistent evidence of telomere shortening in PD [74].
HOW MIGHT ALTERATIONS IN T CELL SENESCENCE IMPACT ON THE PATHOGENESIS OF PD?
The most consistent finding to date in terms of age-related immune changes in PD is that CD8 T cell senescence is reduced, raising the possibility that immunosenescence is protective against PD. However, the mechanisms underlying this observation and its impact on the disease are unclear.
A reduction in senescent late-differentiated T cell populations in PD may simply reflect a shift towards a more activated T cell profile. As a consequence, this could lead to a more pronounced immune response to disease-associated antigens such as oligomeric alpha-synuclein. Work by Sulzer and colleagues has demonstrated the recognition of alpha-synuclein epitopes by autoreactive T lymphocytes in PD [75]. The same authors later showed that the levels of alpha-synuclein specific T cells are highest at the time of PD diagnosis, but could be present years prior to motor symptom development [76], and that they have a very diverse repertoire of T cell receptors [77]. This seems to be accompanied by an elevation of alpha-synuclein auto-antibodies in early stages of PD that wanes as the disease progresses, according to our previous meta-analysis [78]. Taken together, this data suggests that the reduced ability to respond to new pathogens, caused by the replicative senescence of T lymphocytes which occurs with normal ageing, could be protective in the context of PD.
The next question is why there is a reduced senescent shift in the T cell population in aged PD patients. It is possible that this relates to inherent differences in the adaptive immune system which alter the risk of PD. This idea is supported by the well described genetic association between immune loci, particularly those involved in antigen presentation such as the Human Leucocyte Antigen region, and PD risk [79, 80]. These inherent differences could involve an altered response to viral infections, or changes in cell death mechanisms. We and others have shown that in the context of prior CMV infection (indicated by CMV IgG positivity), the expected accumulation of CD8 senescent T cells is not observed in PD patients [29, 30, 32]. This suggests that the T cell response to chronic viral antigen exposure in earlier life may differ in those who will go on to later develop PD. Another possibility is that senescent CD8 T cells in PD are more apoptotic, so have shorter life spans, and as a consequence their numbers are more depleted. Culturing T cells from PD patients both in the absence and presence of stimulation has indeed shown increased levels of apoptosis (as measured by the expression of annexin-V) when compared to cells from healthy individuals [81]. This effect was more pronounced in CD4 subsets, but a similar trend was seen in CD8 T cells. However, the mechanism involved in increased lymphocytic cell death in PD, is not known, hence further functional studies in specific CD8 senescent populations are warranted.
Another important consideration is that the significance of the change in terminally differentiated CD8 cells in PD may not relate to the replicative senescence of these cells but to their cytotoxic capacity. CD8 TEMRAs remain highly cytotoxic on stimulation by their specific target antigen and they are known to contribute to certain disease states, for example they have been implicated in driving graft failure in renal transplant patients [82]. In the context of PD, antigen-specific CD8 TEMRAs could be trafficked out of the blood via the choroid plexus and into the brain where their target antigens (e.g., alpha-synuclein epitopes) are expressed, and induce neurotoxicity. Hence terminally differentiated, so-called “senescent” CD8 cells may be best considered as highly specific effector cells which are not truly reduced in number, but rather sequestered elsewhere to exert their cytotoxic function. In support of this idea, animal studies have previously demonstrated that T lymphocytes in the blood are able to transmigrate across the choroidal epithelium and enter the central nervous system [83]. Furthermore, a single-cell transcriptomic study showed increased clonal expansion of CD8 T cells in the CSF of patients compared to controls, suggesting that a higher proportion of antigen experienced CD8 T cells are circulating in the PD CSF [33]. The immune cell composition of the CSF in PD is not yet clear, but Schröder and colleagues have found a higher proportion of total T lymphocytes in PD versus control CSF. This increase seemed to be driven by a higher percentage of activated CD8 T cells (CD8+ HLA+) [84].
These findings are also in line with postmortem brain studies; we and others have previously demonstrated significantly higher numbers of CD8 and CD4 T lymphocytes infiltrating into the parenchyma of postmortem PD brains compared to controls [85–87]. However, the function of the infiltrating T cells is not yet clear and future studies are necessary for a more extensive characterization of their phenotype and antigen specificity.
Immunosenescence has been found to be influenced by physical activity, an observation which warrants some further consideration in the context of PD. When sustained through life, physical activity can enhance the percentage of naïve T lymphocyte population and reverse the CD4+ CD45RA+/CD4+ CD45RO+ ratio [88], alongside other immune changes (reviewed in [89]). In the context of PD, epidemiological studies have shown that physical activity is linked with improvements in both movement (e.g., gait speed, balance) [90, 91] and cognitive/neuropsychiatric symptoms, such as global cognition [92, 93] and depression [94], and may have the potential to slow down the progression of the disease. It is possible that the benefits of exercise in PD relate in part be due to modulation of the immune system, and hence further research to explore the relationship between exercise, immune ageing and PD would be of interest.
