An Emerging Role for Phosphoinositides in the Pathophysiology of Parkinson’s Disease
Abstract
Recent data support an involvement of defects in homeostasis of phosphoinositides (PIPs) in the pathophysiology of Parkinson’s disease (PD). Genetic mutations have been identified in genes encoding for PIP-regulating and PIP-interacting proteins, that are associated with familial and sporadic PD. Many of these proteins are implicated in vesicular membrane trafficking, mechanisms that were recently highlighted for their close associations with PD. PIPs are phosphorylated forms of the membrane phospholipid, phosphatidylinositol. Their composition in the vesicle’s membrane of origin, as well as membrane of destination, controls vesicular membrane trafficking. We review the converging evidence that points to the involvement of PIPs in PD. The review describes PD- and PIP-associated proteins implicated in clathrin-mediated endocytosis and autophagy, and highlights the involvement of α-synuclein in these mechanisms.
BACKGROUND
Parkinson’s disease (PD) is a heterogeneous neurodegeneration. Disease heterogeneity is reflected both at the clinical and molecular levels. PD is generally considered a sporadic disease of unknown etiology. Nevertheless, in the past two decades, it has become apparent that many cases (about 5–10%) are due to familial, inherited forms of the disease [1]. A growing list of genes and loci has been genetically implicated in the pathogenesis of PD. Classifying these genes, according to their known functions, provides valuable clues about the cellular mechanisms involved in PD. A mechanistic role in vesicular membrane trafficking was recently highlighted for many of the PD-associated proteins (recently reviewed in [2]). The available genetic data also support a role for phosphoinositides (PIP) in the pathophysiology of PD. Central PD-associated genes encode for proteins that regulate PIP homeostasis at cell membranes. Other PD-associated genes encode for proteins that directly interact with PIPs or other PIP-binding, or PIP-metabolizing proteins (Table 1). This review focuses on PD- and PIP-associating proteins that play a role in vesicular membrane trafficking, in particular, clathrin-mediated endocytosis and macroautophagy.
Table 1
PD-associated proteins and their mode of PIP-associations
Pathway | Gene/protein | Mechanism of interaction with PIP | Role in endocytosis/autophagy | Link to PD |
A. PD-associated genes involved in PIP homeostasis | ||||
Endocytosis/autophagy | Synaptojanin1 (SJ1) | A dual PIP phosphatase: a 5’-phosphatase domain and a SAC1 domain [61]. | Growth, maturation and uncoating of clathrin-coated vesicles [19]. Endosomal trafficking; Ultrafast endocytosis [209]; Autophagosome maturation [67, 68]. | PARK20 R258Q [54, 56, 57] and R459P [59] mutations in SAC1 domain; Y832C and R839C mutations in 5-phosphatase domain [60, 266]. |
Endocytosis | INPP5F/Sac2 | PIP 4-phosphatase | Late stages of CME. Recruited to early endosomes by Rab5 [27, 69, 71]. | GWAS risk locus rs117896735 [70, 74]. |
B. PD-associated genes that directly interact with PIP | ||||
Endocytosis/autophagy | SNCA/α-Synuclein (α-Syn) | Increases the steady state levels of PIPs [125, 128]. Binds acidic phospholipids, including PIPs [126, 127]. | Facilitates CME, a role in SV cycling [134, 137, 138] and autophagy [48]. | PARK1, 4 A30P, E46K, A53T, A53E, H50Q, G51D mutations [79–81, 83, 84, 86]. Gene duplication/triplication [76, 78]. Promoter variability [75]. A main component of Lewy-pathology [267]. GWAS risk locus rs2736990,rs356220 [72, 74] |
Endocytosis | DNAJC6/ auxilin 1 | Binds PI3P, PI4P and PI3,4P2 [268, 269]. | recruitS Hsc70 to complete vesicle uncoating in CME [270, 271]. | PARK19 Deletion mutation, splice-site and point R927G mutations [70, 272–275]. |
DNAJC26/ auxilin 2 | Risk factor candidate (rs34311866) [70]. | |||
Receptor mediated endocytosis/autophagy | DNAJC13/RME8 | Binds PI3P, PI3,5P2 and PI3,4,5P3 [276]. | Retrograde transport to the trans-Golgi network (TGN). Endosomal, protein sorting and trafficking [277–281]. | PARK21 N855S mutation [282]. |
Autophagy | ATP13A2 | Interacts with PI3,5P2 in the lysosome [283, 284]. | A lysosomal P-type transporter ATPase [285]. | PARK9 Frame shift (nucleotide deletion) and splice site mutations [286]. |
Mitophagy; Non-vesicular lipid transport | VPS13C | Lipid exchange at membrane contact sites [261]. The yeast homolog binds PI3P [287]. | Acts at MCS to enhance PINK1/Parkin-dependent mitophagy [260]. | PARK23 Truncation mutations [288–292]. |
C. PD-associated genes that interact with other PIP-binding proteins | ||||
Endocytosis/ autophagy | VAC14 | A scaffold for a protein complex containing PIKFYVE kinase, a PI and PI3P 5-kinase. [293, 294]. | Endosomal maturation along the late endosome/lysosome pathway [293–295]. | A562V and W424L mutations [60, 296]. |
Endocytosis/ autophagy | LRRK2 | Protein kinase and GTPase. Phosphorylates the PIP-phosphatase SJ1, and PIP-associating endophilin, auxilin and Rabs proteins [184, 185, 188–190, 297]. | Modulator of CME at multiple steps [184, 191, 192, 297, 298]. Involved in autophagy [193, 212]. | PARK 8 Majority of familial PD cases: G2019S; I2020T ROC GTPase domain: R1441C/G/H COR domain: R1628P; Y1699C GWAS risk locus rs1491923, rs76904798 [72, 173, 175]. |
Endocytosis/ autophagy | SH3GL2/ endophilin A | Binds PIP-interacting proteins, e.g., synaptojanin1 and dynamin [64, 207]. | Clathrin-dependent [65, 66, 207] and clathrin-independent, ultrafast endocytosis [209, 299]. Autophagy [212, 213]. | Risk locus rs10756899 [74]. |
Autophagy/mitophagy | Pink1 | A serine-threonine kinase -dependent mechanism elevates PI3,4,5P3 levels [300]. | Activation of AKT pathway; regulator of mitophagy [301]. | PARK6 G309D, W437X and additional utosomal recessive mutations [302, 303]. |
Autophagy/mitophagy | DJ1 | Involved in PI3K/AktPathway [304]. | A sensor for oxidative stress. Degradation of dysfunctional mitochondria via autophagy [305–307]. | PARK7 Deletion and point/missense (M26I, A104T, D149A, E163K, and L166P, L172Q) mutations [303, 308]. |
Retromer complex; autophagy | VPS35 | A component of the retromer complex, that consists of sorting nexins (SNX), containing PI3P/PI3,5P2 binding domain [230, 309]. | Involved in packaging and delivery of cargos from endosomal membranes to TGN (or PM) [246]. Regulator of LRRK2 kinase activity [181, 182]. | PARK17 Point mutation D620N. Disease-associated variants [227, 242]. |
Late endosome | Rab7 | Interacts with the PI4P 5-kinase, PIP5Kγ [241] and the PI3-kinase Vps34 [240]. Associates with the recruitment of PI4K2A kinase to endosomes [231]. | Involved in late endosome and autophagosome maturation and fusion with the lysosomes. Recruit the retromer complex to endosomal membrane [229, 249]. | No genetic link to PD is currently known. Phosphorylated by LRRK2 [223, 224]. |
Early endosome | Rab5 | RAB5-effector proteins including PIP kinases and phosphatases [236–238]. | Involved in maturation to early endosomes; phagophore closure [310]; and mitophagy [311]. | No genetic link to PD is currently known. Phosphorylated by LRRK2 [223, 224]. |
PIPs are phosphorylated derivatives of the acidic membrane phospholipid, phosphatidylinositol (PI). The seven PIP molecules differ in the position and number of phosphorylated hydroxyls on the inositol ring of this phospholipid. All PIP members are master regulators of cellular signaling pathways [3]. They are distributed between cell membranes in a defined and characteristic manner that is maintained locally by PIP-kinases and PIP-phosphatases, which generally present specificity toward the phosphate(s) position on the inositol ring. The composition of PIPs on cell membranes is also dependent on cell activities, vesicular trafficking, and non-vesicular lipid transport [4]. Accordingly, phosphatidylinositol 3 phosphate (PI3P) is enriched at early endosomes; phosphatidylinositol 4 phosphate (PI4P) at Golgi membranes, the plasma membrane and recycling endosomes; phosphatidylinositol 3,5 bisphosphate (PI3,5P2) at late endocytic compartments; phosphatidylinositol 4,5 bisphosphate (PI4,5P2) and phosphatidylinositol 3,4,5 triphosphate (PI3,4,5P3) are enriched at the plasma membrane [3–6].
The defined and characteristic PIP composition on subcellular membranes provides a lipid signature that directs proteins to specific organelles and facilitates cell activity. Proteins may interact with PIPs through direct binding, mediated by high-affinity PIP-binding domains or charge attractions, formed between the acidic PIPs and positively charged protein domains. PIP-binding proteins may recruit other proteins to the membrane through protein-protein interactions. In addition to directing proteins to specific organelles, the interaction with PIPs may allosterically activate proteins [3].
PI, the precursor of all PIPs, is synthesized at the endoplasmic reticulum. It is then delivered to cell membranes by non-vesicular lipid transport at membrane contact sites (MCSs) [6, 7] and by vesicular trafficking. PI is an abundant membrane phospholipid, representing ∼10–20 mol % among mammalian membranes. However, the total levels of PIPs appear to be maintained at very low levels, estimated at 2–5% of PI [4], suggesting that the regulation of PIP homeostasis may involve additional factors that are yet to be identified.
Table 1 lists PD-associated proteins that are linked with PIPs through one of the following categories: 1) PD-associated genes involved in homeostasis of PIPs, 2) PD-associated genes that directly interact with PIPs, or 3) PD-associated genes that interact with other PIP-binding proteins. The genetic evidence linking these genes with PD includes identified mutations with Mendelian PD inheritance or contributing risk factors identified in genome-wide association studies (GWAS). The genes listed in Table 1 are involved in clathrin-mediated endocytosis and/or macroautophagy, mechanisms consisting of vesicular membrane trafficking.
PIPs AND VESICULAR MEMBRANE TRAFFICKING
Cell membranes regulate vital mechanisms of cell biology and adequate responses to external and internal stimuli. The membranes undergo continuous adjustments of their contents, including phospholipids and fatty-acyl side chains, to enable structural and functional membrane plasticity [8]. The dynamic nature of cell membranes underlies vesicular membrane trafficking and facilitates cargo delivery, including proteins and other molecules wrapped up in double-membrane vesicular structures, between cell compartments or between the cell and its environment. Vesicular membrane trafficking is mediated through vesicles budding from a donor membrane and fusing with a destination membrane. It involves the recruitment of specific membrane-interacting proteins to support and stabilize defined stages in the process. Major vesicular trafficking routes include: 1) the secretary ER to Golgi pathway that delivers folded and post translationally modified proteins, as well as additional cargo molecules, through COPII and COPI-coated vesicles; 2) internalization of molecules from cell exterior into the cell interior by clathrin-coated or other membrane vesicles and then sorting by endosomes; 3) the internalized cargo can be delivered to the lysosomes for degradation or recycled back to the plasma membrane; 4) the retromer complex rescues proteins from lysosomal degradation by diverting them to the retrograde endosome-to-Golgi trafficking pathway or back to the plasma membrane through a recycling pathway [9]; and 5) macroautophagy engulfs a portion of the cellular contents in autophagosome for delivery to the lysosome for degradation [10]. Specific adaptations of vesicular membrane trafficking mechanisms enable specialized cell activities. For example, neuronal cells communicate with their environment through exocytosis of synaptic vesicles (SVs) and release of neurotransmitters. Exocytosis of SVs is coupled with mechanisms of endocytosis, including endocytosis mediated by the formation of clathrin-coated vesicles [11, 12].
PIPs play critical roles in vesicular membrane trafficking [3, 13]. The defined and characteristic composition of PIP on cell membranes enables the formation of a gradient of PIP-concentrations, along which the levels of specific PIPs in the origin membrane of the vesicle are altered to adjust with vesicle development and the composition of PIPs on the target membrane. The process of formation and development of membrane vesicles is accompanied by a coordinated recruitment of PIP-kinases and/or PIP-phosphatase to enable the required transitions in the composition of PIPs and to facilitate the fusion with the target membrane [5].
CLATHRIN-MEDIATED ENDOCYTOSIS (CME)
CME is the major endocytic pathway in mammalian cells (for recent reviews [14, 15]). This mechanism regulates the partitioning of transmembrane transporters and receptor proteins between the surface of the cell and the interior. In this way, CME regulates the availability of these proteins to receiving and internalizing signals and molecules from the external environment into the cell (Fig. 1). CME occurs through clathrin-coated pits (CCPs). A curve-forming membrane-structure coated with a clathrin lattice, which comprises three clathrin heavy chains (CHC), with tightly associated clathrin light chains (CLC) and the adaptor protein-2 (AP2), a heterotetramer consisting of α, β2, μ2, and σ2 subunits. CME can be dissected into four stages: (a) initiation, (b) stabilization, (c) maturation, and (d) membrane scission. The released clathrin-coated vesicle (CCVs) is then uncoated and delivers its cargo to early endosomes. Specific endocytic accessory proteins function to facilitate each stage and the transition between the stages. Importantly, this orchestrated transition between stages is critically regulated by PIPs [14–16]. Sequential recruitment of specific PIP-kinases and PIP-phosphatases generates the spatial and temporal combination of PIPs required to support the process. At initiation on the plasma membrane, PI4,5P2 is generated from PI4P by phosphatidylinositol 4-phosphate 5-kinase 1 (PIPKI). It acts to recruit the endocytic clathrin adaptors and their accessory factors to initiate the formation of a CCP [17, 18]. The maturation of the vesicle is accompanied by a gradual decline of PI4,5P2 and generation of PI3,4P2 in a two steps process where PI4,5P2 is first converted to PI4P by removal of the phosphate from the 5th hydroxyl position on the myo-inositol ring, a process mediated by synaptojanin1 (SJ1) [19] and SHIP2, inositol-5-phosphatase [20]; PI4P is then phosphorylated at the 3rd position of the inositol ring to generate PI3,4P2 by the class II phosphatidylinositol 3-kinase C2α (PIK3C2α) [21]. PI3,4P2 facilitates the recruitment of Bin/Amphiphysin/Rvs (BAR) domain-containing proteins, including sorting nexin (SNX)9/18 and vesicle scission by dynamin [5]. Vesicle uncoating occurs post scission in a process that requires dephosphorylation of PI4,5P2 to PI4P and synthesis of PI3P on the vesicle [22] to facilitate fusion with early endosomes [13]. A certain degree of inconsistency, attributed to differences in tracing molecules or other technologies, persists [5, 15]. The importance of dephosphorylating PI4,5P2 to PI4P for vesicle maturation was initially identified in CME of SVs in neuronal synapses and was shown to depend on the PI4,5P2-phosphatase, SJ1 [23]. Neurons of mice, in which the brain-specific SJ1 isoform was knocked out, accumulated coated SVs as well as PI4,5P2 [24]. In non-neuronal cells, the 5-phosphatase OCRL is implicated in PI4,5P2 dephosphorylation during endocytosis [25, 26]. OCRL is suggested to function in close cooperation with the 4-phosphatase, Sac2/INPP5F [27]. A cooperative function of OCRL/Sac2/INPP5F presents dual 5- and 4-phosphatase activities similar to the neuronal SJ1 [27].
Fig. 1
PD-proteins in clathrin-mediated endocytosis. Stages in clathrin-mediated endocytosis (CME) and the suggested involvement of PD-associated proteins at specific stages of the process. Created with BioRender.com.
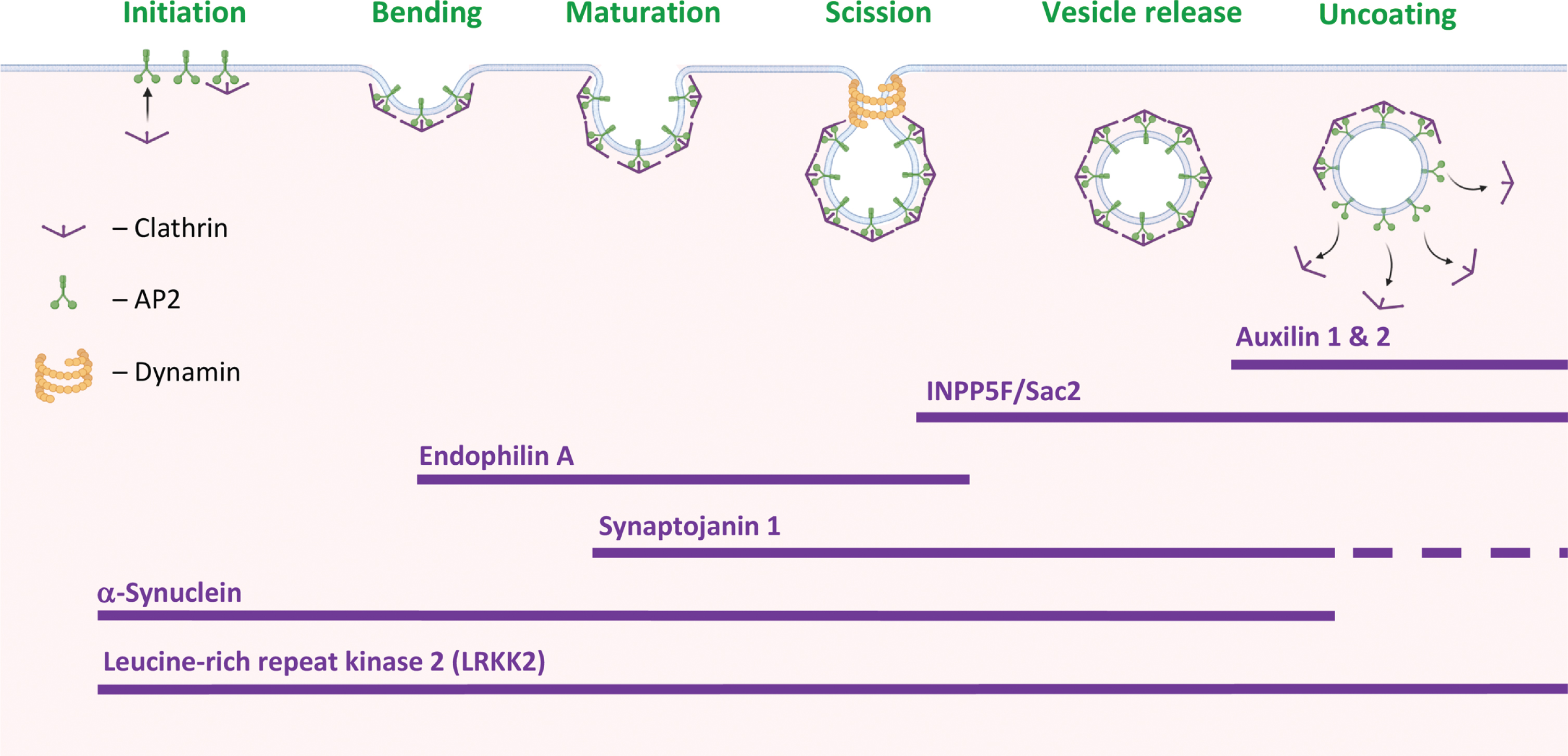
An involvement in CME has been attributed to several PD-associated proteins (Fig. 1). From the initiation and stabilization of the CCP to vesicle scission. That is, α-Synuclein (α-Syn) and Leucine-rich repeat kinase 2 (LRRK2) are involved throughout the process; Endophilin A, in CCP membrane bending and invagination; SJ1, in CCP maturation; INPP5F/Sac2 and SJ1, in vesicle scission and release; and auxilin 1 & 2, in vesicle uncoating.