CONCLUSION
In conclusion, the theory that age-related immune changes may play a contributory role in PD is highly plausible, but studies investigating immunosenescence in PD have been limited to date. The most consistent finding is that “senescent” CD8 T cells, including CD8 TEMRAs and CD8 CD28loCD57hi cells, are reduced in the PD blood, a finding which may seem paradoxical given that ageing is a major risk factor for PD. However, a possible interpretation of these findings is that the typical shift towards more senescent CD8 T cells in healthy ageing confers protection against PD (and other similar neurodegenerative diseases), whilst impaired immunosenescence, perhaps due to an inherited trait, predisposes to an over-active response to newly encountered disease-related antigens (Fig. 1). Whilst it is also possible that the observed reduction in late-differentiated T cells in the blood in PD reflects sequestration to the brain to exert effector functions, evidence to support transition of this specific cell population to the central nervous system is lacking. In addition to changes in the CD8 profile, chronic low-grade inflammation is another consistent finding in PD, which is also a well-recognised component of immune ageing. However, there are several potential mechanistic drivers of this inflammation in PD, and hence it cannot necessarily be attributed to increased “inflammageing”. Alternative measures of immunosenescence such as thymic involution have not been well studied in the context of PD. Further studies investigating immunosenescence in PD are clearly warranted. Addressing the question of how ageing interacts with immune activation in PD will be fundamental to our understanding of the immune component of this disorder, with potential implications for future targeted immune therapies.
Fig. 1
Schematic illustration showing a hypothesized relationship between immunosenescence and Parkinson’s disease risk/progression. With advancing age there is progressive decline in the size and function of the thymus gland (thymic involution), as well as increased exposure to viruses such as cytomegalovirus. This leads to immunosenescence, characterized by a reduction in antigen-inexperienced naïve T cells and an increase in “senescent” terminally differentiated effector T cells (TEMRA). The senescent shift is associated with a weakened immune response to novel antigens. However, in people who are predisposed to develop Parkinson’s disease, the typical age-associated shift towards senescence in the CD8 T cell population may be attenuated, with a reduced accumulation of CD8 TEMRA T cells in these individuals. This could lead to a heightened immune response to newly encountered antigens (such as misfolded alpha-synuclein), thereby increasing the risk of developing Parkinson’s and/or promoting more rapid disease progression. CMV, Cytomegalovirus; PD, Parkinson’s disease.
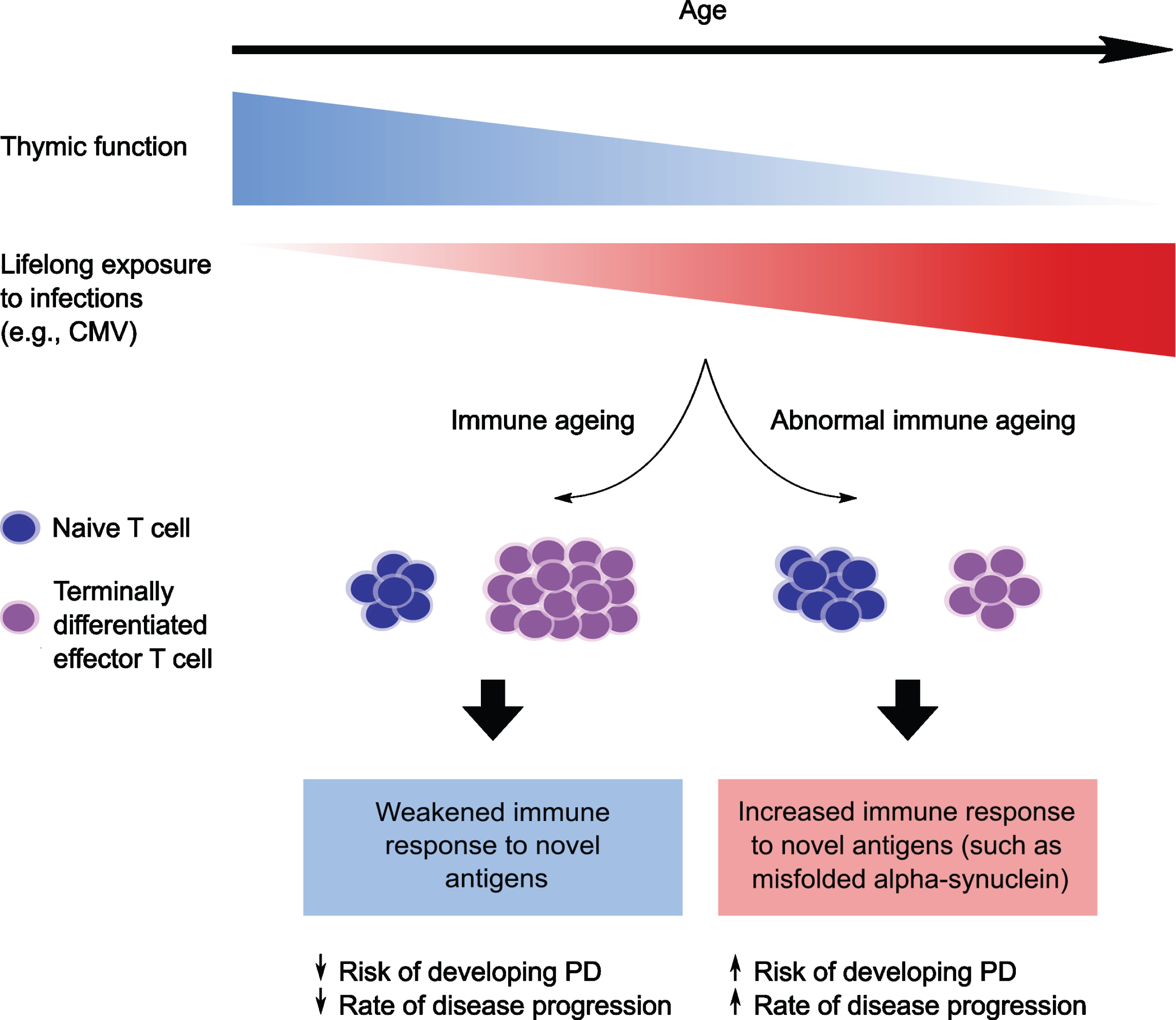
ACKNOWLEDGMENTS
The authors are supported by a RCUK/UKRI Research Innovation Fellowship awarded by the Medical Research Council (MR/R007446/1), the Cambridge Centre for Parkinson-Plus and the NIHR Cambridge Biomedical Research Centre (BRC-1215-20014). The views expressed are those of the authors and not necessarily those of the NHS, the NIHR or the Department of Health. The authors’ own studies on immunosenescence in PD, referred to in this article, were also supported by the Michael J Fox Foundation.