AUTOPHAGY
Autophagy regulates the recycling of cellular components by degrading dysfunctional or damaged proteins and organelles. There are three forms of autophagy: macroautophagy, microautophagy, and chaperone-mediated autophagy (CMA) [10]. In macroautophagy, a membrane-based structure called phagophore, engulfs a portion of the cytoplasm and gradually develops into a mature autophagosome vesicle. The autophagosome membrane will then fuse with membranes of endosomes or lysosomes [28]. In microautophagy, an endosome or lysosome directly invaginates and engulfs its cargo. The invaginated membrane pinches off into the lumen of its originating organelle as a microautophagic body [29]. In CMA, the cargo is selectively recognized by a chaperone protein and then directly internalized into the lysosome for its degradation [30]. Microautophagy and CMA, depend on cognate protein of 70 kDa (Hsc70) for target degradation. In addition, CMA requires the lysosome-associated membrane protein 2 isoform A (Lamp2A) as protein receptor [30, 31]. Thus, macroautophagy operates through vesicular trafficking, however, microautophagy and CMA proceed with a direct engulfment of cargo into the endolysosomal compartment [32]. Evidence for increased abundance of autophagosomes in brains with PD [33, 34] and the abundance of lysosomes in the Lewy-bodies [35] directly connect the autophagy-lysosomal pathway with the disease. Although it is not clear yet whether the data indicate increased clearance of damaged proteins and organelles by autophagy or defective autophagic mechanisms.
PIPs are critically involved in virtually every step in the autophagy process (for a recent review see [36, 37]). It is now generally agreed that PI3P plays a pivotal role in the initiation of autophagy; PI4P and PI4,5P2 are required for the gradual growth of the phagophore and generation of a double- membraned autophagosome; and PI3,5P2 is important for the fusion of the autophagosome with the lysosome, to form an autolysosome. To achieve the fine dynamic balance in membrane PIP composition along the process, specific PIP-interacting proteins or PIP-modifying enzymes are sequentially recruited throughout the process [36, 38]. In mammalian cells, the key signal to suppress or initiate autophagy predominantly relies on the mammalian target of rapamycin complex 1 (mTORC1), which is regulated by PM levels of PI4,5P2. Conversion of PI4,5P2 to PI3,4,5P3 by PI3 kinase is leading to activation of mTORC1 and inhibition of autophagy. The reverse effect of PTEN 3-phosphatase, to dephosphorylate PIP3 and generate PI4,5P2, initiates the autophagy process.
PIPs also regulate autophagic lysosome reformation, an alternative pathway for lysosome generation during autophagy, in which a localized budding of autolysosome membranes forms tubules that undergo scission to generate new lysosomes [39, 40]. This process is facilitated by PI4P and PI4,5P2 that recruit protein effectors to the reformed membranes. The inositol polyphosphate 5-phosphatase, INPP5K, is required for this process [41]. Mutations in INPP5K are associated with marked lysosome depletion and inhibition of autophagy. Resulting from the reduced conversion of PI4,5P2 to PI4P on autolysosomes, and impaired autophagic lysosome reformation [42, 43]. In relevance to this review, INPP5K was identified as a contributing risk factor for PD [44, 45].
The critical relevance of autophagy in familial and sporadic PD was recently reinforced by the discovery of mutations in specific PD-associated genes and contributing risk factors encoding for proteins that are part of the autophagy-lysosomal and mitophagy pathways (recently reviewed [46–48] and Table 1). In addition, histopathological evidence has pointed at the accumulation and defective clearance of autophagosomes in PD brains [48]. Protein markers of autophagosomes and lysosomes were commonly detected within Lewy-pathology of PD brains, as well as the related synucleinopathies, multiple system atrophy and dementia with Lewy-bodies [35, 48]. Together, autophagy is implicated in PD at the genetics and histopathology of the disease.
PIPs, AUTOPHAGY AND CME IN PD
Autophagy and endocytosis are two distinct vesicular membrane trafficking mechanisms that intersect at several stages throughout vesicle formation, transport and fusion. These mechanisms share components of the molecular machinery and a common endpoint at the lysosome (reviewed in [31, 49]). Growing evidence suggests that autophagy is dependent on endocytosis and vice versa [31]. Many of the PD- and PIP-associated genes are involved either in CME or autophagy, of which several genes including, α-Syn, SJ1, endophilin A, LRRK2 and members of the Rabs family, play a role in both mechanisms (Fig. 2).
Fig. 2
PD- and PIP-associated proteins in vesicular trafficking. The distribution of PD- and PIP-associated proteins in cellular mechanisms of vesicular membrane trafficking, and the relevant PIPs. Proteins involved in both mechanisms, macroautophagy and CME (α-Syn, LRRK2, SJ1 and endophilin A); mitophagy (PINK1 and DJ1); and endo-lysosomal proteins (ATP13A2, VAC14, VPS35, Rab5 and Rab7). Created with BioRender.com.
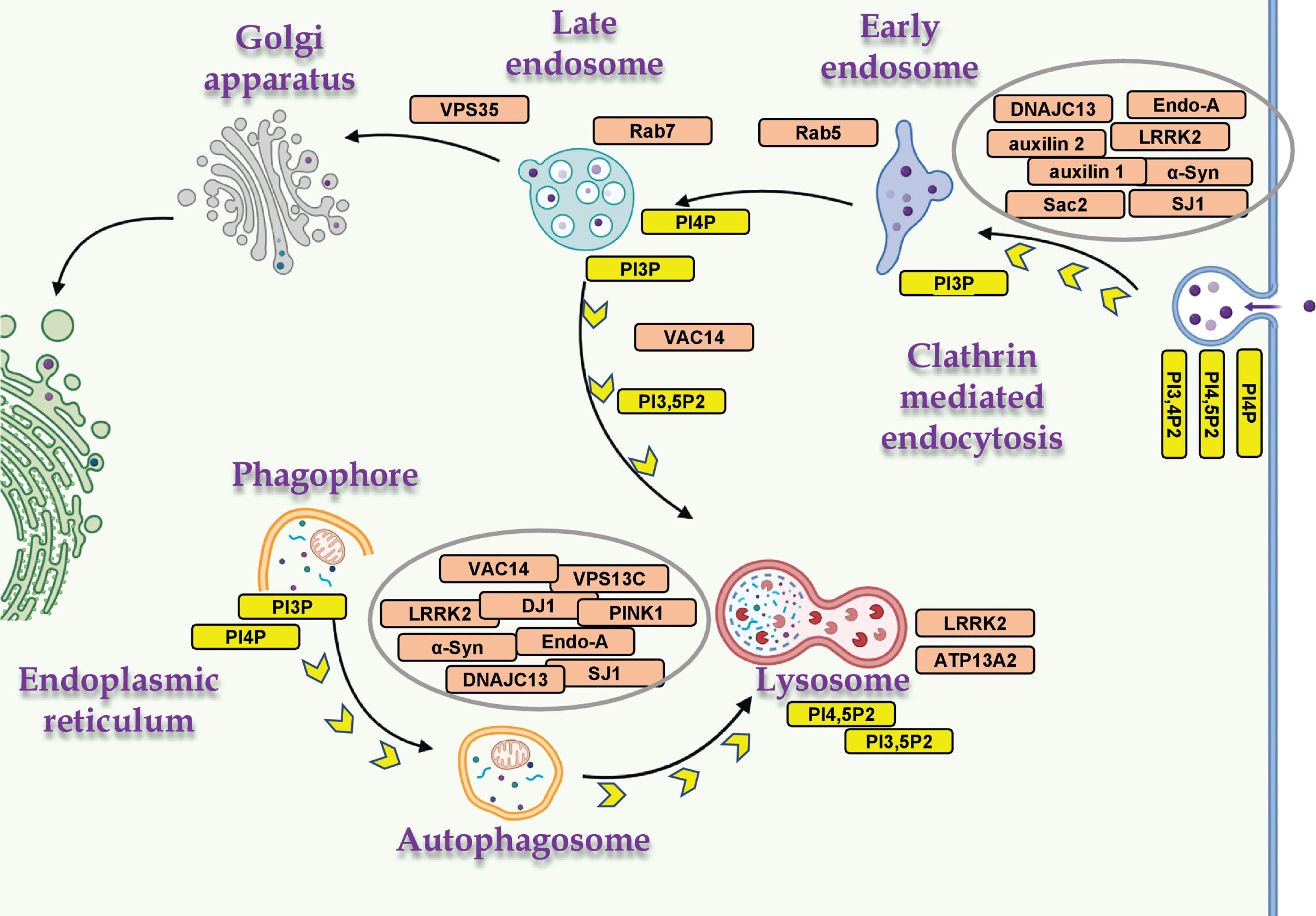
SJ1
SJ1 and PD
SJ1 harbors two PIP-phosphatases. A 5-phosphatase that dephosphorylates PI4,5P2 and also PI3,4,5P3 [23, 50], and a Sac1 PI 4-phosphatase that dephosphorylates PI4P to PI and can also function as a PI-3 phosphatase [23, 51]. Mutations in the Sac domain (R258Q, R459P) or the 5-phosphatase domain (Y832C, R839C) of SJ1 have been associated with early-onset Parkinsonism and typical PD, respectively [52–55], and defined SJ1 as PARK20 [54, 56–60]. Data obtained from genome-wide expression studies reported down-regulation of SJ1 transcript in brains affected with sporadic PD [53]. Knock-in mice, carrying the corresponding human R258Q mutation in the Sac1 domain of the mouse SJ1 [52], showed motor defects and epilepsy. In relevance to PD, the mice demonstrated structural alterations in dopaminergic nerve terminals in their dorsal striatum [52]. In addition, a reduced rate of SVs endocytosis accompanied by abnormal accumulations of clathrin-coated intermediates, was reported. Based on these findings, a role for the Sac domain of SJ1 in vesicle uncoating was suggested. The R258Q mutation in SJ1 was shown to abolish the phosphatase activity of the Sac domain without affecting the 5-phosphatase activity [54]. This finding supports an independent activity for each of SJ1 phosphatases in CME. Together, the PD-associated mutations in SJ1 link CME and disease mechanisms [52]. An additional mouse model, carrying a heterozygous deletion of SJ1 (SJ1+/-) displayed an age-dependent motor dysfunction, impaired SV endocytosis and degeneration of dopaminergic terminals [53].
SJ1, PIP and CME
A well-defined function of SJ1 in the synapse, is to couple SV endocytosis with dephosphorylation of PI4,5P2, by sequentially removing the phosphates from the 5th and 4th positions of the inositol ring via its two phosphatase domains [13, 23, 51, 61]. Two splice variants for SJ1 are known, a 145- and a 170-kDa isoforms [23]. Although both isoforms are ubiquitously expressed, SJ1-145 is present at very high concentrations in presynaptic nerve terminals of the adult brain [62]. The SJ1-170 splice variant contains binding sites for clathrin and the clathrin-adaptor AP2 and is present throughout the formation and maturation of CCPs [19]. Whereas, the SJ1-145 variant is involved in the dephosphorylation of PI4,5P2 as part of vesicle scission and uncoating [19].
Recruitment of SJ1 to endocytic sites is mediated by the PD-associated endocytic protein, endophilin [63, 64]. Genetic deletion of either SJ1 or endophilin in mouse neurons leads to defects in synaptic transmission [24, 65, 66], represented by a lower abundance of SVs in nerve terminals; delayed SV endocytic recycling and accumulation of coated vesicles that have not gone through the uncoating step of CME.
SJ1 and autophagy
While SJ1 has long been known for its involvement in SV cycling, recent data support a role for SJ1 in autophagy in the presynaptic terminals [67, 68]. A study in a knock-in Drosophila model, expressing the equivalent to the human R258Q mutation in the endogenous SJ1 Sac domain (R228Q), reported interference with the 3-phosphatase activity [67] and accumulation of synaptic autophagosomes. The accumulated autophagosomes were positive to WIPI2/Atg18 protein, which is a PI3P/PI3,5P2-binding protein, suggesting abnormal PIP homeostasis at nerve terminals. In addition, abnormal maturation of autophagosomes was detected in patient-derived induced neurons, carrying the human SJ1 R258Q mutation [67]. Impaired autophagy was also reported in mice carrying a heterozygous deletion of SJ1 (SJ1+/-) [53]. These results suggest that the PIP-phosphatase activities of SJ1 couple autophagy with vesicle cycling and synaptic activity.
Of relevance, a second PIP-phosphatase, Sac2/INPP5F, containing a Sac domain that functions primarily as a PI4P phosphatase [27, 69], was located within a PD risk locus identified by GWAS [70]. Sac2/INPP5F has been localized to vesicles formed by CME, as well as other endocytic membranes, including macropinosomes, and Rab5 endosomes [27, 69]. A synergistic role for Sac2/INPP5F and SJ1 in regulating PI4P pool size, whose dysfunction results in PD, was suggested [27, 71].
α-Syn
α-Syn and PD
α-Syn is strongly implicated in the genetics of PD. GWAS data showed that the SNCA gene, encoding for α-Syn protein, makes the largest contribution to the genetic risk associated with idiopathic PD [72–74]. Genetic variability within the SNCA promoter that results in higher expression levels of α-Syn is associated with idiopathic PD [75]; Higher α-Syn expression levels, resulting from gene duplications or triplications of the chromosomal loci encoding SNCA, are a dominant-inherited cause of PD [76–78]. Moreover, six autosomal dominant mutations in SNCA have been described, namely, A53T [79] A30P [80], E46K [81], H50Q [82, 83], G51D [84, 85] and A53E [86, 87].
α-Syn is also implicated in the histopathology of PD. α-Syn is a major constituent of Lewy-pathology [88], the hallmark pathology of PD, including Lewy-bodies and Lewy-neurites [89]. It is currently debated whether in the Lewy-bodies α-Syn occurs primarily in fibrillated or non-fibrillated forms [35, 90]. A debate with a significant impact on the way we understand the pathogenesis of α-Syn in PD and the related synucleinopathies [91].
α-Syn, membrane phospholipids and PIP
Early studies have shown that α-Syn binds membrane lipids [92] with a preference for negatively charged phospholipids [92–94]. A lipid-binding domain in α-Syn protein, consisting of a stretch of ∼100 aa residues at its N-terminus [94], harbors seven imperfect repeats of a KTKEGV motif [95]. Positively charged Lysine residues within the repeat motif were shown to be essential for lipid binding, mediated by charge attraction with the negatively charged phospholipid head groups [96, 97]. Membrane binding enhances structure acquisition for this intrinsically disordered protein [92, 98, 99]. The membrane binding domain forms an extended curved α-helical structure [100] that promotes binding to curved membranes, akin vesicular structures [101]. A hydrophobic core region within the membrane binding domain is suggested to penetrate the phospholipid bilayer beyond the plain of the phospholipids head-group, where it interacts with their fatty acyl chains [102–104]. It has been suggested that the membrane binding domain in α-Syn senses membrane curvature; facilitates the formation or stabilizes a curvature on membrane structures [105]; or forms a tether between vesicles [101]. The acidic C-terminal region in α-Syn has been suggested to support and sterically stabilize curved membrane structures [106]. In accord with these reports, a role for α-Syn in the maintenance [107, 108] and dynamics of SV pools has been reported [109, 110].
Additional sets of data support an involvement of α-Syn in regulating the content of lipids on cell membranes. Previous studies have shown that α-Syn binds fatty acids; enhances their uptake into cell lines and mouse brains; and incorporation into phospholipids [111, 112]. A preference for uptake and metabolism of polyunsaturated fatty acids (PUFA) was suggested [111, 112]. A recent study reported increases in levels and metabolism of Oleic acid, a mono-unsaturated fatty-acid, upon overexpression of α-Syn in yeast cells, mouse neurons and human induced pluripotent stem cell (iPSC)- neurons [113]. Lipidomic data in yeast cells further showed alterations in the profiles of phospholipids, triglycerides and cholesterol with α-Syn expression [113]. α-Syn effects on membranes’ phospholipid content were also demonstrated in myelin membranes purified from α-Syn tg mouse brains. Higher levels of phospholipids, detected by 31P NMR spectra, were reported in young and healthy α-Syn tg mice [114]. In accord with the increases in the content of lipids, accumulation of lipid droplets was detected with α-Syn expression [113, 115, 116]. Importantly, the alterations in lipid content observed in the disease models were shown to associate with α-Syn toxicity [113, 117–121]. Together, the data support a general role for α-Syn in lipid homeostasis and metabolism.
In relevance to disease mechanisms, alterations in lipid profiles were reported in brains affected with PD and multiple system atrophy at autopsy (reviewed in [97, 122, 123]. Importantly, genetic studies pointed at several genes that are involved in lipid metabolism and implicated in the genetics of PD, including biosynthesis and turnover of phospholipids, glycolipids, sphingolipids and fatty acids [97].
Based on two main observations relevant to α-Syn associations with lipids, its preference for binding acidic phospholipids and its effects to increase the content of membrane phospholipids, it was hypothesized that α-Syn associates with PIPs. Indeed, it was found that α-Syn specifically interacts with PIPs [124–128]. The data further suggested that α-Syn expression increased the steady-state levels of PI4P, PI3,4P2 and PI4,5P2 [128]. Expression of the PD-associated mutations in α-Syn, A53T and A30P, further increased membrane levels of these PIPs [128]. A mutant form of α-Syn, which denies lipid binding, due to charge replacement in the KTKEGV repeat motif, was ineffective in this respect [96, 125, 128], suggesting that this motif is critical for α-Syn regulation of PIPs. Of interest, in relevance to α-Syn effect to enhance uptake and incorporation of PUFAs [111, 112], PIPs are normally enriched with polyunsaturated fatty acyl chains [4].
The mechanism through which α-Syn acts to regulate the overall content of membrane lipid is currently unknown. Such a mechanism may potentially involve alterations in the expression levels of genes that are master regulators of lipid synthesis and homeostasis [129, 130]. A potential explanation may involve α-Syn translocation to the nucleus and its involvement in transcription activation mediated by nuclear receptors [95, 131], including regulators of lipid homeostasis [132, 133]. In relevance to this review article, although no mechanistic explanation currently supports α-Syn involvement in the regulation of PIPs, the available data place α-Syn among PD- and PIP-associating protein (Table 1).