For the purpose of open access, the author has applied a Creative Commons Attribution (CC BY) licence to any Author Accepted Manuscript version arising from this submission.
CONFLICT OF INTEREST
The authors have no conflict of interest to report.
REFERENCES
[1] | Fajemiroye JO , Cunha da LC , Saavedra-Rodríguez R , Rodrigues KL , Naves LM , Mourão AA , Silva da EF , Williams NEE , Martins JLR , Sousa RB , Rebelo ACS , Reis da AA S , Santos da R S , Ferreira-Neto ML , Pedrino GR ((2018) ) Aging-induced biological changes and cardiovascular diseases. Biomed Res Int 2018: , e7156435. |
[2] | Laconi E , Marongiu F , DeGregori J ((2020) ) Cancer as a disease of old age: Changing mutational and microenvironmental landscapes. Br J Cancer 122: , 943–952. |
[3] | Hou Y , Dan X , Babbar M , Wei Y , Hasselbalch SG , Croteau DL , Bohr VA ((2019) ) Ageing as a risk factor for neurodegenerative disease. Nat Rev Neurol 15: , 565–581. |
[4] | Goodwin K , Viboud C , Simonsen L ((2006) ) Antibody response to influenza vaccination in the elderly: A quantitative review. Vaccine 24: , 1159–1169. |
[5] | Goronzy JJ , Weyand CM ((2012) ) Immune aging and autoimmunity. Cell Mol Life Sci 69: , 1615–1623. |
[6] | Williams-Gray CH , Wijeyekoon R , Yarnall AJ , Lawson RA , Breen DP , Evans JR , Cummins GA , Duncan GW , Khoo TK , Burn DJ , Barker RA , on behalf of the ICICLE-PD study group ((2016) ) Serum immune markers and disease progression in an incident Parkinson’s disease cohort (ICICLE-PD). Mov Disord 31: , 995–1003. |
[7] | Wijeyekoon RS , Kronenberg-Versteeg D , Scott KM , Hayat S , Kuan W-L , Evans JR , Breen DP , Cummins G , Jones JL , Clatworthy MR , Floto RA , Barker RA , Williams-Gray CH ((2020) ) Peripheral innate immune and bacterial signals relate to clinical heterogeneity in Parkinson’s disease. Brain Behav Immun 87: , 473–488. |
[8] | Saunders JAH , Estes KA , Kosloski LM , Allen HE , Dempsey KM , Torres-Russotto DR , Meza JL , Santamaria PM , Bertoni JM , Murman DL , Ali HH , Standaert DG , Mosley RL , Gendelman HE ((2012) ) CD4+regulatory and effector/memory T cell subsets profile motor dysfunction in Parkinson’s disease. J Neuroimmune Pharmacol 7: , 927–938. |
[9] | Parkinson’s UK (2017) Incidence and Prevalence of Parkinson’s in theUK- Results from the Clinical Practice Research Datalink Summary Report. |
[10] | Rodriguez IJ , Lalinde Ruiz N , Llano León M , Martínez Enríquez L , Montilla Velásquez del M P , Ortiz Aguirre JP , Rodríguez Bohórquez OM , Velandia Vargas EA , Hernández ED , Parra López CA ((2021) ) Immunosenescence study of T cells: A systematic review. Front Immunol 11: , 3460. |
[11] | Chou JP , Effros RB ((2013) ) T cell replicative senescence in human aging. Curr Pharm Des 19: , 1680–1698. |
[12] | Larbi A , Fulop T ((2014) ) From “truly naïve” to “exhaustedsenescent” T cells: When markers predict functionality. Cytometry A 85: , 25–35. |
[13] | Focosi D , Bestagno M , Burrone O , Petrini M ((2010) ) CD57+T lymphocytes and functional immune deficiency. J Leukoc Biol 87: , 107–116. |
[14] | Brenchley JM , Karandikar NJ , Betts MR , Ambrozak DR , Hill BJ , Crotty LE , Casazza JP , Kuruppu J , Migueles SA , Connors M , Roederer M , Douek DC , Koup RA ((2003) ) Expression of CD57 defines replicative senescence and antigen-induced apoptotic death of CD8+T cells. Blood 101: , 2711–2720. |
[15] | Effros RB , Boucher N , Porter V , Zhu X , Spaulding C , Walford RL , Kronenberg M , Cohen D , Schächter F ((1994) ) Decline in CD28+T cells in centenarians and in long-term T cell cultures: A possible cause for both in vivo and in vitro immunosenescence. Exp Gerontol 29: , 601–609. |
[16] | Akbar AN , Henson SM , Lanna A ((2016) ) Senescence of T lymphocytes: Implications for enhancing human immunity. Trends Immunol 37: , 866–876. |
[17] | Geginat J , Lanzavecchia A , Sallusto F ((2003) ) Proliferation and differentiation potential of human CD8+memory T-cell subsets in response to antigen or homeostatic cytokines. Blood 101: , 4260–4266. |
[18] | Xu W , Larbi A ((2017) ) Markers of T cell senescence in humans. Int J Mol Sci 18: , 1742. |
[19] | van der Heiden M , van Zelm MC , Bartol SJW , de Rond LGH , Berbers GAM , Boots AMH , Buisman A-M ((2016) ) Differential effects of Cytomegalovirus carriage on the immune phenotype of middle-aged males and females. Sci Rep 6: , 26892. |
[20] | Derhovanessian E , Maier AB , Hähnel K , Beck R , de Craen AJM , Slagboom EP , Westendorp RGJ , Pawelec G ((2011) ) Infection with cytomegalovirus but not herpes simplex virus induces the accumulation of late-differentiated CD4+and CD8+T-cells in humans. J Gen Virol 92: , 2746–2756. |
[21] | Khan N , Shariff N , Cobbold M , Bruton R , Ainsworth JA , Sinclair AJ , Nayak L , Moss PAH ((2002) ) Cytomegalovirus seropositivity drives the CD8 T cell repertoire toward greater clonality in healthy elderly individuals. J Immunol 169: , 1984–1992. |
[22] | Hadrup SR , Strindhall J , Køllgaard T , Seremet T , Johansson B , Pawelec G , Straten thor P , Wikby A ((2006) ) Longitudinal studies of clonally expanded CD8 T cells reveal a repertoire shrinkage predicting mortality and an increased number of dysfunctional cytomegalovirus-specific T cells in the very elderly. J Immunol 176: , 2645–2653. |
[23] | Hakim FT , Gress RE ((2007) ) Immunosenescence: Deficits in adaptive immunity in the elderly. Tissue Antigens 70: , 179–189. |
[24] | Palmer DB ((2013) ) The effect of age on thymic function. Front Immunol 4: , 316. |
[25] | Shiraishi J , Utsuyama M , Seki S , Akamatsu H , Sunamori M , Kasai M , Hirokawa K ((2003) ) Essential microenvironment for thymopoiesis is preserved in human adult and aged thymus. Clin Dev Immunol 10: , 53–59. |
[26] | Lynch HE , Goldberg GL , Chidgey A , Van den Brink MRM , Boyd R , Sempowski GD ((2009) ) Thymic involution and immune reconstitution. Trends Immunol 30: , 366–373. |
[27] | Trollor JN , Smith E , Agars E , Kuan SA , Baune BT , Campbell L , Samaras K , Crawford J , Lux O , Kochan NA , Brodaty H , Sachdev P ((2012) ) The association between systemic inflammation and cognitive performance in the elderly: The Sydney Memory and Ageing Study. Age 34: , 1295–1308. |
[28] | Alberro A , Iribarren-Lopez A , Sáenz-Cuesta M , Matheu A , Vergara I , Otaegui D ((2021) ) Inflammaging markers characteristic of advanced age show similar levels with frailty and dependency. Sci Rep 11: , 4358. |
[29] | Williams-Gray CH , Wijeyekoon RS , Scott KM , Hayat S , Barker RA , Jones JL ((2018) ) Abnormalities of age-related T cell senescence in Parkinson’s disease. J Neuroinflammation 15: , 166. |
[30] | Kouli A , Jensen M , Papastavrou V , Scott KM , Kolenda C , Parker C , Solim IH , Camacho M , Martin-Ruiz C , Williams-Gray CH ((2021) ) T lymphocyte senescence is attenuated in Parkinson’s disease. J Neuroinflammation 18: , 228. |
[31] | Vandenberk B , Brouwers B , Hatse S , Wildiers H ((2011) ) p16INK4a: Acentral player in cellular senescence and a promising agingbiomarker in elderly cancer patients. J Geriatr Oncol 2: , 259–269. |
[32] | Vavilova JD , Boyko AA , Ponomareva NV , Fokin VF , Fedotova EY , Streltsova MA , Kust SA , Grechikhina MV , Bril EV , Zimnyakova OS , Kovalenko EI , Sapozhnikov AM ((2021) ) Reduced immunosenescence of peripheral blood T cells in Parkinson’s disease with CMV infection background. Int J Mol Sci 22: , 13119. |
[33] | Wang P , Yao L , Luo M , Zhou W , Jin X , Xu Z , Yan S , Li Y , Xu C , Cheng R , Huang Y , Lin X , Ma K , Cao H , Liu H , Xue G , Han F , Nie H , Jiang Q ((2021) ) Single-cell transcriptome and TCR profiling reveal activated and expanded T cell populations in Parkinson’s disease. Cell Discov 7: , 52. |
[34] | Moro-García MA , Alonso-Arias R , Lopez-Larrea C ((2013) ) When aging reaches CD4+T-cells: Phenotypic and functional changes. Front Immunol 4: , 107. |
[35] | Baba Y , Kuroiwa A , Uitti RJ , Wszolek ZK , Yamada T ((2005) ) Alterations of T-lymphocyte populations in Parkinson disease. Parkinsonism Relat Disord 11: , 493–498. |