α-Syn, CME and SV cycling
A role for α-Syn in CME was first suggested based on the determination of transferrin’s internalization, a CME prototype, in cultured dopaminergic cells [134]. α-Syn-mediated enrichment of cell membranes with PUFAs enhanced membrane fluidity [112] and further enhanced endocytosis of transferrin [134]. Accordingly, enrichment of membrane phospholipids with PUFA facilitated membrane invagination and scission of membrane vesicles mediated by endophilin and dynamin proteins [135]. The reported enrichment of α-Syn expression at presynaptic terminals [95, 136] and the knowledge that CME is a major route for SVs endocytosis, supported a potential role for α-Syn in SVs cycling. Indeed, loading the synapses with the lipophilic FM1-43 dye following evoked synaptic activity indicated lower uptake in cultured hippocampal neurons from α-Syn-/- than in wild type mouse neurons [134]. Restoration of α-Syn expression in primary cortical neurons from α-Syn-/- mouse brain enhanced endocytosis determined by the pH-sensitive fluorescence of Synaptophysin-pHluorin (SypHy) chimeric indicator [128]. It was further suggested based on α, β and γ-Syn knock-out in mice that all three synucleins are involved in clathrin-mediated SV recycling at presynaptic nerve terminals [137].
Growing evidence now supports a role for α-Syn to facilitate and increase the rate of endocytosis [128, 134, 137–139]. α-Syn colocalizes with clathrin [134, 137]. Its colocalization with phosphorylated AP2, PI4,5P2 and PI3,4P2 [128] supports its recruitment to CME already at the initiation stage. Data obtained in synapses of dynamin 1, 3 KO neurons, in which CME is arrested at the scission step and synapses accumulate clathrin pits [140, 141], show that α-Syn is colocalizing with clathrin in the arrested clathrin pits [137]. Moreover, α-Syn was shown to directly interact with Hsc70 and excess of α-Syn at the lamprey reticulospinal synapse leads to sequestration of Hsc70 and impairment of CCV uncoating at the synapse [142]. Together, these findings support an association of α-Syn with CCPs from vesicle initiation, throughout their growth and maturation, to scission and uncoating. However, other studies reported that excess of α-Syn in the synapse interfered with endocytosis [124, 142, 143]. Moreover, loading synapses of the lamprey neurons with α-Syn resulted in inhibition of SV endocytosis during intense electrical stimulation [144]; and neurons overexpressing α-Syn were shown to internalize lower amounts of styryl dye indicators for SV recycling, indicating a reduction in endocytosis [109, 145].
Alongside the reports describing a role for α-Syn in CME and SV endocytosis, a large body of evidence also supports a role for α-Syn in SV exocytosis (recently reviewed in [146, 147]). α-Syn was shown to play roles in the assembly of the SNARE complex [148–151]; vesicle docking and fusion [152, 153]; transmitter release [110, 154, 155]; and regulation of fusion pore dilation [156]. To summarize a large body of evidence that links α-Syn with vesicular membrane trafficking, it is currently difficult to conclude whether α-Syn enhances or inhibits the process, and whether it is endocytosis or exocytosis [146, 147].
A recent study has shown that the actual composition of PIPs in the plasma membrane determines α-Syn’s activity in the endocytosis of transferrin. A rapid recruitment of PIP 5-phosphatase to the plasma membrane [157], acutely depleted PI4,5P2 content and in accord, inhibited endocytosis of transferrin. The data further supported a role for membrane PIPs in SV endocytosis mediated by α-Syn [128]. Importantly, in addition to its roles in SVs endocytosis, PI4,5P2 is critically involved in SVs exocytosis. Including, priming and fusion steps of Ca2 + -triggered vesicle release [158]; recruitment and activation of specific protein regulators of SNARE complex assembly and function [158, 159]; and involvement in dilation of the fusion pore [158, 159]. PI4,5P2 roles in exocytosis may also involve PI4,5P2-binding proteins such as CAPS, Munc13 and synaptotagmin [160–163] or its effects on F-actin polymerization [164].
Considering the findings showing that α-Syn involvement in SVs cycling is mediated through its effects to enrich cell membranes with PIPs, it is reasonable to hypothesize that similar to its effects in endocytosis, α-Syn may also act in exocytosis by enriching the presynaptic membranes with PIPs.
It is thus important to better understand the involvement of α-Syn in the regulation of synaptic PI4,5P2 levels. Whether there are single or multiple pools of PI4,5P2 at the synapse and whether regulatory checkpoints may act to maintain a balance in PIP availability for the endocytic and exocytic arms of SV cycling.
α-Syn and autophagy
The associations of α-Syn with autophagy are complex. Toxic α-Syn forms, including misfolded or aggregated α-Syn, are cleared by CMA [165, 166] and macroautophagy [167]. In relevance to the clearance of α-Syn, data suggest the occurrence of a cellular cross-talk between macroautophagy and CMA. A compromise in CMA results in activation of macroautophagy and vice versa [30]. Additional data support an involvement of α-Syn in macroautophagy, yet with a certain degree of controversy. That is, over expression of α-Syn was shown to enhance macroautophagy [165, 168, 169]. However, other studies have reported evidence showing an inhibitory role for α-Syn in autophagosome formation and macroautophagy [170, 171].
In summary, the controversies over α-Syn effects in autophagy and CME, may relate to one another. The shared components between the two pathways, including the dependence on vesicular membrane trafficking and the critical roles of PIPs throughout the processes, bring up a question related to the mechanism of action of α-Syn in autophagy. Whether similar to its role in CME, α-Syn may act in autophagy to alter the steady state levels of specific membrane PIPs?
LRRK2
LRRK2 and PD
LRRK2 is a large multidomain protein harboring a kinase, GTPase and protein-binding domains [172]. Missense mutations in LRRK2 cause late-onset autosomal dominant PD which is clinically indistinguishable from sporadic PD [173, 174]. LRRK2 mutations are the most common genetic cause of familial PD [173, 175] and GWAS studies identified LRRK2 as a risk factor for idiopathic PD [70]. Pathologically, LRRK2 mutation carriers experience progressive neurodegeneration of the nigrostriatal pathway and often develop α-Syn-positive Lewy-bodies. In addition, a subset of patients with LRRK2 mutations presents inclusion bodies that are negative for α-Syn yet, positive for tau and TDP-43 [173, 176–178]. Suggesting that the pathophysiology of LRRK2 in PD may occur independently of classical Lewy-pathology. Through its kinase activity, LRRK2 interacts with many PD-associated proteins. In addition, Rab29 [179–181] and VPS35 [181, 182] proteins associated with PD, are upstream regulators of LRRK2. Due to its central roles in the genetics and pathophysiology of PD, LRRK2 is a subject of intensive research. The readers are referred to other recent reviews that provide a comprehensive description of the current knowledge (for example [172, 183]).
LRRK2 and PIP
While no evidence is currently known to directly link LRRK2 and PIPs, ample evidence shows that LRRK2 interacts with and phosphorylates PD-associated, PIP-interacting proteins. These include the following proteins that are involved in vesicular membrane trafficking: ATP13A2; Auxilin 1, and 2; Dynamin; endophilin-A1; SJ1; VPS35; and specific Rab proteins (see Table 1 and below).
LRRK2 and CME
LRRK2 plays a role in CME and SV endocytosis. Silencing LRRK2 expression in flies or mice resulted in accumulation of endocytic intermediates; an abnormal vesicle morphology; and a reduced number of synaptic vesicles [184, 185]. Notably, the defects in LRRK2 activity in endocytosis were attributed to its kinase activity [185]. The most common LRRK2 G2019S PD-mutation was shown to impair SV endocytosis in mouse ventral midbrain neurons, including in dopaminergic neurons [186]. It was further shown that the impairments in endocytosis, resulting from LRRK2 G2019S transgenic expression, could be rescued by inhibiting LRRK2 kinase activity [186, 187]. Reductions in SV densities were also reported for iPSC-derived dopaminergic neurons expressing the LRRK2 R1441C mutation [188]. Moreover, the vesicles appeared to lack distinct surrounding membranes, consistent with membrane-less clathrin cages observed in models involving defective clathrin uncoating [188].
LRRK2 was shown to interact physically and functionally with critical components or facilitators of CME and SV endocytosis, including auxilin [188], Dynamin 1, 2, and 3 [189], SJ1 [186, 190] and endophilin-A1 [184, 185]. LRRK2 also interacts with clathrin [191] and the clathrin adaptor AP2 complex, regulating the recruitment of AP2 [187] and phosphorylation of AP2M1 at threonine 156 [192]. Finally, data show evidence for indirect associations between α-Syn and LRRK2 [177]. Together, the reported data suggest that, through its phosphorylation and other associations with proteins involved in CME, LRRK2 is a master regulator of CME, recruited to the process at the early stage of initiation and supports the process throughout, to vesicle uncoating (Fig. 1).
LRRK2 and autophagy
An involvement for LRRK2 in autophagy is now well documented (reviewed in [172, 193]). However, inconsistencies in autophagy outcome, whether increasing or decreasing the autophagic flux, persists [172, 183]. The inconsistencies are explained by differences in experimental models and deny a conclusion about the mechanism of action of LRRK2 in autophagy.
Similar to α-Syn, LRRK2 is a substrate for CMA [165, 194]. The PD-associated G2019S mutation in LRRK2 and high levels of wild type LRRK2 were shown to inhibit CMA [194–197]. CMA inhibition by LRRK2 interfered with α-Syn clearance [194, 198], resulting in intracellular accumulation of α-Syn. The G2019S mutation increases LRRK2 kinase activity [199–201]. Interestingly, inhibitors for LRRK2 kinase activity were shown to reduce the accumulation of α-Syn in human neuronal cell lines carrying the G2019S mutation [202]. Supporting a pathogenic role for LRRK2 that involves α-Syn toxicity in PD.
LRRK2 was found to form a complex with Rab7L1 and auxilin 2 proteins, encoded by genes identified as risk genes for sporadic PD, and BCL2-associated athanogene 5 (Bag5). This complex promotes clearance of Golgi-derived vesicles through the autophagy–lysosome system [203]. Together, although the mechanism of action for LRRK2 in autophagy, CMA and the lysosome is still missing, it appears to be strongly associated with disease mechanisms.
Endophilin-A1
SH3GL2 encoding endophilin-A1 was identified in a PD risk locus by a large-scale GWAS meta-analysis [204]. Additional evidence linking endophilin with PD includes its phosphorylation by LRRK2 [185]; association with Parkin [205] and with the synucleins [137]; and its upregulation in PD brains [206]. Endophilin’s associations with PIPs are indirect and mediated through its interactions with the PIP-phosphatase, SJ1 [64, 207] and the PIP-binding protein, dynamin [64].
Endophilin CME
Endophilin is best known for its role in CME [64, 208]. However, it is also implicated in clathrin independent, ultrafast SV endocytosis [209], and exocytosis of neurosecretory vesicles [210]. Its interactions with the endocytic proteins, dynamin and SJ1 [49], facilitate neck formation in the endocytic vesicle [209] and clathrin-uncoating [65, 211]. Impairment of endophilin expression or function results in severe deficits in SV endocytosis [65, 66, 207, 211].
Endophilin and autophagy
Together with SJ1, endophilin belongs to the growing family of presynaptic endocytic proteins that are involved in autophagy (reviewed in [49]). Endophilin-A1 colocalizes with autophagosomes and its loss of expression interfered with the formation or transport of autophagosomes [212, 213]. An operational switch in endophilin’s function upon its phosphorylation by LRRK2 is suggested to differentiate non-phosphorylated endophilin activity in CME and membrane tubulation, while phosphorylated endophilin facilitates the formation of highly curved membranes, serving as docking sites for the autophagic factor Atg3 [212]. Thus, phosphorylation of endophilin is suggested to balance synaptic activity between endocytosis and autophagy.
Rabs
Rab GTPases include ∼70 family members in humans. They control all aspects of intracellular vesicle trafficking by acting as regulatable switches that recruit effector molecules [214, 215]. Rab GTPases are reversibly anchored into membranes by hydrophobic geranylgeranyl groups. Rabs cycle between two states, an active GTP-bound and an inactive GDP-bound state. The conversion between active and inactive states is catalyzed by guanine nucleotide exchange factors (GEFs) and GTPase-activating proteins (GAPs) [216].
Rabs and PD
Mutations in Rab39b (Park 21 locus) predispose to PD in humans [217, 218]. Additional genetic findings link Rab7L1, a LRRK2 effector protein also known as Rab29 [179, 180], with PD [219]. Overexpression of Rab1, Rab3a, Rab8a and Rab11 attenuate α-Syn-induced cytotoxicity in cell and animal models [220–222]. The potential involvement of additional Rabs is supported by data showing that LRRK2 interacts with and phosphorylates several Rab proteins, including Rab5 and Rab7 [172, 180, 223–226]. The phosphorylation of Rab proteins is augmented by PD-associated mutations in LRRK2, which interfere with Rabs’ ability to bind both upstream regulatory proteins and downstream effector proteins [223, 224]. In addition, Vps35, a PD-associated gene [227] that is part of the retromer complex, interacts with Rab7 [228] to recruit the retromer complex to the endosomal membrane [229, 230].
Rab5, Rab7, endo-lysosomes and PIPs
The conversion between the Rab-GTP and Rab-GDP bound states, regulated by GEFs and GAPs, is mediated by the content of specific PIPs on the membrane [231, 232]. Rabs associate with PIPs, yet, these associations are generally indirect and mediated by other proteins (Table 1). Rabs regulate the recruitment and activation of PIP kinases and phosphatases [233]. For example, Rab35 binds and recruits OCRL, a PI4,5P2 5-phosphatase, to facilitate PI4,5P2 hydrolysis and clathrin-uncoating in CME, and the subsequent steps of cargo sorting through endosomes [234, 235]. A dynamic conversion of PIPs occurs following vesicle uncoating, where PI4-phosphates (e.g., PI4P and PI4,5P2 [22]) are converted to PI3P alongside with recruitment of Rab5 to the vesicle as it matures into Rab5-positive early endosome [236]. At early endosomes, Rab5 interacts with the PI 3-kinases Vps34, PI3Kβ and PI3KC2γ to synthesize PI3P [236, 237]. Rab5 also interacts with the PIP-phosphatases, type II inositol 5-phosphatase (INPP5B) [238] and Sac2/INPP5F inositol 4-phosphatase [27].
A shift in PIPs composition couples the transition between early to late endosomes and the replacement of Rab5 with Rab7 [214, 239]. Similar to Rab5, Rab7 interacts with Vps34 [240]. However, Rab7-positive endosomes are associated with higher PI4P levels, primarily produced by PI4K2A kinase [231]. Rab7 interacts with the PI4P 5-kinase, PIP5Kγ [241] and the acute conversion of endosomal PI4P to PI4,5P2 causes Rab7 to dissociate from late endosomes [231]. Recent studies suggest the occurrence of a late endosomal regulatory loop that impacts autophagosome flux and involves Rab7 cycling and PI4P to PI4,5P2 conversion [231, 241]. That is, interference with PI4,5P2 synthesis on the late endosomal membrane resulted in accumulation of LC3-positive structures with defective autophagosome-lysosome fusion.
The retromer
The retromer is a peripheral membrane protein complex that plays an instrumental role in protein recycling from endosomes to the trans-Golgi network. The retromer consists of two distinct sub-complexes, a membrane recognizing, PI3P binding sorting nexin (SNX) complex and a cargo recognition, vacuolar protein sorting (Vps) complex. Importantly, a mutation in the VPS35 gene encoding a retromer core protein, is a rare cause of familial PD [227, 242]. An involvement of the retromer and the specific D620N mutation in VPS35 has been implicated in dysfunction of the dopaminergic synapse [243] and neurodegeneration [244–248]. The D620N mutation in VPS35 was also shown to enhance LRRK2-mediated phosphorylation of several Rab members [182]. Finally, Rab7 interacts with VPS35 and recruits the retromer to late endosomes [229], in a mechanism that also requires Rab5 [249].
A role for the retromer in SV recycling, autophagy and lysosomal degradation has been reported [250, 251]. The D620N mutation in VPS35 disrupted cargo sorting [252]; associated with an abnormal endosome morphology [253]; interfered with endosomal subcellular localization and displayed evidence of retention of early and late endosome markers [252]. Moreover, the D620N mutation poorly associates with SNX3 that binds PI3P on endosomal membranes and impairs the recruitment of the WASH complex to endosomes. However, the effects of D620N mutations in autophagy are not clear yet [253, 254].
PIPs AND NON-VESICULAR TRANSPORT
Cells rely on lipid transport mechanisms to maintain structural and functional membrane plasticity, and replenish PIPs levels at the plasma membrane. Vesicular membrane trafficking is an essential route for lipid transfer between cell membranes. Following transport, PIPs and their precursor PI may serve as substrates for enzymatic conversions that will generate the required local PIP composition for membrane activities.
A key mechanism that drives local delivery of lipids from the endoplasmic reticulum (ER), the site of PI synthesis, to the plasma membrane as well as other organellar membranes, is non-vesicular lipid transport [255]. A mechanism mediated by MCS, formed between two distinct organellar membrane compartments [256, 257]. MCS are stabilized by tethering complexes that maintain close proximity between the connecting membranes but without membrane fusion. This direct route of communication is regulating lipid exchange between membranes [255]. PIPs, including PI4P and PI4,5P2, are playing fundamental roles in non-vesicular lipid transport by serving for the exchange of lipids between the plasma membrane and the ER, mediated by lipid transfer proteins [3]. A mechanistic cooperation between vesicular trafficking and non-vesicular lipid transport has recently been implicated in phagolysosome resolution, a late stage in the phagolysosomal process that facilitates the absorbance of phagolysosomal contents. Transfer of PI4P from the phagolysosomes to the ER through membrane contact sites plays a significant role in this process [258]. Moreover, de novo phospholipid synthesis at the ER was shown to support the expansion of the growing phagophore vesicle by generating stable contacts between the membranes [259]. Of interest, the PD-associated VPS13C gene encodes for a lipid transporter, acting at MCSs [242, 260, 261]. In addition, α-Syn was detected MCSs formed between the ER and mitochondria [262].
β-GLUCOCEREBROSIDASE (GBA) AND PIPs
Although not directly related to the focus of this review, it is of interest to include a recent study that reported evidence for a role for PIPs in the cell trafficking of GBA, a sphingolipids hydrolyze [263]. Homozygous GBA mutations are a genetic cause of Gaucher disease. However, heterozygous carriers of GBA mutations have significantly increased risk for PD [264, 265]. Data show that the catalytic activity of the PI 4-kinases, PI4KIIIβ and PI4KIIα are required for the physiological trafficking of GBA along the route of its synthesis and maturation to lysosomes, where it is involved in sphingolipids catabolism.
CONCLUDING REMARKS
Understanding the implications of altered PIP homeostasis in PD mechanisms may provide clues to solve open questions and controversies over the function and dysfunction of several PD genes, improve disease diagnosis and management. Attempts are currently being made to develop specific chemical modulators for specific PIP-modifying enzymes as therapeutic strategies for diseases, including, infectious diseases, cancer and neurodegenerations. These developments hold promise for therapeutic approaches that seek the restoration of a physiologic PIP homeostasis.
ACKNOWLEDGMENTS
This work was funded by the Israel Science Foundation 182/12.
CONFLICT OF INTEREST
The authors have no conflict of interest to report.