[36] | Bas J , Calopa M , Mestre M , Mollevı DG , Cutillas B , Ambrosio S , Buendia E ((2001) ) Lymphocyte populations in Parkinson’s disease andin rat models of parkinsonism. J Neuroimmunol 113: , 146–152. |
[37] | Li R , Tropea TF , Baratta LR , Zuroff L , Diaz-Ortiz ME , Zhang B , Shinoda K , Rezk A , Alcalay RN , Chen-Plotkin A , Bar-Or A ((2022) ) Abnormal B-cell and Tfh-cell profiles in patients with Parkinson disease: A cross-sectional study. Neurol Neuroimmunol Neuroinflamm 9: , e1125. |
[38] | Jing Y , Shaheen E , Drake RR , Chen N , Gravenstein S , Deng Y ((2009) ) Aging is associated with a numerical and functional decline in plasmacytoid dendritic cells, whereas myeloid dendritic cells are relatively unaltered in human peripheral blood. Hum Immunol 70: , 777–784. |
[39] | Panda A , Qian F , Mohanty S , van Duin D , Newman FK , Zhang L , Chen S , Towle V , Belshe RB , Fikrig E , Allore HG , Montgomery RR , Shaw AC ((2010) ) Age-associated decrease in TLR function in primary human dendritic cells predicts influenza vaccine response. J Immunol 184: , 2518–2527. |
[40] | Dutertre C-A , Becht E , Irac SE , Khalilnezhad A , Narang V , Khalilnezhad S , Ng PY , van den Hoogen LL , Leong JY , Lee B , Chevrier M , Zhang XM , Yong PJA , Koh G , Lum J , Howland SW , Mok E , Chen J , Larbi A , Tan HKK , Lim TKH , Karagianni P , Tzioufas AG , Malleret B , Brody J , Albani S , van Roon J , Radstake T , Newell EW , Ginhoux F ((2019) ) Single-cell analysis of human mononuclear phagocytes reveals subset-defining markers and identifies circulating inflammatory dendritic cells.. Immunity 51: , 573–589.e8. |
[41] | Konstantin Nissen S , Farmen K , Carstensen M , Schulte C , Goldeck D , Brockmann K , Romero-Ramos M ((2022) ) Changes in CD163+, CD11b+, and CCR2+peripheral monocytes relate to Parkinson’s disease and cognition. Brain Behav Immun 101: , 182–193. |
[42] | Ciaramella A , Salani F , Bizzoni F , Pontieri FE , Stefani A , Pierantozzi M , Assogna F , Caltagirone C , Spalletta G , Bossù P ((2013) ) Blood dendritic cell frequency declines in idiopathic Parkinson’s disease and is associated with motor symptom severity. PLoS One 8: , e65352. |
[43] | Álvarez-Luquín DD , Arce-Sillas A , Leyva-Hernández J , Sevilla-Reyes E , Boll MC , Montes-Moratilla E , Vivas-Almazán V , Pérez-Correa C , Rodríguez-Ortiz U , Espinoza-Cárdenas R , Fragoso G , Sciutto E , Adalid-Peralta L ((2019) ) Regulatory impairment in untreated Parkinson’s disease is not restricted to Tregs: Other regulatory populations are also involved. J Neuroinflammation 16: , 212. |
[44] | Frasca D , Diaz A , Romero M , Blomberg BB ((2017) ) Human peripheral late/exhausted memory B cells express a senescent-associated secretory phenotype and preferentially utilize metabolic signaling pathways. Exp Gerontol 87: , 113–120. |
[45] | Monteiro J , Batliwalla F , Ostrer H , Gregersen PK ((1996) ) Shortened telomeres in clonally expanded CD28-CD8+T cells imply a replicative history that is distinct from their CD28+CD8+counterparts. J Immunol 156: , 3587–3590. |
[46] | Vallejo AN ((2005) ) CD28 extinction in human T cells: Altered functions and the program of T-cell senescence. Immunol Rev 205: , 158–169. |
[47] | Cawthon RM , Smith KR , O’Brien E , Sivatchenko A , Kerber RA ((2003) ) Association between telomere length in blood and mortality in people aged 60 years or older. Lancet 361: , 393–395. |
[48] | Hudson G , Faini D , Stutt A , Eccles M , Robinson L , Burn DJ , Chinnery PF ((2011) ) No evidence of substantia nigra telomere shortening in Parkinson’s disease. Neurobiol Aging 32: , 2107.e3–5. |
[49] | Degerman S , Domellöf M , Landfors M , Linder J , Lundin M , Haraldsson S , Elgh E , Roos G , Forsgren L ((2014) ) Long leukocyte telomere length at diagnosis is a risk factor for dementia progression in idiopathic parkinsonism. PLoS One 9: , e113387. |
[50] | Martin-Ruiz C , Williams-Gray CH , Yarnall AJ , Boucher JJ , Lawson RA , Wijeyekoon RS , Barker RA , Kolenda C , Parker C , Burn DJ , Von Zglinicki T , Saretzki G ((2020) ) Senescence and inflammatory markers for predicting clinical progression in Parkinson’s disease: The ICICLE-PD Study. J Parkinsons Dis 10: , 193–206. |
[51] | Haines CJ , Giffon TD , Lu L-S , Lu X , Tessier-Lavigne M , Ross DT , Lewis DB ((2009) ) Human CD4+T cell recent thymic emigrants are identified by protein tyrosine kinase 7 and have reduced immune function. J Exp Med 206: , 275–285. |
[52] | McFarland RD , Douek DC , Koup RA , Picker LJ ((2000) ) Identification of a human recent thymic emigrant phenotype. Proc Natl Acad Sci U S A 97: , 4215–4220. |
[53] | Qin X-Y , Zhang S-P , Cao C , Loh YP , Cheng Y ((2016) ) Aberrations in peripheral inflammatory cytokine levels in Parkinson disease: A systematic review and meta-analysis. JAMA Neurol 73: , 1316–1324. |
[54] | King E , O’Brien JT , Donaghy P , Morris C , Barnett N , Olsen K , Martin-Ruiz C , Taylor J-P , Thomas AJ ((2018) ) Peripheral inflammation in prodromal Alzheimer’s and Lewy body dementias. J Neurol Neurosurg Psychiatry 89: , 339–345. |
[55] | Postuma RB , Iranzo A , Hu M , Högl B , Boeve BF , Manni R , Oertel WH , Arnulf I , Ferini-Strambi L , Puligheddu M , Antelmi E , Cochen De Cock V , Arnaldi D , Mollenhauer B , Videnovic A , Sonka K , Jung K-Y , Kunz D , Dauvilliers Y , Provini F , Lewis SJ , Buskova J , Pavlova M , Heidbreder A , Montplaisir JY , Santamaria J , Barber TR , Stefani A , St.Louis EK , Terzaghi M , Janzen A , Leu-Semenescu S , Plazzi G , Nobili F , Sixel-Doering F , Dusek P , Bes F , Cortelli P , Ehgoetz Martens K , Gagnon J-F , Gaig C , Zucconi M , Trenkwalder C , Gan-Or Z , Lo C , Rolinski M , Mahlknecht P , Holzknecht E , Boeve AR , Teigen LN , Toscano G , Mayer G , Morbelli S , Dawson B , Pelletier A ((2019) ) Risk and predictors of dementia and parkinsonism in idiopathic REM sleep behaviour disorder: A multicentre study. Brain 142: , 744–759. |
[56] | Schenck CH , Boeve BF , Mahowald MW ((2013) ) Delayed emergence of a parkinsonian disorder or dementia in 81% of older men initially diagnosed with idiopathic rapid eye movement sleep behavior disorder: A 16-year update on a previously reported series. Sleep Med 14: , 744–748. |
[57] | Mondello S , Kobeissy F , Mechref Y , Zhao J , Talih FR , Cosentino F , Antelmi E , Moresco M , Plazzi G , Ferri R ((2018) ) Novel biomarker signatures for idiopathic REM sleep behavior disorder: A proteomic and system biology approach. Neurology 91: , e1710–e1715. |
[58] | Hu WT , Howell JC , Ozturk T , Gangishetti U , Kollhoff AL , Hatcher-Martin JM , Anderson AM , Tyor WR ((2019) ) CSF cytokines in aging, multiple sclerosis, and dementia. Front Immunol 10: , 480. |
[59] | Yu S , Zuo L , Wang F , Chen Z , Hu Y , Wang Y , Wang X , Zhang W ((2014) ) Potential biomarkers relating pathological proteins, neuroinflammatory factors and free radicals in PD patients with cognitive impairment: A cross-sectional study. BMC Neurol 14: , 113. |
[60] | Janelidze S , Lindqvist D , Francardo V , Hall S , Zetterberg H , Blennow K , Adler CH , Beach TG , Serrano GE , van Westen D , Londos E , Cenci MA , Hansson O ((2015) ) Increased CSF biomarkers of angiogenesis in Parkinson disease. Neurology 85: , 1834–1842. |
[61] | Hall S , Janelidze S , Surova Y , Widner H , Zetterberg H , Hansson O ((2018) ) Cerebrospinal fluid concentrations of inflammatory markers in Parkinson’s disease and atypical parkinsonian disorders. Sci Rep 8: , 13276. |
[62] | Lindqvist D , Hall S , Surova Y , Nielsen HM , Janelidze S , Brundin L , Hansson O ((2013) ) Cerebrospinal fluid inflammatory markers in Parkinson’s disease - Associations with depression, fatigue, and cognitive impairment. Brain Behav Immun 33: , 183–189. |