REFERENCES
[1] | Obeso JA , Stamelou M , Goetz CG , Poewe W , Lang AE , Weintraub D , Burn D , Halliday GM , Bezard E , Przedborski S , Lehericy S , Brooks DJ , Rothwell JC , Hallett M , DeLong MR , Marras C , Tanner CM , Ross GW , Langston JW , Klein C , Bonifati V , Jankovic J , Lozano AM , Deuschl G , Bergman H , Tolosa E , Rodriguez-Violante M , Fahn S , Postuma RB , Berg D , Marek K , Standaert DG , Surmeier DJ , Olanow CW , Kordower JH , Calabresi P , Schapira AHV , Stoessl AJ ((2017) ) Past, present, and future of Parkinson’s disease: A special essay on the 200th Anniversary of the Shaking Palsy. Mov Disord 32: , 1264–1310. |
[2] | Abeliovich A , Gitler AD ((2016) ) Defects in trafficking bridge Parkinson’s disease pathology and genetics. Nature 539: , 207–216. |
[3] | Hammond GRV , Burke JE ((2020) ) Novel roles of phosphoinositides in signaling, lipid transport, and disease. Curr Opin Cell Biol 63: , 57–67. |
[4] | Balla T ((2013) ) Phosphoinositides: Tiny lipids with giant impact on cell regulation. Physiol Rev 93: , 1019–1137. |
[5] | Wang H , Lo WT , Haucke V ((2019) ) Phosphoinositide switches in endocytosis and in the endolysosomal system. Curr Opin Cell Biol 59: , 50–57. |
[6] | Dickson EJ , Hille B ((2019) ) Understanding phosphoinositides: Rare, dynamic, and essential membrane phospholipids. Biochem J 476: , 1–23. |
[7] | Balla T , Kim YJ , Alvarez-Prats A , Pemberton J ((2019) ) Lipid dynamics at contact sites between the endoplasmic reticulum and other organelles. Annu Rev Cell Dev Biol 35: , 85–109. |
[8] | Barelli H , Antonny B ((2016) ) Lipid unsaturation and organelle dynamics. Curr Opin Cell Biol 41: , 25–32. |
[9] | Yarwood R , Hellicar J , Woodman PG , Lowe M ((2020) ) Membrane trafficking in health and disease. Dis Model Mech 13: , dmm043448. |
[10] | Scrivo A , Bourdenx M , Pampliega O , Cuervo AM ((2018) ) Selective autophagy as a potential therapeutic target for neurodegenerative disorders. Lancet Neurol 17: , 802–815. |
[11] | Gan Q , Watanabe S ((2018) ) Synaptic vesicle endocytosis in different model systems. Front Cell Neurosci 12: , 171. |
[12] | Milosevic I ((2018) ) Revisiting the role of clathrin-mediated endoytosis in synaptic vesicle recycling. Front Cell Neurosci 12: , 27. |
[13] | Di Paolo G , De Camilli P ((2006) ) Phosphoinositides in cell regulation and membrane dynamics. Nature 443: , 651–657. |
[14] | Kaksonen M , Roux A ((2018) ) Mechanisms of clathrin-mediated endocytosis. Nat Rev Mol Cell Biol 19: , 313–326. |
[15] | Mettlen M , Chen PH , Srinivasan S , Danuser G , Schmid SL ((2018) ) Regulation of clathrin-mediated endocytosis. Annu Rev Biochem 87: , 871–896. |
[16] | Haucke V , Kozlov MM ((2018) ) Membrane remodeling in clathrin-mediated endocytosis. J Cell Sci 131: , jcs216812. |
[17] | Antonescu CN , Aguet F , Danuser G , Schmid SL ((2011) ) Phosphatidylinositol-(4,5)-bisphosphate regulates clathrin-coated pit initiation, stabilization, and size. Mol Biol Cell 22: , 2588–2600. |
[18] | Zoncu R , Perera RM , Sebastian R , Nakatsu F , Chen H , Balla T , Ayala G , Toomre D , De Camilli PV ((2007) ) Loss of endocytic clathrin-coated pits upon acute depletion of phosphatidylinositol 4,5-bisphosphate. Proc Natl Acad Sci U S A 104: , 3793–3798. |
[19] | Perera RM , Zoncu R , Lucast L , De Camilli P , Toomre D ((2006) ) Two synaptojanin 1 isoforms are recruited to clathrin-coated pits at different stages. Proc Natl Acad Sci U S A 103: , 19332–19337. |
[20] | Nakatsu F , Perera RM , Lucast L , Zoncu R , Domin J , Gertler FB , Toomre D , De Camilli P ((2010) ) The inositol 5-phosphatase SHIP2 regulates endocytic clathrin-coated pit dynamics. J Cell Biol 190: , 307–315. |
[21] | Posor Y , Eichhorn-Gruenig M , Puchkov D , Schoneberg J , Ullrich A , Lampe A , Muller R , Zarbakhsh S , Gulluni F , Hirsch E , Krauss M , Schultz C , Schmoranzer J , Noe F , Haucke V ((2013) ) Spatiotemporal control of endocytosis by phosphatidylinositol-3,4-bisphosphate. Nature 499: , 233–237. |
[22] | He K , Marsland R III , Upadhyayula S , Song E , Dang S , Capraro BR , Wang W , Skillern W , Gaudin R , Ma M , Kirchhausen T ((2017) ) Dynamics of phosphoinositide conversion in clathrin-mediated endocytic traffic. Nature 552: , 410–414. |
[23] | McPherson PS , Garcia EP , Slepnev VI , David C , Zhang X , Grabs D , Sossin WS , Bauerfeind R , Nemoto Y , De Camilli P ((1996) ) A presynaptic inositol-5-phosphatase. Nature 379: , 353–357. |
[24] | Cremona O , Di Paolo G , Wenk MR , Luthi A , Kim WT , Takei K , Daniell L , Nemoto Y , Shears SB , Flavell RA , McCormick DA , De Camilli P ((1999) ) Essential role of phosphoinositide metabolism in synaptic vesicle recycling. Cell 99: , 179–188. |
[25] | Zhang X , Hartz PA , Philip E , Racusen LC , Majerus PW ((1998) ) Cell lines from kidney proximal tubules of a patient with Lowe syndrome lack OCRL inositol polyphosphate 5-phosphatase and accumulate phosphatidylinositol 4,5-bisphosphate. J Biol Chem 273: , 1574–1582. |
[26] | Nandez R , Balkin DM , Messa M , Liang L , Paradise S , Czapla H , Hein MY , Duncan JS , Mann M , De Camilli P ((2014) ) A role of OCRL in clathrin-coated pit dynamics and uncoating revealed by studies of Lowe syndrome cells. Elife 3: , e02975. |
[27] | Nakatsu F , Messa M , Nandez R , Czapla H , Zou Y , Strittmatter SM , De Camilli P ((2015) ) Sac2/INPP5F is an inositol 4-phosphatase that functions in the endocytic pathway. J Cell Biol 209: , 85–95. |
[28] | Levine B , Klionsky DJ ((2017) ) Autophagy wins the 2016 Nobel Prize in Physiology or Medicine: Breakthroughs in baker’s yeast fuel advances in biomedical research. Proc Natl Acad Sci U S A 114: , 201–205. |
[29] | Marzella L , Ahlberg J , Glaumann H ((1981) ) Autophagy, heterophagy, microautophagy and crinophagy as the means for intracellular degradation. Virchows Arch B Cell Pathol Incl Mol Pathol 36: , 219–234. |
[30] | Tekirdag K , Cuervo AM ((2018) ) Chaperone-mediated autophagy and endosomal microautophagy: Joint by a chaperone. J Biol Chem 293: , 5414–5424. |
[31] | Birgisdottir AB , Johansen T ((2020) ) Autophagy and endocytosis - interconnections and interdependencies. J Cell Sci 133: , jcs228114. |
[32] | Schuck S ((2020) ) Microautophagy – distinct molecular mechanisms handle cargoes of many sizes. J Cell Sci 133: , jcs246322. |
[33] | Anglade P , Vyas S , Javoy-Agid F , Herrero MT , Michel PP , Marquez J , Mouatt-Prigent A , Ruberg M , Hirsch EC , Agid Y ((1997) ) Apoptosis and autophagy in nigral neurons of patients with Parkinson’s disease. Histol Histopathol 12: , 25–31. |
[34] | Xilouri M , Brekk OR , Stefanis L ((2016) ) Autophagy and alpha-synuclein: Relevance toarkinson’s disease and related synucleopathies. Mov Disord 31: , 178–192. |
[35] | Shahmoradian SH , Lewis AJ , Genoud C , Hench J , Moors TE , Navarro PP , Castaño-Díez D , Schweighauser G , Graff-Meyer A , Goldie KN , Sütterlin R , Huisman E , Ingrassia A , Gier Y , Rozemuller AJM , Wang J , Paepe A , Erny J , Staempfli A , Hoernschemeyer J , Großerüschkamp F , Niedieker D , El-Mashtoly SF , Quadri M , Van IWFJ , Bonifati V , Gerwert K , Bohrmann B , Frank S , Britschgi M , Stahlberg H , Van de Berg WDJ , Lauer ME ((2019) ) Lewy pathology in Parkinson’s disease consists of crowded organelles and lipid membranes. Nat Neurosci 22: , 1099–1109. |
[36] | Palamiuc L , Ravi A , Emerling BM ((2020) ) Phosphoinositides in autophagy: Current roles and future insights. FEBS J 287: , 222–238. |
[37] | Baba T , Balla T ((2020) ) Emerging roles of phosphatidylinositol 4-phosphate and phosphatidylinositol 4,5-bisphosphate as regulators of multiple steps in autophagy. J Biochem 168: , 329–336. |
[38] | Wallroth A , Haucke V ((2018) ) Phosphoinositide conversion in endocytosis and the endolysosomal system. J Biol Chem 293: , 1526–1535. |
[39] | Yu L , McPhee CK , Zheng L , Mardones GA , Rong Y , Peng J , Mi N , Zhao Y , Liu Z , Wan F , Hailey DW , Oorschot V , Klumperman J , Baehrecke EH , Lenardo MJ ((2010) ) Termination of autophagy and reformation of lysosomes regulated by mTOR. Nature 465: , 942–946. |
[40] | Chen Y , Yu L ((2018) ) Development of research into autophagic lysosome reformation. Mol Cells 41: , 45–49. |
[41] | Gurung R , Tan A , Ooms LM , McGrath MJ , Huysmans RD , Munday AD , Prescott M , Whisstock JC , Mitchell CA ((2003) ) Identification of a novel domain in two mammalian inositol-polyphosphate 5-phosphatases that mediates membrane ruffle localization. The inositol 5-phosphatase skip localizes to the endoplasmic reticulum and translocates to membrane ruffles following epidermal growth factor stimulation. J Biol Chem 278: , 11376–11385. |
[42] | McGrath MJ , Eramo MJ , Gurung R , Sriratana A , Gehrig SM , Lynch GS , Lourdes SR , Koentgen F , Feeney SJ , Lazarou M , McLean CA , Mitchell CA ((2021) ) Defective lysosome reformation during autophagy causes skeletal muscle disease. J Clin Invest 131: , e135124. |
[43] | Eramo MJ , Gurung R , Mitchell CA , McGrath MJ ((2021) ) Bidirectional interconversion between PtdIns4P and PtdIns(4,5)P(2) is required for autophagic lysosome reformation and protection from skeletal muscle disease. Autophagy 17: , 1287–1289. |
[44] | Liu G , Zhao Y , Sun JY , Sun BL ((2019) ) Parkinson’s disease risk variant rs1109303 regulates the expression of INPP5K and CRK in human brain. Neurosci Bull 35: , 365–368. |
[45] | Zhu W , Luo X , Adnan A , Yu P , Zhang S , Huo Z , Xu Q , Pang H ((2018) ) Association analysis of NUCKS1 and INPP5K polymorphism with Parkinson’s disease. Genes Genet Syst 93: , 59–64. |
[46] | Hou X , Watzlawik JO , Fiesel FC , Springer W ((2020) ) Autophagy in Parkinson’s disease. J Mol Biol 432: , 2651–2672. |
[47] | Cerri S , Blandini F ((2019) ) Role of autophagy in Parkinson’s disease. Curr Med Chem 26: , 3702–3718. |
[48] | Arotcarena ML , Teil M , Dehay B ((2019) ) Autophagy in synucleinopathy: The overwhelmed and defective machinery. Cells 8: , 565. |
[49] | Azarnia Tehran D , Kuijpers M , Haucke V ((2018) ) Presynaptic endocytic factors in autophagy and neurodegeneration. Curr Opin Neurobiol 48: , 153–159. |
[50] | Pirruccello M , Nandez R , Idevall-Hagren O , Alcazar-Roman A , Abriola L , Berwick SA , Lucast L , Morel D , De Camilli P ((2014) ) Identification of inhibitors of inositol 5-phosphatases through multiple screening strategies. ACS Chem Biol 9: , 1359–1368. |
[51] | Guo S , Stolz LE , Lemrow SM , York JD ((1999) ) SAC1-like domains of yeast SAC1, INP52, and INP53 and of human synaptojanin encode polyphosphoinositide phosphatases. J Biol Chem 274: , 12990–12995. |
[52] | Cao M , Wu Y , Ashrafi G , McCartney AJ , Wheeler H , Bushong EA , Boassa D , Ellisman MH , Ryan TA , De Camilli P ((2017) ) Parkinson sac domain mutation in synaptojanin 1 impairs clathrin uncoating at synapses and triggers dystrophic changes in dopaminergic axons. Neuron 93: , 882–896 e885. |
[53] | Pan PY , Sheehan P , Wang Q , Zhu X , Zhang Y , Choi I , Li X , Saenz J , Zhu J , Wang J , El Gaamouch F , Zhu L , Cai D , Yue Z ((2020) ) Synj1 haploinsufficiency causes dopamine neuron vulnerability and alpha-synuclein accumulation in mice. Hum Mol Genet 29: , 2300–2312. |
[54] | Krebs CE , Karkheiran S , Powell JC , Cao M , Makarov V , Darvish H , Di Paolo G , Walker RH , Shahidi GA , Buxbaum JD , De Camilli P , Yue Z , Paisan-Ruiz C ((2013) ) The Sac1 domain of SYNJ1 identified mutated in a family with early-onset progressive Parkinsonism with generalized seizures. Hum Mutat 34: , 1200–1207. |
[55] | Xie F , Chen S , Cen ZD , Chen Y , Yang DH , Wang HT , Zhang BR , Luo W ((2019) ) A novel homozygous SYNJ1 mutation in two siblings with typical Parkinson’s disease. Parkinsonism Relat Disord 69: , 134–137. |
[56] | Olgiati S , De Rosa A , Quadri M , Criscuolo C , Breedveld GJ , Picillo M , Pappata S , Quarantelli M , Barone P , De Michele G , Bonifati V ((2014) ) PARK20 caused by SYNJ1 homozygous Arg258Gln mutation in a new Italian family. Neurogenetics 15: , 183–188. |
[57] | Quadri M , Fang M , Picillo M , Olgiati S , Breedveld GJ , Graafland J , Wu B , Xu F , Erro R , Amboni M , Pappata S , Quarantelli M , Annesi G , Quattrone A , Chien HF , Barbosa ER , International Parkinsonism Genetics N, Oostra BA , Barone P , Wang J , Bonifati V ((2013) ) Mutation in the SYNJ1 gene associated with autosomal recessive, early-onset Parkinsonism. Hum Mutat 34: , 1208–1215. |
[58] | Chen KH , Wu RM , Lin HI , Tai CH , Lin CH ((2015) ) Mutational analysis of SYNJ1 gene (PARK20) in Parkinson’s disease in a Taiwanese population. Neurobiol Aging 36: , 2905.e2907–2908. |
[59] | Kirola L , Behari M , Shishir C , Thelma BK ((2016) ) Identification of a novel homozygous mutation Arg459Pro in SYNJ1 gene of an Indian family with autosomal recessive juvenile Parkinsonism. Parkinsonism Relat Disord 31: , 124–128. |
[60] | Taghavi S , Chaouni R , Tafakhori A , Azcona LJ , Firouzabadi SG , Omrani MD , Jamshidi J , Emamalizadeh B , Shahidi GA , Ahmadi M , Habibi SAH , Ahmadifard A , Fazeli A , Motallebi M , Petramfar P , Askarpour S , Askarpour S , Shahmohammadibeni HA , Shahmohammadibeni N , Eftekhari H , Shafiei Zarneh AE , Mohammadihosseinabad S , Khorrami M , Najmi S , Chitsaz A , Shokraeian P , Ehsanbakhsh H , Rezaeidian J , Ebrahimi Rad R , Madadi F , Andarva M , Alehabib E , Atakhorrami M , Mortazavi SE , Azimzadeh Z , Bayat M , Besharati AM , Harati-Ghavi MA , Omidvari S , Dehghani-Tafti Z , Mohammadi F , Mohammad Hossein Pour B , Noorollahi Moghaddam H , Esmaili Shandiz E , Habibi A , Taherian-Esfahani Z , Darvish H , Paisán-Ruiz C ((2018) ) A clinical and molecular genetic study of 50 families with autosomal recessive parkinsonism revealed known and novel gene mutations. Mol Neurobiol 55: , 3477–3489. |
[61] | Mani M , Lee SY , Lucast L , Cremona O , Di Paolo G , De Camilli P , Ryan TA ((2007) ) The dual phosphatase activity of synaptojanin1 is required for both efficient synaptic vesicle endocytosis and reavailability at nerve terminals. Neuron 56: , 1004–1018. |
[62] | Ramjaun AR , McPherson PS ((1996) ) Tissue-specific alternative splicing generates two synaptojanin isoforms with differential membrane binding properties. J Biol Chem 271: , 24856–24861. |
[63] | de Heuvel E , Bell AW , Ramjaun AR , Wong K , Sossin WS , McPherson PS ((1997) ) Identification of the major synaptojanin-binding proteins in brain. J Biol Chem 272: , 8710–8716. |
[64] | Ringstad N , Nemoto Y , De Camilli P ((1997) ) The SH3p4/Sh3p8/SH3p13 protein family: Binding partners for synaptojanin and dynamin via a Grb2-like Src homology 3 domain. Proc Natl Acad Sci U S A 94: , 8569–8574. |
[65] | Milosevic I , Giovedi S , Lou X , Raimondi A , Collesi C , Shen H , Paradise S , O’Toole E , Ferguson S , Cremona O , De Camilli P ((2011) ) Recruitment of endophilin to clathrin-coated pit necks is required for efficient vesicle uncoating after fission. Neuron 72: , 587–601. |
[66] | Gad H , Ringstad N , Löw P , Kjaerulff O , Gustafsson J , Wenk M , Di Paolo G , Nemoto Y , Crun J , Ellisman MH , De Camilli P , Shupliakov O , Brodin L ((2000) ) Fission and uncoating of synaptic clathrin-coated vesicles are perturbed by disruption of interactions with the SH3 domain of endophilin. Neuron 27: , 301–312. |
[67] | Vanhauwaert R , Kuenen S , Masius R , Bademosi A , Manetsberger J , Schoovaerts N , Bounti L , Gontcharenko S , Swerts J , Vilain S , Picillo M , Barone P , Munshi ST , de Vrij FM , Kushner SA , Gounko NV , Mandemakers W , Bonifati V , Meunier FA , Soukup SF , Verstreken P ((2017) ) The SAC1 domain in synaptojanin is required for autophagosome maturation at presynaptic terminals. EMBO J 36: , 1392–1411. |
[68] | George AA , Hayden S , Stanton GR , Brockerhoff SE ((2016) ) Arf6 and the 5’phosphatase of synaptojanin 1 regulate autophagy in cone photoreceptors. Bioessays 38: (Suppl 1), S119–135. |
[69] | Hsu F , Hu F , Mao Y ((2015) ) Spatiotemporal control of phosphatidylinositol 4-phosphate by Sac2 regulates endocytic recycling. J Cell Biol 209: , 97–110. |
[70] | Nalls MA , Pankratz N , Lill CM , Do CB , Hernandez DG , Saad M , DeStefano AL , Kara E , Bras J , Sharma M , Schulte C , Keller MF , Arepalli S , Letson C , Edsall C , Stefansson H , Liu X , Pliner H , Lee JH , Cheng R , Ikram MA , Ioannidis JP , Hadjigeorgiou GM , Bis JC , Martinez M , Perlmutter JS , Goate A , Marder K , Fiske B , Sutherland M , Xiromerisiou G , Myers RH , Clark LN , Stefansson K , Hardy JA , Heutink P , Chen H , Wood NW , Houlden H , Payami H , Brice A , Scott WK , Gasser T , Bertram L , Eriksson N , Foroud T , Singleton AB ((2014) ) Large-scale meta-analysis of genome-wide association data identifies six new risk loci for Parkinson’s disease. Nat Genet 46: , 989–993. |
[71] | Cao M , Park D , Wu Y , De Camilli P ((2020) ) Absence of Sac2/INPP5F enhances the phenotype of a Parkinson’s disease mutation of synaptojanin 1. Proc Natl Acad Sci U S A 117: , 12428–12434. |
[72] | Simón-Sánchez J , Schulte C , Bras JM , Sharma M , Gibbs JR , Berg D , Paisan-Ruiz C , Lichtner P , Scholz SW , Hernandez DG , Krüger R , Federoff M , Klein C , Goate A , Perlmutter J , Bonin M , Nalls MA , Illig T , Gieger C , Houlden H , Steffens M , Okun MS , Racette BA , Cookson MR , Foote KD , Fernandez HH , Traynor BJ , Schreiber S , Arepalli S , Zonozi R , Gwinn K , van der Brug M , Lopez G , Chanock SJ , Schatzkin A , Park Y , Hollenbeck A , Gao J , Huang X , Wood NW , Lorenz D , Deuschl G , Chen H , Riess O , Hardy JA , Singleton AB , Gasser T ((2009) ) Genome-wide association study reveals genetic risk underlying Parkinson’s disease. Nat Genet 41: , 1308–1312. |
[73] | Satake W , Nakabayashi Y , Mizuta I , Hirota Y , Ito C , Kubo M , Kawaguchi T , Tsunoda T , Watanabe M , Takeda A , Tomiyama H , Nakashima K , Hasegawa K , Obata F , Yoshikawa T , Kawakami H , Sakoda S , Yamamoto M , Hattori N , Murata M , Nakamura Y , Toda T ((2009) ) Genome-wide association study identifies common variants at four loci as genetic risk factors for Parkinson’s disease. Nat Genet 41: , 1303–1307. |
[74] | Nalls MA , Blauwendraat C , Vallerga CL , Heilbron K , Bandres-Ciga S , Chang D , Tan M , Kia DA , Noyce AJ , Xue A , Bras J , Young E , von Coelln R , Simón-Sánchez J , Schulte C , Sharma M , Krohn L , Pihlstrøm L , Siitonen A , Iwaki H , Leonard H , Faghri F , Gibbs JR , Hernandez DG , Scholz SW , Botia JA , Martinez M , Corvol JC , Lesage S , Jankovic J , Shulman LM , Sutherland M , Tienari P , Majamaa K , Toft M , Andreassen OA , Bangale T , Brice A , Yang J , Gan-Or Z , Gasser T , Heutink P , Shulman JM , Wood NW , Hinds DA , Hardy JA , Morris HR , Gratten J , Visscher PM , Graham RR , Singleton AB ((2019) ) Identification of novel risk loci, causal insights, and heritable risk for Parkinson’s disease: A meta-analysis of genome-wide association studies. Lancet Neurol 18: , 1091–1102. |
[75] | Pals P , Lincoln S , Manning J , Heckman M , Skipper L , Hulihan M , Van den Broeck M , De Pooter T , Cras P , Crook J , Van Broeckhoven C , Farrer MJ ((2004) ) alpha-Synuclein promoter confers susceptibility to Parkinson’s disease. Ann Neurol 56: , 591–595. |
[76] | Singleton AB , Farrer M , Johnson J , Singleton A , Hague S , Kachergus J , Hulihan M , Peuralinna T , Dutra A , Nussbaum R , Lincoln S , Crawley A , Hanson M , Maraganore D , Adler C , Cookson MR , Muenter M , Baptista M , Miller D , Blancato J , Hardy J , Gwinn-Hardy K ((2003) ) alpha-Synuclein locus triplication causes Parkinson’s disease. Science 302: , 841. |
[77] | Chartier-Harlin MC , Kachergus J , Roumier C , Mouroux V , Douay X , Lincoln S , Levecque C , Larvor L , Andrieux J , Hulihan M , Waucquier N , Defebvre L , Amouyel P , Farrer M , Destée A ((2004) ) Alpha-synuclein locus duplication as a cause of familial Parkinson’s disease. Lancet 364: , 1167–1169. |
[78] | Ibanez P , Bonnet AM , Debarges B , Lohmann E , Tison F , Pollak P , Agid Y , Durr A , Brice A ((2004) ) Causal relation between alpha-synuclein gene duplication and familial Parkinson’s disease. Lancet 364: , 1169–1171. |
[79] | Polymeropoulos MH , Lavedan C , Leroy E , Ide SE , Dehejia A , Dutra A , Pike B , Root H , Rubenstein J , Boyer R , Stenroos ES , Chandrasekharappa S , Athanassiadou A , Papapetropoulos T , Johnson WG , Lazzarini AM , Duvoisin RC , Di Iorio G , Golbe LI , Nussbaum RL ((1997) ) Mutation in the alpha-synuclein gene identified in families with Parkinson’s disease. Science 276: , 2045–2047. |
[80] | Kruger R , Kuhn W , Muller T , Woitalla D , Graeber M , Kosel S , Przuntek H , Epplen JT , Schols L , Riess O ((1998) ) Ala30Pro mutation in the gene encoding alpha-synuclein in Parkinson’s disease. Nat Genet 18: , 106–108. |
[81] | Zarranz JJ , Alegre J , Gomez-Esteban JC , Lezcano E , Ros R , Ampuero I , Vidal L , Hoenicka J , Rodriguez O , Atares B , Llorens V , Gomez Tortosa E , del Ser T , Munoz DG , de Yebenes JG ((2004) ) The new mutation, E46K, of alpha-synuclein causes Parkinson and Lewy body dementia. Ann Neurol 55: , 164–173. |
[82] | Appel-Cresswell S , Vilarino-Guell C , Encarnacion M , Sherman H , Yu I , Shah B , Weir D , Thompson C , Szu-Tu C , Trinh J , Aasly JO , Rajput A , Rajput AH , Jon Stoessl A , Farrer MJ ((2013) ) Alpha-synuclein p.H50Q, a novel pathogenic mutation for Parkinson’s disease. Mov Disord 28: , 811–813. |
[83] | Proukakis C , Dudzik CG , Brier T , MacKay DS , Cooper JM , Millhauser GL , Houlden H , Schapira AH ((2013) ) A novel α-synuclein missense mutation in Parkinson disease. Neurology 80: , 1062–1064. |
[84] | Kiely AP , Asi YT , Kara E , Limousin P , Ling H , Lewis P , Proukakis C , Quinn N , Lees AJ , Hardy J , Revesz T , Houlden H , Holton JL ((2013) ) α-Synucleinopathy associated with G51D SNCA mutation: A link between Parkinson’s disease and multiple system atrophy? Acta Neuropathol 125: , 753–769. |
[85] | Lesage S , Anheim M , Letournel F , Bousset L , Honoré A , Rozas N , Pieri L , Madiona K , Dürr A , Melki R , Verny C , Brice A ((2013) ) G51D α-synuclein mutation causes a novel parkinsonian-pyramidal syndrome. Ann Neurol 73: , 459–471. |
[86] | Pasanen P , Myllykangas L , Siitonen M , Raunio A , Kaakkola S , Lyytinen J , Tienari PJ , Pöyhönen M , Paetau A ((2014) ) Novel α-synuclein mutation A53E associated with atypical multiple system atrophy and Parkinson’s disease-type pathology. Neurobiol Aging 35: , 2180. e2181–2185. |
[87] | Martikainen MH , Päivärinta M , Hietala M , Kaasinen V ((2015) ) Clinical and imaging findings in Parkinson disease associated with the A53E SNCA mutation. Neurol Genet 1: , e27. |
[88] | Spillantini MG , Schmidt ML , Lee VM , Trojanowski JQ , Jakes R , Goedert M ((1997) ) Alpha-synuclein in Lewy bodies. Nature 388: , 839–840. |
[89] | Lewy FH ((1913) ) Zur pathologischen anatomie der paralysis agitans. Dtsch Z Nervenheilkd 50: , 50–55. |
[90] | Lashuel HA ((2020) ) Do Lewy bodies contain alpha-synuclein fibrils? and Does it matter? A brief history and critical analysis of recent reports. Neurobiol Dis 141: , 104876. |
[91] | Galvin JE , Lee VM , Trojanowski JQ ((2001) ) Synucleinopathies: Clinical and pathological implications. Arch Neurol 58: , 186–190. |
[92] | Davidson WS , Jonas A , Clayton DF , George JM ((1998) ) Stabilization of alpha-synuclein secondary structure upon binding to synthetic membranes. J Biol Chem 273: , 9443–9449. |
[93] | Stockl M , Fischer P , Wanker E , Herrmann A ((2008) ) Alpha-synuclein selectively binds to anionic phospholipids embedded in liquid-disordered domains. J Mol Biol 375: , 1394–1404. |
[94] | Bodner CR , Maltsev AS , Dobson CM , Bax A ((2010) ) Differential phospholipid binding of alpha-synuclein variants implicated in Parkinson’s disease revealed by solution NMR spectroscopy. Biochemistry 49: , 862–871. |
[95] | Maroteaux L , Campanelli JT , Scheller RH ((1988) ) Synuclein: A neuron-secific protein localized to the nucleus and presynaptic nerve terminal. J Neurosci 8: , 2804–2815. |
[96] | Zarbiv Y , Simhi-Haham D , Israeli E , Elhadi SA , Grigoletto J , Sharon R ((2014) ) Lysine residues at the first and second KTKEGV repeats mediate alpha-Synuclein binding to membrane phospholipids. Neurobiol Dis 70: , 90–98. |
[97] | Fanning S , Selkoe D , Dettmer U ((2020) ) Parkinson’s disease: Proteinopathy or lipidopathy? NPJ Parkinsons Dis 6: , 3. |
[98] | Weinreb PH , Zhen W , Poon AW , Conway KA , Lansbury PT Jr. ((1996) ) NACP, a protein implicated in Alzheimer’s disease and learning, is natively unfolded. Biochemistry 35: , 13709–13715. |
[99] | Kaur U , Lee JC ((2020) ) Unroofing site-specific α-synuclein– lipid interactions at the plasma membrane. Proc Natl Acad Sci U S A 117: , 18977–18983. |
[100] | Jao CC , Hegde BG , Chen J , Haworth IS , Langen R ((2008) ) Structure of membrane-bound alpha-synuclein from site-directed spin labeling and computational refinement. Proc Natl Acad Sci U S A 105: , 19666–19671. |
[101] | Fusco G , Pape T , Stephens AD , Mahou P , Costa AR , Kaminski CF , Kaminski Schierle GS , Vendruscolo M , Veglia G , Dobson CM , De Simone A ((2016) ) Structural basis of synaptic vesicle assembly promoted by alpha-synuclein. Nat Commun 7: , 12563. |
[102] | Wietek J , Haralampiev I , Amoussouvi A , Herrmann A , Stockl M ((2013) ) Membrane bound alpha-synuclein is fully embedded in the lipid bilayer while segments with higher flexibility remain. FEBS Lett 587: , 2572–2577. |
[103] | Tsigelny IF , Sharikov Y , Wrasidlo W , Gonzalez T , Desplats PA , Crews L , Spencer B , Masliah E ((2012) ) Role of α-synuclein penetration into the membrane in the mechanisms of oligomer pore formation. FEBS J 279: , 1000–1013. |
[104] | Cheng CY , Varkey J , Ambroso MR , Langen R , Han S ((2013) ) Hydration dynamics as an intrinsic ruler for refining protein structure at lipid membrane interfaces. Proc Natl Acad Sci U S A 110: , 16838–16843. |
[105] | Antonny B ((2011) ) Mechanisms of membrane curvature sensing. Annu Rev Biochem 80: , 101–123. |
[106] | Chung PJ , Hwang HL , Slaw BR , Leong A , Adams EJ , Lee KYC ((2020) ) The C-terminal domain of α-synuclein confers steric stabilization on synaptic vesicle-like surfaces. Adv Mater Interfaces 7: , 1902151. |
[107] | Cabin DE , Shimazu K , Murphy D , Cole NB , Gottschalk W , McIlwain KL , Orrison B , Chen A , Ellis CE , Paylor R , Lu B , Nussbaum RL ((2002) ) Synaptic vesicle depletion correlates with attenuated synaptic responses to prolonged repetitive stimulation in mice lacking alpha-synuclein. J Neurosci 22: , 8797–8807. |
[108] | Murphy DD , Rueter SM , Trojanowski JQ , Lee VM ((2000) ) Synucleins are developmentally expressed, and alpha-synuclein regulates the size of the presynaptic vesicular pool in primary hippocampal neurons. J Neurosci 20: , 3214–3220. |
[109] | Scott D , Roy S ((2012) ) alpha-Synuclein inhibits intersynaptic vesicle mobility and maintains recycling-pool homeostasis. J Neurosci 32: , 10129–10135. |
[110] | Nemani VM , Lu W , Berge V , Nakamura K , Onoa B , Lee MK , Chaudhry FA , Nicoll RA , Edwards RH ((2010) ) Increased expression of alpha-synuclein reduces neurotransmitter release by inhibiting synaptic vesicle reclustering after endocytosis. Neuron 65: , 66–79. |
[111] | Golovko MY , Barcelo-Coblijn G , Castagnet PI , Austin S , Combs CK , Murphy EJ ((2009) ) The role of alpha-synuclein in brain lipid metabolism: A downstream impact on brain inflammatory response. Mol Cell Biochem 326: , 55–66. |
[112] | Sharon R , Bar-Joseph I , Mirick GE , Serhan CN , Selkoe DJ ((2003) ) Altered fatty acid composition of dopaminergic neurons expressing alpha-synuclein and human brains with alpha-synucleinopathies. J Biol Chem 278: , 49874–49881. |
[113] | Fanning S , Haque A , Imberdis T , Baru V , Barrasa MI , Nuber S , Termine D , Ramalingam N , Ho GPH , Noble T , Sandoe J , Lou Y , Landgraf D , Freyzon Y , Newby G , Soldner F , Terry-Kantor E , Kim TE , Hofbauer HF , Becuwe M , Jaenisch R , Pincus D , Clish CB , Walther TC , Farese RV Jr. , Srinivasan S , Welte MA , Kohlwein SD , Dettmer U , Lindquist S , Selkoe D ((2019) ) Lipidomic analysis of α-synuclein neurotoxicity identifies stearoyl CoA desaturase as a target for parkinson treatment. Mol Cell 73: , 1001–1014.e1008. |
[114] | Grigoletto J , Pukass K , Gamliel A , Davidi D , Katz-Brull R , Richter-Landsberg C , Sharon R ((2017) ) Higher levels of myelin phospholipids in brains of neuronal alpha-synuclein transgenic mice precede myelin loss. Acta Neuropathol Commun 5: , 37. |
[115] | Cole NB , Murphy DD , Grider T , Rueter S , Brasaemle D , Nussbaum RL ((2002) ) Lipid droplet binding and oligomerization properties of the Parkinson’s disease protein alpha-synuclein. J Biol Chem 277: , 6344–6352. |
[116] | Outeiro TF , Lindquist S ((2003) ) Yeast cells provide insight into alpha-synuclein biology and pathobiology. Science 302: , 1772–1775. |
[117] | Snead D , Eliezer D ((2014) ) Alpha-synuclein function and dysfunction on cellular membranes. Exp Neurobiol 23: , 292–313. |
[118] | Alza NP , Iglesias González PA , Conde MA , Uranga RM , Salvador GA ((2019) ) Lipids at the crossroad of α-synuclein function and dysfunction: Biological and pathological implications. Front Cell Neurosci 13: , 175. |
[119] | Alecu I , Bennett SAL ((2019) ) Dysregulated lipid metabolism and its role in α-synucleinopathy in Parkinson’s disease. Front Neurosci 13: , 328. |
[120] | O’Leary EI , Lee JC ((2019) ) Interplay between α-synuclein amyloid formation and membrane structure. Biochim Biophys Acta Proteins Proteom 1867: , 483–491. |
[121] | Soper JH , Kehm V , Burd CG , Bankaitis VA , Lee VM ((2011) ) Aggregation of α-synuclein in S. cerevisiae is associated with defects in endosomal trafficking and phospholipid biosynthesis. J Mol Neurosci 43: , 391–405. |
[122] | Bosio A , Binczek E , Haupt WF , Stoffel W ((1998) ) Composition and biophysical properties of myelin lipid define the neurological defects in galactocerebroside- and sulfatide-deficient mice. J Neurochem 70: , 308–315. |
[123] | Don AS , Hsiao JH , Bleasel JM , Couttas TA , Halliday GM , Kim WS ((2014) ) Altered lipid levels provide evidence for myelin dysfunction in multiple system atrophy. Acta Neuropathol Commun 2: , 150. |
[124] | Soll LG , Eisen JN , Vargas KJ , Medeiros AT , Hammar KM , Morgan JR ((2020) ) α-Synuclein-112 impairs synaptic vesicle recycling consistent with its enhanced membrane binding properties. Front Cell Dev Biol 8: , 405. |
[125] | Schechter M , Grigoletto J , Abd-Elhadi S , Glickstein H , Friedman A , Serrano GE , Beach TG , Sharon R ((2020) ) A role for alpha-Synuclein in axon growth and its implications in corticostriatal glutamatergic plasticity in Parkinson’s disease. Mol Neurodegener 15: , 24. |
[126] | Eichmann C , Jacob RS , Dema A , Mercadante D , Selenko P ((2020) ) α-Synuclein plasma membrane localization correlates with cellular phosphatidylinositol polyphosphate levels. bioRxiv, 2020.2007.2031.224600. |