[63] | Karayel O , Winter SV , Padmanabhan S , Kuras YI , Vu DT , Tuncali I , Merchant K , Wills A-M , Scherzer CR , Mann M (2021) Proteome profiling of cerebrospinal fluid reveals novel biomarker candidates for Parkinson’s disease. bioRxiv, 2021.07.22.453322. |
[64] | Lobanova E , Whiten D , Ruggeri FS , Taylor C , Kouli A , Xia Z , Emin D , Zhang YP , Lam JYL , Williams-Gray CH , Klenerman D ((2021) ) Imaging protein aggregates in the serum and cerebrospinal fluid in Parkinson’s disease. Brain 145: , 632–643. |
[65] | Kouli A , Horne CB , Williams-Gray CH ((2019) ) Toll-like receptors and their therapeutic potential in Parkinson’s disease and α-synucleinopathies. Brain Behav Immun 81: , 41–51. |
[66] | Sampson TR , Debelius JW , Thron T , Janssen S , Shastri GG , Ilhan ZE , Challis C , Schretter CE , Rocha S , Gradinaru V , Chesselet M-F , Keshavarzian A , Shannon KM , Krajmalnik-Brown R , Wittung-Stafshede P , Knight R , Mazmanian SK ((2016) ) Gut microbiota regulate motor deficits and neuroinflammation in a model of Parkinson’s disease. Cell 167: , 1469–1480.e12. |
[67] | Houser MC , Tansey MG ((2017) ) The gut-brain axis: Is intestinal inflammation a silent driver of Parkinson’s disease pathogenesis? NPJ Parkinsons Dis 3: , 3. |
[68] | Richartz-Salzburger E , Batra A , Stransky E , Laske C , Köhler N , Bartels M , Buchkremer G , Schott K ((2007) ) Altered lymphocyte distribution in Alzheimer’s disease. J Psychiatr Res 41: , 174–178. |
[69] | Larbi A , Pawelec G , Witkowski JM , Schipper HM , Derhovanessian E , Goldeck D , Fulop T ((2009) ) Dramatic shifts in circulating CD4 but not CD8 T cell subsets in mild Alzheimer’s disease. J Alzheimers Dis 17: , 91–103. |
[70] | Gate D , Saligrama N , Leventhal O , Yang AC , Unger MS , Middeldorp J , Chen K , Lehallier B , Channappa D , De Los Santos MB , McBride A , Pluvinage J , Elahi F , Tam GK-Y , Kim Y , Greicius M , Wagner AD , Aigner L , Galasko DR , Davis MM , Wyss-Coray T ((2020) ) Clonally expanded CD8 T cells patrol the cerebrospinal fluid in Alzheimer’s disease. Nature 577: , 399–404. |
[71] | Westman G , Lidehall A-K , Magnusson P , Ingelsson M , Kilander L , Lannfelt L , Korsgren O , Eriksson B-M ((2013) ) Decreased proportion of cytomegalovirus specific CD8 T-cells but no signs of general immunosenescence in Alzheimer’s disease.e. PLoS One 8: , 77921. |
[72] | Westman G , Berglund D , Widén J , Ingelsson M , Korsgren O , Lannfelt L , Sehlin D , Lidehall A-K , Eriksson B-M ((2014) ) Increased inflammatory response in cytomegalovirus seropositive patients with Alzheimer’s disease. PLoS One 9: , e96779. |
[73] | Forero DA , González-Giraldo Y , López-Quintero C , Castro-Vega LJ , Barreto GE , Perry G ((2016) ) Meta-analysis of telomere length in Alzheimer’s disease. J Gerontol A Biol Sci Med Sci 71: , 1069–1073. |
[74] | Forero DA , González-Giraldo Y , López-Quintero C , Castro-Vega LJ , Barreto GE , Perry G ((2016) ) Telomere length in Parkinson’s disease: A meta-analysis. Exp Gerontol 75: , 53–55. |
[75] | Sulzer D , Alcalay RN , Garretti F , Cote L , Kanter E , Agin-Liebes J , Liong C , McMurtrey C , Hildebrand WH , Mao X , Dawson VL , Dawson TM , Oseroff C , Pham J , Sidney J , Dillon MB , Carpenter C , Weiskopf D , Phillips E , Mallal S , Peters B , Frazier A , Arlehamn CSL , Sette A ((2017) ) T cells from patients with Parkinson’s disease recognize α-synuclein peptides. Nature 546: , 656. |
[76] | Lindestam Arlehamn CS , Dhanwani R , Pham J , Kuan R , Frazier A , Rezende Dutra J , Phillips E , Mallal S , Roederer M , Marder KS , Amara AW , Standaert DG , Goldman JG , Litvan I , Peters B , Sulzer D , Sette A ((2020) ) α-Synuclein-specific T cell reactivity is associated with preclinical and early Parkinson’s disease. Nat Commun 11: , 1875. |
[77] | Singhania A , Pham J , Dhanwani R , Frazier A , Rezende Dutra J , Marder KS , Phillips E , Mallal S , Amara AW , Standaert DG , Sulzer D , Peters B , Sette A , Lindestam Arlehamn CS ((2021) ) The TCR repertoire of α-synuclein-specific T cells in Parkinson’s disease is surprisingly diverse. Sci Rep 11: , 302. |
[78] | Scott KM , Kouli A , Yeoh SL , Clatworthy MR , Williams-Gray CH ((2018) ) A systematic review and meta-analysis of alpha synuclein auto-antibodies in Parkinson’s disease. Front Neurol 9: , 815. |
[79] | Saiki M , Baker A , Williams-Gray CH , Foltynie T , Goodman RS , Taylor CJ , Compston DAS , Barker RA , Sawcer SJ , Goris A ((2010) ) Association of the human leucocyte antigen region with susceptibility to Parkinson’s disease. J Neurol Neurosurg Psychiatry 81: , 890–891. |
[80] | Hamza TH , Zabetian CP , Tenesa A , Laederach A , Montimurro J , Yearout D , Kay DM , Doheny KF , Paschall J , Pugh E , Kusel VI , Collura R , Roberts J , Griffith A , Samii A , Scott WK , Nutt J , Factor SA , Payami H ((2010) ) Common genetic variation in the HLA region is associated with late-onset sporadic Parkinson’s disease. Nat Genet 42: , 781–785. |
[81] | Calopa M , Bas J , Callén A , Mestre M ((2010) ) Apoptosis of peripheral blood lymphocytes in Parkinson patients. Neurobiol Dis 38: , 1–7. |
[82] | Jacquemont L , Tilly G , Yap M , Doan-Ngoc T-M , Danger R , Guérif P , Delbos F , Martinet B , Giral M , Foucher Y , Brouard S , Degauque N ((2020) ) Terminally differentiated effector memory CD8+T cells identify kidney transplant recipients at high risk of graft failure. J Am Soc Nephrol 31: , 876–891. |
[83] | Strazielle N , Creidy R , Malcus C , Boucraut J , Ghersi-Egea J-F ((2016) ) T-lymphocytes traffic into the brain across the blood-CSF barrier: Evidence using a reconstituted choroid plexus epithelium. PLoS One 11: , e0150945. |
[84] | Schröder JB , Pawlowski M , Meyerzu Hörste G , Gross CC , Wiendl H , Meuth SG , Ruck T , Warnecke T ((2018) ) Immune cell activation in the cerebrospinal fluid of patients with Parkinson’s disease. Front Neurol 9: , 1081. |
[85] | Brochard V , Combadière B , Prigent A , Laouar Y , Perrin A , Beray-Berthat V , Bonduelle O , Alvarez-Fischer D , Callebert J , Launay J-M , Duyckaerts C , Flavell RA , Hirsch EC , Hunot S ((2009) ) Infiltration of CD4+lymphocytes into the brain contributes to neurodegeneration in a mouse model of Parkinson disease. J Clin Invest 119: , 182–192. |
[86] | Kouli A , Camacho M , Allinson K , Williams-Gray CH ((2020) ) Neuroinflammation and protein pathology in Parkinson’s disease dementia. Acta Neuropathol Commun 8: , 211. |
[87] | Galiano-Landeira J , Torra A , Vila M , Bové J ((2020) ) CD8 T cell nigral infiltration precedes synucleinopathy in early stages of Parkinson’s disease. Brain 143: , 3717–3733. |
[88] | Tylutka A , Morawin B , Gramacki A , Zembron-Lacny A ((2021) ) Lifestyle exercise attenuates immunosenescence; flow cytometry analysis. BMC Geriatr 21: , 200. |
[89] | Duggal NA , Niemiro G , Harridge SDR , Simpson RJ , Lord JM ((2019) ) Can physical activity ameliorate immunosenescence and thereby reduce age-related multi-morbidity? Nat Rev Immunol 19: , 563–572. |
[90] | Goodwin VA , Richards SH , Taylor RS , Taylor AH , Campbell JL ((2008) ) The effectiveness of exercise interventions for people with Parkinson’s disease: A systematic review and meta-analysis. Mov Disord 23: , 631–640. |
[91] | Lauzé M , Daneault J-F , Duval C ((2016) ) The effects of physical activity in Parkinson’s disease: A review. J Parkinsons Dis 6: , 685–698. |
[92] | Hindle JV , Petrelli A , Clare L , Kalbe E ((2013) ) Nonpharmacological enhancement of cognitive function in Parkinson’s disease: A systematic review. Mov Disord 28: , 1034–1049. |
[93] | da Silva FC , Iop da R R , de Oliveira LC , Boll AM , de Alvarenga JGS , Gutierres Filho PJB , de Melo LMAB , Xavier AJ , da Silva R ((2018) ) Effects of physical exercise programs on cognitive function in Parkinson’s disease patients: A systematic review of randomized controlled trials of the last 10 years. PLoS One 13: , e0193113. |
[94] | Wu P-L , Lee M , Huang T-T ((2017) ) Effectiveness of physical activity on patients with depression and Parkinson’s disease: A systematic review. PLoS One 12: , e0181515. |