[127] | Narayanan V , Guo Y , Scarlata S ((2005) ) Fluorescence studies suggest a role for alpha-synuclein in the phosphatidylinositol lipid signaling pathway. Biochemistry 44: , 462–470. |
[128] | Schechter M , Atias M , Abd Elhadi S , Davidi D , Gitler D , Sharon R ((2020) ) alpha-Synuclein facilitates endocytosis by elevating the steady-state levels of phosphatidylinositol 4,5-bisphosphate. J Biol Chem 295: , 18076–18090. |
[129] | Scherzer CR , Feany MB ((2004) ) Yeast genetics targets lipids in Parkinson’s disease. Trends Genet 20: , 273–277. |
[130] | Khurana V , Peng J , Chung CY , Auluck PK , Fanning S , Tardiff DF , Bartels T , Koeva M , Eichhorn SW , Benyamini H , Lou Y , Nutter-Upham A , Baru V , Freyzon Y , Tuncbag N , Costanzo M , San Luis BJ , Schöndorf DC , Barrasa MI , Ehsani S , Sanjana N , Zhong Q , Gasser T , Bartel DP , Vidal M , Deleidi M , Boone C , Fraenkel E , Berger B , Lindquist S ((2017) ) Genome-scale networks link neurodegenerative disease genes to α-synuclein through specific molecular pathways. Cell Syst 4: , 157–170.e114. |
[131] | Davidi D , Schechter M , Matatov A , Nathanzon L , Sharon R ((2020) ) α-Synuclein translocates to the nucleus to activate retinoic acid-dependent gene transcription. iScience 23: , 100910. |
[132] | Eschbach J , von Einem B , Muller K , Bayer H , Scheffold A , Morrison BE , Rudolph KL , Thal DR , Witting A , Weydt P , Otto M , Fauler M , Liss B , McLean PJ , Spada AR , Ludolph AC , Weishaupt JH , Danzer KM ((2015) ) Mutual exacerbation of peroxisome proliferator-activated receptor gamma coactivator 1alpha deregulation and alpha-synuclein oligomerization. Ann Neurol 77: , 15–32. |
[133] | Zheng B , Liao Z , Locascio JJ , Lesniak KA , Roderick SS , Watt ML , Eklund AC , Zhang-James Y , Kim PD , Hauser MA , Grunblatt E , Moran LB , Mandel SA , Riederer P , Miller RM , Federoff HJ , Wullner U , Papapetropoulos S , Youdim MB , Cantuti-Castelvetri I , Young AB , Vance JM , Davis RL , Hedreen JC , Adler CH , Beach TG , Graeber MB , Middleton FA , Rochet JC , Scherzer CR ((2010) ) PGC-1alpha, a potential therapeutic target for early intervention in Parkinson’s disease. Sci Transl Med 2: , 52ra73. |
[134] | Ben Gedalya T , Loeb V , Israeli E , Altschuler Y , Selkoe DJ , Sharon R ((2009) ) Alpha-synuclein and polyunsaturated fatty acids promote clathrin-mediated endocytosis and synaptic vesicle recycling. Traffic 10: , 218–234. |
[135] | Pinot M , Vanni S , Pagnotta S , Lacas-Gervais S , Payet LA , Ferreira T , Gautier R , Goud B , Antonny B , Barelli H ((2014) ) Lipid cell biology. Polyunsaturated phospholipids facilitate membrane deformation and fission by endocytic proteins. Science 345: , 693–697. |
[136] | George JM , Jin H , Woods WS , Clayton DF ((1995) ) Characterization of a novel protein regulated during the critical period for song learning in the zebra finch. Neuron 15: , 361–372. |
[137] | Vargas KJ , Makani S , Davis T , Westphal CH , Castillo PE , Chandra SS ((2014) ) Synucleins regulate the kinetics of synaptic vesicle endocytosis. J Neurosci 34: , 9364–9376. |
[138] | Vargas KJ , Colosi PL , Girardi E , Chandra SS ((2020) ) α-Synuclein facilitates clathrin assembly in synaptic vesicle endocytosis. bioRxiv, 2020.2004.2029.069344. |
[139] | Vargas KJ , Schrod N , Davis T , Fernandez-Busnadiego R , Taguchi YV , Laugks U , Lucic V , Chandra SS ((2017) ) Synucleins have multiple effects on presynaptic architecture. Cell Rep 18: , 161–173. |
[140] | Ferguson SM , Brasnjo G , Hayashi M , Wolfel M , Collesi C , Giovedi S , Raimondi A , Gong LW , Ariel P , Paradise S , O’Toole E , Flavell R , Cremona O , Miesenbock G , Ryan TA , De Camilli P ((2007) ) A selective activity-dependent requirement for dynamin 1 in synaptic vesicle endocytosis. Science 316: , 570–574. |
[141] | Raimondi A , Ferguson SM , Lou X , Armbruster M , Paradise S , Giovedi S , Messa M , Kono N , Takasaki J , Cappello V , O’Toole E , Ryan TA , De Camilli P ((2011) ) Overlapping role of dynamin isoforms in synaptic vesicle endocytosis. Neuron 70: , 1100–1114. |
[142] | Banks SML , Medeiros AT , McQuillan M , Busch DJ , Ibarraran-Viniegra AS , Sousa R , Lafer EM , Morgan JR ((2020) ) Hsc70 ameliorates the vesicle recycling defects caused by excess α-synuclein at synapses. eNeuro 7: , ENEURO.0448-19.2020. |
[143] | Xu J , Wu XS , Sheng J , Zhang Z , Yue HY , Sun L , Sgobio C , Lin X , Peng S , Jin Y , Gan L , Cai H , Wu LG ((2016) ) alpha-Synuclein mutation inhibits endocytosis at mammalian central nerve terminals. J Neurosci 36: , 4408–4414. |
[144] | Busch DJ , Oliphint PA , Walsh RB , Banks SM , Woods WS , George JM , Morgan JR ((2014) ) Acute increase of alpha-synuclein inhibits synaptic vesicle recycling evoked during intense stimulation. Mol Biol Cell 25: , 3926–3941. |
[145] | Scott DA , Tabarean I , Tang Y , Cartier A , Masliah E , Roy S ((2010) ) A pathologic cascade leading to synaptic dysfunction in alpha-synuclein-induced neurodegeneration. J Neurosci 30: , 8083–8095. |
[146] | Lautenschlager J , Kaminski CF , Kaminski Schierle GS ((2017) ) alpha-Synuclein - regulator of exocytosis, endocytosis, or both? Trends Cell Biol 27: , 468–479. |
[147] | Sulzer D , Edwards RH ((2019) ) The physiological role of alpha-synuclein and its relationship to Parkinson’s disease. J Neurochem 150: , 475–486. |
[148] | Burre J , Sharma M , Tsetsenis T , Buchman V , Etherton MR , Sudhof TC ((2010) ) Alpha-synuclein promotes SNARE-complex assembly in vivo and in vitro. Science 329: , 1663–1667. |
[149] | Chandra S , Gallardo G , Fernandez-Chacon R , Schluter OM , Sudhof TC ((2005) ) Alpha-synuclein cooperates with CSPalpha in preventing neurodegeneration. Cell 123: , 383–396. |
[150] | Burre J , Sharma M , Sudhof TC ((2014) ) alpha-Synuclein assembles into higher-order multimers upon membrane binding to promote SNARE complex formation. Proc Natl Acad Sci U S A 111: , E4274–4283. |
[151] | Sun J , Wang L , Bao H , Premi S , Das U , Chapman ER , Roy S ((2019) ) Functional cooperation of alpha-synuclein and VAMP2 in synaptic vesicle recycling. Proc Natl Acad Sci U S A 116: , 11113–11115. |
[152] | DeWitt DC , Rhoades E ((2013) ) alpha-Synuclein can inhibit SNARE-mediated vesicle fusion through direct interactions with lipid bilayers. Biochemistry 52: , 2385–2387. |
[153] | Lai Y , Kim S , Varkey J , Lou X , Song JK , Diao J , Langen R , Shin YK ((2014) ) Nonaggregated alpha-synuclein influences SNARE-dependent vesicle docking via membrane binding. Biochemistry 53: , 3889–3896. |
[154] | Larsen KE , Schmitz Y , Troyer MD , Mosharov E , Dietrich P , Quazi AZ , Savalle M , Nemani V , Chaudhry FA , Edwards RH , Stefanis L , Sulzer D ((2006) ) Alpha-synuclein overexpression in PC12 and chromaffin cells impairs catecholamine release by interfering with a late step in exocytosis. J Neurosci 26: , 11915–11922. |
[155] | Lundblad M , Decressac M , Mattsson B , Bjorklund A ((2012) ) Impaired neurotransmission caused by overexpression of alpha-synuclein in nigral dopamine neurons. Proc Natl Acad Sci U S A 109: , 3213–3219. |
[156] | Logan T , Bendor J , Toupin C , Thorn K , Edwards RH ((2017) ) alpha-Synuclein promotes dilation of the exocytotic fusion pore. Nat Neurosci 20: , 681–689. |
[157] | Suh BC , Inoue T , Meyer T , Hille B ((2006) ) Rapid chemically induced changes of PtdIns(4,5)P2 gate KCNQ ion channels. Science 314: , 1454–1457. |
[158] | Martin TF ((2015) ) PI(4,5)P(2)-binding effector proteins for vesicle exocytosis. Biochim Biophys Acta 1851: , 785–793. |
[159] | Martin TF ((2012) ) Role of PI(4,5)P(2) in vesicle exocytosis and membrane fusion. Subcell Biochem 59: , 111–130. |
[160] | Martens S , Kozlov MM , McMahon HT ((2007) ) How synaptotagmin promotes membrane fusion. Science 316: , 1205–1208. |
[161] | Lynch KL , Gerona RR , Kielar DM , Martens S , McMahon HT , Martin TF ((2008) ) Synaptotagmin-1 utilizes membrane bending and SNARE binding to drive fusion pore expansion. Mol Biol Cell 19: , 5093–5103. |
[162] | Hui E , Johnson CP , Yao J , Dunning FM , Chapman ER ((2009) ) Synaptotagmin-mediated bending of the target membrane is a critical step in Ca(2+)-regulated fusion. Cell 138: , 709–721. |
[163] | Shin OH , Lu J , Rhee JS , Tomchick DR , Pang ZP , Wojcik SM , Camacho-Perez M , Brose N , Machius M , Rizo J , Rosenmund C , Südhof TC ((2010) ) Munc13 C2B domain is an activity-dependent Ca2+regulator of synaptic exocytosis. Nat Struct Mol Biol 17: , 280–288. |
[164] | Berberian K , Torres AJ , Fang Q , Kisler K , Lindau M ((2009) ) F-actin and myosin II accelerate catecholamine release from chromaffin granules. J Neurosci 29: , 863–870. |
[165] | Cuervo AM , Stefanis L , Fredenburg R , Lansbury PT , Sulzer D ((2004) ) Impaired degradation of mutant alpha-synuclein by chaperone-mediated autophagy. Science 305: , 1292–1295. |
[166] | Mak SK , McCormack AL , Manning-Bog AB , Cuervo AM , Di Monte DA ((2010) ) Lysosomal degradation of alpha-synuclein in vivo. J Biol Chem 285: , 13621–13629. |
[167] | Vogiatzi T , Xilouri M , Vekrellis K , Stefanis L ((2008) ) Wild type alpha-synuclein is degraded by chaperone-mediated autophagy and macroautophagy in neuronal cells. J Biol Chem 283: , 23542–23556. |
[168] | Huang Y , Chegini F , Chua G , Murphy K , Gai W , Halliday GM ((2012) ) Macroautophagy in sporadic and the genetic form of Parkinson’s disease with the A53T α-synuclein mutation. Transl Neurodegener 1: , 2. |
[169] | Xilouri M , Vogiatzi T , Vekrellis K , Park D , Stefanis L ((2009) ) Abberant alpha-synuclein confers toxicity to neurons in part through inhibition of chaperone-mediated autophagy. PLoS One 4: , e5515. |
[170] | Winslow AR , Chen CW , Corrochano S , Acevedo-Arozena A , Gordon DE , Peden AA , Lichtenberg M , Menzies FM , Ravikumar B , Imarisio S , Brown S , O’Kane CJ , Rubinsztein DC ((2010) ) α-Synuclein impairs macroautophagy: Implications for Parkinson’s disease. J Cell Biol 190: , 1023–1037. |
[171] | Sarkar S , Olsen AL , Sygnecka K , Lohr KM , Feany MB ((2021) ) α-synuclein impairs autophagosome maturation through abnormal actin stabilization. PLoS Genet 17: , e1009359. |
[172] | Berwick DC , Heaton GR , Azeggagh S , Harvey K ((2019) ) LRRK2 Biology from structure to dysfunction: Research progresses, but the themes remain the same. Mol Neurodegener 14: , 49. |
[173] | Zimprich A , Biskup S , Leitner P , Lichtner P , Farrer M , Lincoln S , Kachergus J , Hulihan M , Uitti RJ , Calne DB , Stoessl AJ , Pfeiffer RF , Patenge N , Carbajal IC , Vieregge P , Asmus F , Müller-Myhsok B , Dickson DW , Meitinger T , Strom TM , Wszolek ZK , Gasser T ((2004) ) Mutations in LRRK2 cause autosomal-dominant parkinsonism with pleomorphic pathology. Neuron 44: , 601–607. |
[174] | Albanese F , Novello S , Morari M ((2019) ) Autophagy and LRRK2 in the aging brain. Front Neurosci 13: , 1352. |
[175] | Paisan-Ruiz C , Jain S , Evans EW , Gilks WP , Simon J , van der Brug M , Lopez de Munain A , Aparicio S , Gil AM , Khan N , Johnson J , Martinez JR , Nicholl D , Marti Carrera I , Pena AS , de Silva R , Lees A , Marti-Masso JF , Perez-Tur J , Wood NW , Singleton AB ((2004) ) Cloning of the gene containing mutations that cause PARK8-linked Parkinson’s disease. Neuron 44: , 595–600. |
[176] | Ling H , Kara E , Bandopadhyay R , Hardy J , Holton J , Xiromerisiou G , Lees A , Houlden H , Revesz T ((2013) ) TDP-43 pathology in a patient carrying G2019S LRRK2 mutation and a novel p.Q124E MAPT. Neurobiol Aging 34: , 2889.e2885–2889. |
[177] | O’Hara DM , Pawar G , Kalia SK , Kalia LV ((2020) ) LRRK2 and α-synuclein: Distinct or synergisticlayers in Parkinson’s disease? Front Neurosci 14: , 577. |
[178] | Henderson MX , Sengupta M , Trojanowski JQ , Lee VMY ((2019) ) Alzheimer’s disease tau is a prominent pathology in LRRK2 Parkinson’s disease. Acta Neuropathol Commun 7: , 183. |
[179] | Purlyte E , Dhekne HS , Sarhan AR , Gomez R , Lis P , Wightman M , Martinez TN , Tonelli F , Pfeffer SR , Alessi DR ((2018) ) Rab29 activation of the Parkinson’s disease-associated LRRK2 kinase. EMBO J 37: , 1–18. |
[180] | Liu Z , Bryant N , Kumaran R , Beilina A , Abeliovich A , Cookson MR , West AB ((2018) ) LRRK2 phosphorylates membrane-bound Rabs and is activated by GTP-bound Rab7L1 to promote recruitment to the trans-Golgi network. Hum Mol Genet 27: , 385–395. |
[181] | MacLeod David A , Rhinn H , Kuwahara T , Zolin A , Di Paolo G , McCabe Brian D , Marder Karen S , Honig Lawrence S , Clark Lorraine N , Small Scott A , Abeliovich A ((2013) ) RAB7L1 interacts with LRRK2 to modify intraneuronal protein sorting and Parkinson’s disease risk. Neuron 77: , 425–439. |
[182] | Mir R , Tonelli F , Lis P , Macartney T , Polinski NK , Martinez TN , Chou MY , Howden AJM , König T , Hotzy C , Milenkovic I , Brücke T , Zimprich A , Sammler E , Alessi DR ((2018) ) The Parkinson’s disease VPS35[D620N] mutation enhances LRRK2-mediated Rab protein phosphorylation in mouse and human. Biochem J 475: , 1861–1883. |
[183] | Taylor M , Alessi DR ((2020) ) Advances in elucidating the function of leucine-rich repeat protein kinase-2 in normal cells and Parkinson’s disease. Curr Opin Cell Biol 63: , 102–113. |
[184] | Arranz AM , Delbroek L , Van Kolen K , Guimaraes MR , Mandemakers W , Daneels G , Matta S , Calafate S , Shaban H , Baatsen P , De Bock PJ , Gevaert K , Vanden Berghe P , Verstreken P , De Strooper B , Moechars D ((2015) ) LRRK2 functions in synaptic vesicle endocytosis through a kinase-dependent mechanism. J Cell Sci 128: , 541–552. |
[185] | Matta S , Van Kolen K , da Cunha R , van den Bogaart G , Mandemakers W , Miskiewicz K , De Bock PJ , Morais VA , Vilain S , Haddad D , Delbroek L , Swerts J , Chavez-Gutierrez L , Esposito G , Daneels G , Karran E , Holt M , Gevaert K , Moechars DW , De Strooper B , Verstreken P ((2012) ) LRRK2 controls an EndoA phosphorylation cycle in synaptic endocytosis. Neuron 75: , 1008–1021. |
[186] | Pan PY , Li X , Wang J , Powell J , Wang Q , Zhang Y , Chen Z , Wicinski B , Hof P , Ryan TA , Yue Z ((2017) ) Parkinson’s disease-associated LRRK2 hyperactive kinase mutant disrupts synaptic vesicle trafficking in ventral midbrain neurons. J Neurosci 37: , 11366–11376. |
[187] | Heaton GR , Landeck N , Mamais A , Nalls MA , Nixon-Abell J , Kumaran R , Beilina A , Pellegrini L , Li Y , International Parkinson Disease Genomics Consortium, Harvey K , Cookson MR ((2020) ) Sequential screening nominates the Parkinson’s disease associated kinase LRRK2 as a regulator of Clathrin-mediated endocytosis. Neurobiol Dis 141: , 104948. |
[188] | Nguyen M , Krainc D ((2018) ) LRRK2 phosphorylation of auxilin mediates synaptic defects in dopaminergic neurons from patients with Parkinson’s disease. Proc Natl Acad Sci U S A 115: , 5576–5581. |
[189] | Stafa K , Tsika E , Moser R , Musso A , Glauser L , Jones A , Biskup S , Xiong Y , Bandopadhyay R , Dawson VL , Dawson TM , Moore DJ ((2014) ) Functional interaction of Parkinson’s disease-associated LRRK2 with members of the dynamin GTPase superfamily. Hum Mol Genet 23: , 2055–2077. |
[190] | Islam MS , Nolte H , Jacob W , Ziegler AB , Pütz S , Grosjean Y , Szczepanowska K , Trifunovic A , Braun T , Heumann H , Heumann R , Hovemann B , Moore DJ , Krüger M ((2016) ) Human R1441C LRRK2 regulates the synaptic vesicle proteome and phosphoproteome in a Drosophila model of Parkinson’s disease. Hum Mol Genet 25: , 5365–5382. |
[191] | Schreij AM , Chaineau M , Ruan W , Lin S , Barker PA , Fon EA , McPherson PS ((2015) ) LRRK2 localizes to endosomes and interacts with clathrin-light chains to limit Rac1 activation. EMBO Rep 16: , 79–86. |
[192] | Liu Q , Bautista-Gomez J , Higgins DA , Yu J , Xiong Y ((2020) ) LRRK2 regulates AP2M1 phosphorylation cycles to mediate endocytosis and dopaminergic neurodegeneration. bioRxiv, 2020.2005.2027.119388. |
[193] | Madureira M , Connor-Robson N , Wade-Martins R ((2020) ) “LRRK2: Autophagy and lysosomal activity”. Front Neurosci 14: , 498. |
[194] | Orenstein SJ , Kuo SH , Tasset I , Arias E , Koga H , Fernandez-Carasa I , Cortes E , Honig LS , Dauer W , Consiglio A , Raya A , Sulzer D , Cuervo AM ((2013) ) Interplay of LRRK2 with chaperone-mediated autophagy. Nat Neurosci 16: , 394–406. |
[195] | Sánchez-Danés A , Richaud-Patin Y , Carballo-Carbajal I , Jiménez-Delgado S , Caig C , Mora S , Di Guglielmo C , Ezquerra M , Patel B , Giralt A , Canals JM , Memo M , Alberch J , López-Barneo J , Vila M , Cuervo AM , Tolosa E , Consiglio A , Raya A ((2012) ) Disease-specific phenotypes in dopamine neurons from human iPS-based models of genetic and sporadic Parkinson’s disease. EMBO Mol Med 4: , 380–395. |
[196] | Nguyen HN , Byers B , Cord B , Shcheglovitov A , Byrne J , Gujar P , Kee K , Schüle B , Dolmetsch RE , Langston W , Palmer TD , Pera RR ((2011) ) LRRK2 mutant iPSC-derived DA neurons demonstrate increased susceptibility to oxidative stress. Cell Stem Cell 8: , 267–280. |
[197] | Reinhardt P , Schmid B , Burbulla LF , Schöndorf DC , Wagner L , Glatza M , Höing S , Hargus G , Heck SA , Dhingra A , Wu G , Müller S , Brockmann K , Kluba T , Maisel M , Krüger R , Berg D , Tsytsyura Y , Thiel CS , Psathaki OE , Klingauf J , Kuhlmann T , Klewin M , Müller H , Gasser T , Schöler HR , Sterneckert J ((2013) ) Genetic correction of a LRRK2 mutation in human iPSCs links parkinsonian neurodegeneration to ERK-dependent changes in gene expression. Cell Stem Cell 12: , 354–367. |
[198] | Ho PW , Leung CT , Liu H , Pang SY , Lam CS , Xian J , Li L , Kung MH , Ramsden DB , Ho SL ((2020) ) Age-dependent accumulation of oligomeric SNCA/α-synuclein from impaired degradation in mutant LRRK2 knockin mouse model of Parkinson disease: Role for therapeutic activation of chaperone-mediated autophagy (CMA). Autophagy 16: , 347–370. |
[199] | Dusonchet J , Kochubey O , Stafa K , Young SM Jr. , Zufferey R , Moore DJ , Schneider BL , Aebischer P ((2011) ) A rat model of progressive nigral neurodegeneration induced by the Parkinson’s disease-associated G2019S mutation in LRRK2. J Neurosci 31: , 907–912. |
[200] | Greggio E , Jain S , Kingsbury A , Bandopadhyay R , Lewis P , Kaganovich A , van der Brug MP , Beilina A , Blackinton J , Thomas KJ , Ahmad R , Miller DW , Kesavapany S , Singleton A , Lees A , Harvey RJ , Harvey K , Cookson MR ((2006) ) Kinase activity is required for the toxic effects of mutant LRRK2/dardarin. Neurobiol Dis 23: , 329–341. |
[201] | Lee BD , Shin JH , VanKampen J , Petrucelli L , West AB , Ko HS , Lee YI , Maguire-Zeiss KA , Bowers WJ , Federoff HJ , Dawson VL , Dawson TM ((2010) ) Inhibitors of leucine-rich repeat kinase-2 protect against models of Parkinson’s disease. Nat Med 16: , 998–1000. |
[202] | Zhao Y , Keshiya S , Perera G , Schramko L , Halliday GM , Dzamko N ((2020) ) LRRK2 kinase inhibitors reduce alpha-synuclein in human neuronal cell lines with the G2019S mutation. Neurobiol Dis 144: , 105049. |
[203] | Beilina A , Rudenko IN , Kaganovich A , Civiero L , Chau H , Kalia SK , Kalia LV , Lobbestael E , Chia R , Ndukwe K , Ding J , Nalls MA ; International Parkinson’s Disease Genomics Consortium; North American Brain Expression Consortium, Olszewski M , Hauser DN , Kumaran R , Lozano AM , Baekelandt V , Greene LE , Taymans JM , Greggio E , Cookson MR ((2014) ) Unbiased screen for interactors of leucine-rich repeat kinase 2 supports a common pathway for sporadic and familial Parkinson disease. Proc Natl Acad Sci U S A 111: , 2626–2631. |
[204] | Chang D , Nalls MA , Hallgrímsdóttir IB , Hunkapiller J , van der Brug M , Cai F , Kerchner GA , Ayalon G , Bingol B , Sheng M , Hinds D , Behrens TW , Singleton AB , Bhangale TR , Graham RR ((2017) ) A meta-analysis of genome-wide association studies identifies 17 new Parkinson’s disease risk loci. Nat Genet 49: , 1511–1516. |
[205] | Cao M , Milosevic I , Giovedi S , De Camilli P ((2014) ) Upregulation of Parkin in endophilin mutant mice. J Neurosci 34: , 16544–16549. |
[206] | Shi M , Bradner J , Bammler TK , Eaton DL , Zhang J , Ye Z , Wilson AM , Montine TJ , Pan C , Zhang J ((2009) ) Identification of glutathione S-transferase pi as a protein involved in Parkinson disease progression. Am J Pathol 175: , 54–65. |
[207] | Schuske KR , Richmond JE , Matthies DS , Davis WS , Runz S , Rube DA , van der Bliek AM , Jorgensen EM ((2003) ) Endophilin is required for synaptic vesicle endocytosis by localizing synaptojanin. Neuron 40: , 749–762. |
[208] | Boucrot E , Ferreira AP , Almeida-Souza L , Debard S , Vallis Y , Howard G , Bertot L , Sauvonnet N , McMahon HT ((2015) ) Endophilin marks and controls a clathrin-independent endocytic pathway. Nature 517: , 460–465. |
[209] | Watanabe S , Mamer LE , Raychaudhuri S , Luvsanjav D , Eisen J , Trimbuch T , Sohl-Kielczynski B , Fenske P , Milosevic I , Rosenmund C , Jorgensen EM ((2018) ) Synaptojanin and endophilin mediate neck formation during ultrafast endocytosis. Neuron 98: , 1184–1197.e6. |
[210] | Gowrisankaran S , Houy S , Del Castillo JGP , Steubler V , Gelker M , Kroll J , Pinheiro PS , Schwitters D , Halbsgut N , Pechstein A , van Weering JRT , Maritzen T , Haucke V , Raimundo N , Sørensen JB , Milosevic I ((2020) ) Endophilin-A coordinates priming and fusion of neurosecretory vesicles via intersectin. Nat Commun 11: , 1266. |
[211] | Verstreken P , Kjaerulff O , Lloyd TE , Atkinson R , Zhou Y , Meinertzhagen IA , Bellen HJ ((2002) ) Endophilin mutations block clathrin-mediated endocytosis but not neurotransmitter release. Cell 109: , 101–112. |
[212] | Soukup SF , Kuenen S , Vanhauwaert R , Manetsberger J , Hernandez-Diaz S , Swerts J , Schoovaerts N , Vilain S , Gounko NV , Vints K , Geens A , De Strooper B , Verstreken P ((2016) ) A LRRK2-dependent endophilinA phosphoswitch is critical for macroautophagy at presynaptic terminals. Neuron 92: , 829–844. |
[213] | Murdoch JD , Rostosky CM , Gowrisankaran S , Arora AS , Soukup SF , Vidal R , Capece V , Freytag S , Fischer A , Verstreken P , Bonn S , Raimundo N , Milosevic I ((2016) ) Endophilin-A deficiency induces the Foxo3a-Fbxo32 network in the brain and causes dysregulation of autophagy and the ubiquitin-proteasome system. Cell Rep 17: , 1071–1086. |
[214] | Stenmark H ((2009) ) Rab GTPases as coordinators of vesicle traffic. Nat Rev Mol Cell Biol 10: , 513–525. |
[215] | Rivero-Ríos P , Gómez-Suaga P , Fernández B , Madero-Pérez J , Schwab Andrew J , Ebert Allison D , Hilfiker S ((2015) ) Alterations in late endocytic trafficking related to the pathobiology of LRRK2-linked Parkinson’s disease. Biochem Soc Trans 43: , 390–395. |
[216] | Homma Y , Hiragi S , Fukuda M ((2021) ) Rab family of small GTPases: An updated view on their regulation and functions. FEBS J 288: , 36–55. |
[217] | Wilson GR , Sim JC , McLean C , Giannandrea M , Galea CA , Riseley JR , Stephenson SE , Fitzpatrick E , Haas SA , Pope K , Hogan KJ , Gregg RG , Bromhead CJ , Wargowski DS , Lawrence CH , James PA , Churchyard A , Gao Y , Phelan DG , Gillies G , Salce N , Stanford L , Marsh AP , Mignogna ML , Hayflick SJ , Leventer RJ , Delatycki MB , Mellick GD , Kalscheuer VM , D’Adamo P , Bahlo M , Amor DJ , Lockhart PJ ((2014) ) Mutations in RAB39B cause X-linked intellectual disability and early-onset Parkinson disease with alpha-synuclein pathology. Am J Hum Genet 95: , 729–735. |
[218] | Mata IF , Jang Y , Kim CH , Hanna DS , Dorschner MO , Samii A , Agarwal P , Roberts JW , Klepitskaya O , Shprecher DR , Chung KA , Factor SA , Espay AJ , Revilla FJ , Higgins DS , Litvan I , Leverenz JB , Yearout D , Inca-Martinez M , Martinez E , Thompson TR , Cholerton BA , Hu SC , Edwards KL , Kim KS , Zabetian CP ((2015) ) The RAB39B p.G192R mutation causes X-linked dominant Parkinson’s disease. Mol Neurodegener 10: , 50. |
[219] | Gan-Or Z , Bar-Shira A , Dahary D , Mirelman A , Kedmi M , Gurevich T , Giladi N , Orr-Urtreger A ((2012) ) Association of sequence alterations in the putative promoter of RAB7L1 with a reduced parkinson disease risk. Arch Neurol 69: , 105–110. |
[220] | Cooper AA , Gitler AD , Cashikar A , Haynes CM , Hill KJ , Bhullar B , Liu K , Xu K , Strathearn KE , Liu F , Cao S , Caldwell KA , Caldwell GA , Marsischky G , Kolodner RD , Labaer J , Rochet JC , Bonini NM , Lindquist S ((2006) ) Alpha-synuclein blocks ER-Golgi traffic and Rab1 rescues neuron loss in Parkinson’s models. Science 313: , 324–328. |
[221] | Gitler AD , Bevis BJ , Shorter J , Strathearn KE , Hamamichi S , Su LJ , Caldwell KA , Caldwell GA , Rochet JC , McCaffery JM , Barlowe C , Lindquist S ((2008) ) The Parkinson’s disease protein alpha-synuclein disrupts cellular Rab homeostasis. Proc Natl Acad Sci U S A 105: , 145–150. |
[222] | Breda C , Nugent ML , Estranero JG , Kyriacou CP , Outeiro TF , Steinert JR , Giorgini F ((2015) ) Rab11 modulates α-synuclein-mediated defects in synaptic transmission and behaviour. Hum Mol Genet 24: , 1077–1091. |
[223] | Steger M , Tonelli F , Ito G , Davies P , Trost M , Vetter M , Wachter S , Lorentzen E , Duddy G , Wilson S , Baptista MA , Fiske BK , Fell MJ , Morrow JA , Reith AD , Alessi DR , Mann M ((2016) ) Phosphoproteomics reveals that Parkinson’s disease kinase LRRK2 regulates a subset of Rab GTPases. Elife 5: , e12813. |
[224] | Steger M , Diez F , Dhekne HS , Lis P , Nirujogi RS , Karayel O , Tonelli F , Martinez TN , Lorentzen E , Pfeffer SR , Alessi DR , Mann M ((2017) ) Systematic proteomic analysis of LRRK2-mediated Rab GTPase phosphorylation establishes a connection to ciliogenesis. Elife 6: , e31012. |
[225] | Madero-Pérez J , Fdez E , Fernández B , Lara Ordóñez AJ , Blanca Ramírez M , Gómez-Suaga P , Waschbüsch D , Lobbestael E , Baekelandt V , Nairn AC , Ruiz-Martínez J , Aiastui A , López de Munain A , Lis P , Comptdaer T , Taymans JM , Chartier-Harlin MC , Beilina A , Gonnelli A , Cookson MR , Greggio E , Hilfiker S ((2018) ) Parkinson disease-associated mutations in LRRK2 cause centrosomal defects via Rab8a phosphorylation. Mol Neurodegener 13: , 3. |
[226] | Fujimoto T , Kuwahara T , Eguchi T , Sakurai M , Komori T , Iwatsubo T ((2018) ) Parkinson’s disease-associated mutant LRRK2 phosphorylates Rab7L1 and modifies trans-Golgi morphology. Biochem Biophys Res Commun 495: , 1708–1715. |
[227] | Vilariño-Güell C , Wider C , Ross OA , Dachsel JC , Kachergus JM , Lincoln SJ , Soto-Ortolaza AI , Cobb SA , Wilhoite GJ , Bacon JA , Behrouz B , Melrose HL , Hentati E , Puschmann A , Evans DM , Conibear E , Wasserman WW , Aasly JO , Burkhard PR , Djaldetti R , Ghika J , Hentati F , Krygowska-Wajs A , Lynch T , Melamed E , Rajput A , Rajput AH , Solida A , Wu RM , Uitti RJ , Wszolek ZK , Vingerhoets F , Farrer MJ ((2011) ) VPS35 mutations in Parkinson disease. Am J Hum Genet 89: , 162–167. |
[228] | Seaman MN , Harbour ME , Tattersall D , Read E , Bright N ((2009) ) Membrane recruitment of the cargo-selective retromer subcomplex is catalysed by the small GTPase Rab7 and inhibited by the Rab-GAP TBC1D5. J Cell Sci 122: , 2371–2382. |
[229] | Priya A , Kalaidzidis IV , Kalaidzidis Y , Lambright D , Datta S ((2015) ) Molecular insights into Rab7-mediated endosomal recruitment of core retromer: Deciphering the role of Vps26 and Vps35. Traffic 16: , 68–84. |
[230] | Harrison MS , Hung CS , Liu TT , Christiano R , Walther TC , Burd CG ((2014) ) A mechanism for retromer endosomal coat complex assembly with cargo. Proc Natl Acad Sci U S A 111: , 267–272. |
[231] | Baba T , Toth DJ , Sengupta N , Kim YJ , Balla T ((2019) ) Phosphatidylinositol 4,5-bisphosphate controls Rab7 and PLEKHM1 membrane cycling during autophagosome-lysosome fusion. EMBO J 38: , e100312. |
[232] | Mizuno-Yamasaki E , Medkova M , Coleman J , Novick P ((2010) ) Phosphatidylinositol 4-phosphate controls both membrane recruitment and a regulatory switch of the Rab GEF Sec2p. Dev Cell 18: , 828–840. |
[233] | Jean S , Kiger AA ((2012) ) Coordination between RAB GTPase and phosphoinositide regulation and functions. Nat Rev Mol Cell Biol 13: , 463–470. |
[234] | Cauvin C , Rosendale M , Gupta-Rossi N , Rocancourt M , Larraufie P , Salomon R , Perrais D , Echard A ((2016) ) Rab35 GTPase triggers switch-like recruitment of the Lowe syndrome lipid phosphatase OCRL on newborn endosomes. Curr Biol 26: , 120–128. |
[235] | Klinkert K , Echard A ((2016) ) Rab35 GTPase: A central regulator of phosphoinositides and F-actin in endocytic recycling and beyond. Traffic 17: , 1063–1077. |
[236] | Shin HW , Hayashi M , Christoforidis S , Lacas-Gervais S , Hoepfner S , Wenk MR , Modregger J , Uttenweiler-Joseph S , Wilm M , Nystuen A , Frankel WN , Solimena M , De Camilli P , Zerial M ((2005) ) An enzymatic cascade of Rab5 effectors regulates phosphoinositide turnover in the endocytic pathway. J Cell Biol 170: , 607–618. |
[237] | Braccini L , Ciraolo E , Campa CC , Perino A , Longo DL , Tibolla G , Pregnolato M , Cao Y , Tassone B , Damilano F , Laffargue M , Calautti E , Falasca M , Norata GD , Backer JM , Hirsch E ((2015) ) PI3K-C2γ is a Rab5 effector selectively controlling endosomal Akt2 activation downstream of insulin signalling. Nat Commun 6: , 7400. |
[238] | Bohdanowicz M , Balkin DM , De Camilli P , Grinstein S ((2012) ) Recruitment of OCRL and Inpp5B to phagosomes by Rab5 and APPL1 depletes phosphoinositides and attenuates Akt signaling. Mol Biol Cell 23: , 176–187. |
[239] | Huotari J , Helenius A ((2011) ) Endosome maturation. EMBO J 30: , 3481–3500. |
[240] | Stein MP , Feng Y , Cooper KL , Welford AM , Wandinger-Ness A ((2003) ) Human VPS34 and p150 are Rab7 interacting partners. Traffic 4: , 754–771. |
[241] | Sun M , Luong G , Plastikwala F , Sun Y ((2020) ) Control of Rab7a activity and localization through endosomal type Igamma PIP 5-kinase is required for endosome maturation and lysosome function. FASEB J 34: , 2730–2748. |
[242] | Zimprich A , Benet-Pages A , Struhal W , Graf E , Eck SH , Offman MN , Haubenberger D , Spielberger S , Schulte EC , Lichtner P , Rossle SC , Klopp N , Wolf E , Seppi K , Pirker W , Presslauer S , Mollenhauer B , Katzenschlager R , Foki T , Hotzy C , Reinthaler E , Harutyunyan A , Kralovics R , Peters A , Zimprich F , Brucke T , Poewe W , Auff E , Trenkwalder C , Rost B , Ransmayr G , Winkelmann J , Meitinger T , Strom TM ((2011) ) A mutation in VPS35, encoding a subunit of the retromer complex, causes late-onset Parkinson disease. Am J Hum Genet 89: , 168–175. |
[243] | Cataldi S , Follett J , Fox JD , Tatarnikov I , Kadgien C , Gustavsson EK , Khinda J , Milnerwood AJ , Farrer MJ ((2018) ) Altered dopamine release and monoamine transporters in Vps35 p.D620N knock-in mice. NPJ Parkinsons Dis 4: , 27. |
[244] | Inoshita T , Arano T , Hosaka Y , Meng H , Umezaki Y , Kosugi S , Morimoto T , Koike M , Chang HY , Imai Y , Hattori N ((2017) ) Vps35 in cooperation with LRRK2 regulates synaptic vesicle endocytosis through the endosomal pathway in Drosophila. Hum Mol Genet 26: , 2933–2948. |
[245] | Muhammad A , Flores I , Zhang H , Yu R , Staniszewski A , Planel E , Herman M , Ho L , Kreber R , Honig LS , Ganetzky B , Duff K , Arancio O , Small SA ((2008) ) Retromer deficiency observed in Alzheimer’s disease causes hippocampal dysfunction, neurodegeneration, and Abeta accumulation. Proc Natl Acad Sci U S A 105: , 7327–7332. |
[246] | Seaman MN , McCaffery JM , Emr SD ((1998) ) A membrane coat complex essential for endosome-to-Golgi retrograde transport in yeast. J Cell Biol 142: , 665–681. |
[247] | Ye H , Ojelade SA , Li-Kroeger D , Zuo Z , Wang L , Li Y , Gu JY , Tepass U , Rodal AA , Bellen HJ , Shulman JM ((2020) ) Retromer subunit, VPS29, regulates synaptic transmission and is required for endolysosomal function in the aging brain. Elife 9: , e51977. |
[248] | Chen X , Kordich JK , Williams ET , Levine N , Cole-Strauss A , Marshall L , Labrie V , Ma J , Lipton JW , Moore DJ ((2019) ) Parkinson’s disease-linked D620N VPS35 knockin mice manifest tau neuropathology and dopaminergic neurodegeneration. Proc Natl Acad Sci U S A 116: , 5765–5774. |
[249] | Rojas R , van Vlijmen T , Mardones GA , Prabhu Y , Rojas AL , Mohammed S , Heck AJ , Raposo G , van der Sluijs P , Bonifacino JS ((2008) ) Regulation of retromer recruitment to endosomes by sequential action of Rab5 and Rab7. J Cell Biol 183: , 513–526. |
[250] | Martini-Stoica H , Xu Y , Ballabio A , Zheng H ((2016) ) The autophagy-lysosomal pathway in neurodegeneration: A TFEB perspective. Trends Neurosci 39: , 221–234. |
[251] | Menzies FM , Fleming A , Rubinsztein DC ((2015) ) Compromised autophagy and neurodegenerative diseases. Nat Rev Neurosci 16: , 345–357. |
[252] | Follett J , Norwood SJ , Hamilton NA , Mohan M , Kovtun O , Tay S , Zhe Y , Wood SA , Mellick GD , Silburn PA , Collins BM , Bugarcic A , Teasdale RD ((2014) ) The Vps35 D620N mutation linked to Parkinson’s disease disrupts the cargo sorting function of retromer. Traffic 15: , 230–244. |
[253] | Cui Y , Yang Z , Flores-Rodriguez N , Follett J , Ariotti N , Wall AA , Parton RG , Teasdale RD ((2021) ) Formation of retromer transport carriers is disrupted by the Parkinson disease-linked Vps35 D620N variant. Traffic 22: , 123–136. |
[254] | Zavodszky E , Seaman MN , Moreau K , Jimenez-Sanchez M , Breusegem SY , Harbour ME , Rubinsztein DC ((2014) ) Mutation in VPS35 associated with Parkinson’s disease impairs WASH complex association and inhibits autophagy. Nat Commun 5: , 3828. |
[255] | Pemberton JG , Kim YJ , Balla T ((2020) ) Integrated regulation of the phosphatidylinositol cycle and phosphoinositide-driven lipid transport at ER-PM contact sites. Traffic 21: , 200–219. |
[256] | Scorrano L , De Matteis MA , Emr S , Giordano F , Hajnóczky G , Kornmann B , Lackner LL , Levine TP , Pellegrini L , Reinisch K , Rizzuto R , Simmen T , Stenmark H , Ungermann C , Schuldiner M ((2019) ) Coming together to define membrane contact sites. Nat Commun 10: , 1287. |
[257] | Saheki Y , De Camilli P ((2017) ) Endoplasmic reticulum-plasma membrane contact sites. Annu Rev Biochem 86: , 659–684. |
[258] | Levin-Konigsberg R , Montaño-Rendón F , Keren-Kaplan T , Li R , Ego B , Mylvaganam S , DiCiccio JE , Trimble WS , Bassik MC , Bonifacino JS , Fairn GD , Grinstein S ((2019) ) Phagolysosome resolution requires contacts with the endoplasmic reticulum and phosphatidylinositol-4-phosphate signalling. Nat Cell Biol 21: , 1234–1247. |
[259] | Schütter M , Giavalisco P , Brodesser S , Graef M ((2020) ) Local fatty acid channeling into phospholipid synthesis drives phagophore expansion during autophagy. Cell 180: , 135–149.e114. |
[260] | Lesage S , Drouet V , Majounie E , Deramecourt V , Jacoupy M , Nicolas A , Cormier-Dequaire F , Hassoun SM , Pujol C , Ciura S , Erpapazoglou Z , Usenko T , Maurage CA , Sahbatou M , Liebau S , Ding J , Bilgic B , Emre M , Erginel-Unaltuna N , Guven G , Tison F , Tranchant C , Vidailhet M , Corvol JC , Krack P , Leutenegger AL , Nalls MA , Hernandez DG , Heutink P , Gibbs JR , Hardy J , Wood NW , Gasser T , Durr A , Deleuze JF , Tazir M , Destee A , Lohmann E , Kabashi E , Singleton A , Corti O , Brice A , French Parkinson’s Disease Genetics Study (PDG); International Parkinson’s Disease Genomics Consortium (IPDGC) ((2016) ) Loss of VPS13C function in autosomal-recessive parkinsonism causes mitochondrial dysfunction and increases PINK1/parkin-dependent mitophagy. Am J Hum Genet 98: , 500–513. |
[261] | Kumar N , Leonzino M , Hancock-Cerutti W , Horenkamp FA , Li P , Lees JA , Wheeler H , Reinisch KM , De Camilli P ((2018) ) VPS13A and VPS13C are lipid transport proteins differentially localized at ER contact sites. J Cell Biol 217: , 3625–3639. |
[262] | Paillusson S , Gomez-Suaga P , Stoica R , Little D , Gissen P , Devine MJ , Noble W , Hanger DP , Miller CCJ ((2017) ) alpha-Synuclein binds to the ER-mitochondria tethering protein VAPB to disrupt Ca(2+) homeostasis and mitochondrial ATP production. Acta Neuropathol 134: , 129–149. |
[263] | Jović M , Kean MJ , Szentpetery Z , Polevoy G , Gingras AC , Brill JA , Balla T ((2012) ) Two phosphatidylinositol 4-kinases control lysosomal delivery of the Gaucher disease enzyme, β-glucocerebrosidase. Mol Biol Cell 23: , 1533–1545. |
[264] | Clark LN , Ross BM , Wang Y , Mejia-Santana H , Harris J , Louis ED , Cote LJ , Andrews H , Fahn S , Waters C , Ford B , Frucht S , Ottman R , Marder K ((2007) ) Mutations in the glucocerebrosidase gene are associated with early-onset Parkinson disease. Neurology 69: , 1270–1277. |
[265] | Sidransky E , Samaddar T , Tayebi N ((2009) ) Mutations in GBA are associated with familial Parkinson disease susceptibility and age at onset. Neurology 73: , 1424–1425, author reply 1425-1426. |
[266] | Ben Romdhan S , Sakka S , Farhat N , Triki S , Dammak M , Mhiri C ((2018) ) A novel SYNJ1 mutation in a Tunisian family with juvenile Parkinson’s disease associated with epilepsy. J Mol Neurosci 66: , 273–278. |
[267] | Spillantini MG , Crowther RA , Jakes R , Hasegawa M , Goedert M ((1998) ) alpha-Synuclein in filamentous inclusions of Lewy bodies from Parkinson’s disease and dementia with lewy bodies. Proc Natl Acad Sci U S A 95: , 6469–6473. |
[268] | Massol RH , Boll W , Griffin AM , Kirchhausen T ((2006) ) A burst of auxilin recruitment determines the onset of clathrin-coated vesicle uncoating. Proc Natl Acad Sci U S A 103: , 10265–10270. |
[269] | Guan R , Dai H , Harrison SC , Kirchhausen T ((2010) ) Structure of the PTEN-like region of auxilin, a detector of clathrin-coated vesicle budding. Structure 18: , 1191–1198. |
[270] | He K , Song E , Upadhyayula S , Dang S , Gaudin R , Skillern W , Bu K , Capraro BR , Rapoport I , Kusters I , Ma M , Kirchhausen T ((2020) ) Dynamics of Auxilin 1 and GAK in clathrin-mediated traffic. J Cell Biol 219: , e201908142. |
[271] | Ungewickell E , Ungewickell H , Holstein SE , Lindner R , Prasad K , Barouch W , Martin B , Greene LE , Eisenberg E ((1995) ) Role of auxilin in uncoating clathrin-coated vesicles. Nature 378: , 632–635. |
[272] | Edvardson S , Cinnamon Y , Ta-Shma A , Shaag A , Yim YI , Zenvirt S , Jalas C , Lesage S , Brice A , Taraboulos A , Kaestner KH , Greene LE , Elpeleg O ((2012) ) A deleterious mutation in DNAJC6 encoding the neuronal-specific clathrin-uncoating co-chaperone auxilin, is associated with juvenile parkinsonism. PLoS One 7: , e36458. |
[273] | Olgiati S , Quadri M , Fang M , Rood JP , Saute JA , Chien HF , Bouwkamp CG , Graafland J , Minneboo M , Breedveld GJ , Zhang J , Verheijen FW , Boon AJ , Kievit AJ , Jardim LB , Mandemakers W , Barbosa ER , Rieder CR , Leenders KL , Wang J , Bonifati V ((2016) ) DNAJC6 mutations associated with early-onset Parkinson’s disease. Ann Neurol 79: , 244–256. |
[274] | Elsayed LE , Drouet V , Usenko T , Mohammed IN , Hamed AA , Elseed MA , Salih MA , Koko ME , Mohamed AY , Siddig RA , Elbashir MI , Ibrahim ME , Durr A , Stevanin G , Lesage S , Ahmed AE , Brice A ((2016) ) A novel nonsense mutation in DNAJC6 expands the phenotype of autosomal-recessive juvenile-onset Parkinson’s disease. Ann Neurol 79: , 335–337. |
[275] | Köroğlu Ç , Baysal L , Cetinkaya M , Karasoy H , Tolun A ((2013) ) DNAJC6 is responsible for juvenile parkinsonism with phenotypic variability. Parkinsonism Relat Disord 19: , 320–324. |
[276] | Xhabija B , Vacratsis PO ((2015) ) Receptor-mediated endocytosis 8 utilizes an N-terminal phosphoinositide-binding motif to regulate endosomal clathrin dynamics. J Biol Chem 290: , 21676–21689. |
[277] | Freeman CL , Hesketh G , Seaman MN ((2014) ) RME-8 coordinates the activity of the WASH complex with the function of the retromer SNX dimer to control endosomal tubulation. J Cell Sci 127: , 2053–2070. |
[278] | Shi A , Sun L , Banerjee R , Tobin M , Zhang Y , Grant BD ((2009) ) Regulation of endosomal clathrin and retromer-mediated endosome to Golgi retrograde transport by the J-domain protein RME-8. EMBO J 28: , 3290–3302. |
[279] | Popoff V , Mardones GA , Bai SK , Chambon V , Tenza D , Burgos PV , Shi A , Benaroch P , Urbe S , Lamaze C , Grant BD , Raposo G , Johannes L ((2009) ) Analysis of articulation between clathrin and retromer in retrograde sorting on early endosomes. Traffic 10: , 1868–1880. |
[280] | Norris A , Tammineni P , Wang S , Gerdes J , Murr A , Kwan KY , Cai Q , Grant BD ((2017) ) SNX-1 and RME-8 oppose the assembly of HGRS-1/ESCRT-0 degradative microdomains on endosomes. Proc Natl Acad Sci U S A 114: , E307–E316. |
[281] | Girard M , Poupon V , Blondeau F , McPherson PS ((2005) ) The DnaJ-domain protein RME-8 functions in endosomal trafficking. J Biol Chem 280: , 40135–40143. |
[282] | Vilariño-Güell C , Rajput A , Milnerwood AJ , Shah B , Szu-Tu C , Trinh J , Yu I , Encarnacion M , Munsie LN , Tapia L , Gustavsson EK , Chou P , Tatarnikov I , Evans DM , Pishotta FT , Volta M , Beccano-Kelly D , Thompson C , Lin MK , Sherman HE , Han HJ , Guenther BL , Wasserman WW , Bernard V , Ross CJ , Appel-Cresswell S , Stoessl AJ , Robinson CA , Dickson DW , Ross OA , Wszolek ZK , Aasly JO , Wu RM , Hentati F , Gibson RA , McPherson PS , Girard M , Rajput M , Rajput AH , Farrer MJ ((2014) ) DNAJC13 mutations in Parkinson disease. Hum Mol Genet 23: , 1794–1801. |
[283] | Holemans T , Sørensen DM , van Veen S , Martin S , Hermans D , Kemmer GC , Van den Haute C , Baekelandt V , Günther Pomorski T , Agostinis P , Wuytack F , Palmgren M , Eggermont J , Vangheluwe P ((2015) ) A lipid switch unlocks Parkinson’s disease-associated ATP13A2. Proc Natl Acad Sci U S A 112: , 9040–9045. |
[284] | Demirsoy S , Martin S , Motamedi S , van Veen S , Holemans T , Van den Haute C , Jordanova A , Baekelandt V , Vangheluwe P , Agostinis P ((2017) ) ATP13A2/PARK9 regulates endo-/lysosomal cargo sorting and proteostasis through a novel PI(3, 5)P2-mediated scaffolding function. Hum Mol Genet 26: , 1656–1669. |
[285] | van Veen S , Martin S , Van den Haute C , Benoy V , Lyons J , Vanhoutte R , Kahler JP , Decuypere JP , Gelders G , Lambie E , Zielich J , Swinnen JV , Annaert W , Agostinis P , Ghesquiere B , Verhelst S , Baekelandt V , Eggermont J , Vangheluwe P ((2020) ) ATP13A2 deficiency disrupts lysosomal polyamine export. Nature 578: , 419–424. |
[286] | Ramirez A , Heimbach A , Gründemann J , Stiller B , Hampshire D , Cid LP , Goebel I , Mubaidin AF , Wriekat AL , Roeper J , Al-Din A , Hillmer AM , Karsak M , Liss B , Woods CG , Behrens MI , Kubisch C ((2006) ) Hereditary parkinsonism with dementia is caused by mutations in ATP13A2, encoding a lysosomal type 5 P-type ATPase. Nat Genet 38: , 1184–1191. |
[287] | Rzepnikowska W , Flis K , Kaminska J , Grynberg M , Urbanek A , Ayscough KR , Zoladek T ((2017) ) Amino acid substitution equivalent to human chorea-acanthocytosis I2771R in yeast Vps13 protein affects its binding to phosphatidylinositol 3-phosphate. Hum Mol Genet 26: , 1497–1510. |
[288] | Lang AB , John Peter AT , Walter P , Kornmann B ((2015) ) ER-mitochondrial junctions can be bypassed by dominant mutations in the endosomal protein Vps13. J Cell Biol 210: , 883–890. |
[289] | Foo JN , Tan LC , Irwan ID , Au WL , Low HQ , Prakash KM , Ahmad-Annuar A , Bei J , Chan AY , Chen CM , Chen YC , Chung SJ , Deng H , Lim SY , Mok V , Pang H , Pei Z , Peng R , Shang HF , Song K , Tan AH , Wu YR , Aung T , Cheng CY , Chew FT , Chew SH , Chong SA , Ebstein RP , Lee J , Saw SM , Seow A , Subramaniam M , Tai ES , Vithana EN , Wong TY , Heng KK , Meah WY , Khor CC , Liu H , Zhang F , Liu J , Tan EK ((2017) ) Genome-wide association study of Parkinson’s disease in East Asians. Hum Mol Genet 26: , 226–232. |
[290] | Schormair B , Kemlink D , Mollenhauer B , Fiala O , Machetanz G , Roth J , Berutti R , Strom TM , Haslinger B , Trenkwalder C , Zahorakova D , Martasek P , Ruzicka E , Winkelmann J ((2018) ) Diagnostic exome sequencing in early-onset Parkinson’s disease confirms VPS13C as a rare cause of autosomal-recessive Parkinson’s disease. Clin Genet 93: , 603–612. |
[291] | Darvish H , Bravo P , Tafakhori A , Azcona LJ , Ranji-Burachaloo S , Johari AH , Paisán-Ruiz C ((2018) ) Identification of a large homozygous VPS13C deletion in a patient with early-onset Parkinsonism. Mov Disord 33: , 1968–1970. |
[292] | Mufti K , Rudakou U , Yu E , Krohn L , Ruskey JA , Asayesh F , Laurent SB , Spiegelman D , Arnulf I , Hu MTM , Montplaisir JY , Gagnon JF , Desautels A , Dauvilliers Y , Gigli GL , Valente M , Janes F , Högl B , Stefani A , Holzknecht E , Šonka K , Kemlink D , Oertel W , Janzen A , Plazzi G , Antelmi E , Figorilli M , Puligheddu M , Mollenhauer B , Trenkwalder C , Sixel-Döring F , Cochen De Cock V , Monaca CC , Heidbreder A , Ferini-Strambi L , Dijkstra F , Viaene M , Abril B , Boeve BF , Postuma RB , Rouleau GA , Gan-Or Z ((2020) ) Comprehensive analysis of familial parkinsonism genes in rapid-eye-movement sleep behavior disorder. Mov Disord 36: , 235–240. |
[293] | Sbrissa D , Ikonomov OC , Fenner H , Shisheva A ((2008) ) ArPIKfyve homomeric and heteromeric interactions scaffold PIKfyve and Sac3 in a complex to promote PIKfyve activity and functionality. J Mol Biol 384: , 766–779. |
[294] | Zhang Y , Zolov SN , Chow CY , Slutsky SG , Richardson SC , Piper RC , Yang B , Nau JJ , Westrick RJ , Morrison SJ , Meisler MH , Weisman LS ((2007) ) Loss of Vac14, a regulator of the signaling lipid phosphatidylinositol 3,5-bisphosphate, results in neurodegeneration in mice. Proc Natl Acad Sci U S A 104: , 17518–17523. |
[295] | Schulze U , Vollenbröker B , Braun DA , Van Le T , Granado D , Kremerskothen J , Fränzel B , Klosowski R , Barth J , Fufezan C , Wolters DA , Pavenstädt H , Weide T ((2014) ) The Vac14-interaction network is linked to regulators of the endolysosomal and autophagic pathway. Mol Cell Proteomics 13: , 1397–1411. |
[296] | Stutterd C , Diakumis P , Bahlo M , Fanjul Fernandez M , Leventer RJ , Delatycki M , Amor D , Chow CW , Stephenson S , Meisler MH , McLean C , Lockhart PJ ((2017) ) Neuropathology of childhood-onset basal ganglia degeneration caused by mutation of VAC14. Ann Clin Transl Neurol 4: , 859–864. |
[297] | Shin N , Jeong H , Kwon J , Heo HY , Kwon JJ , Yun HJ , Kim CH , Han BS , Tong Y , Shen J , Hatano T , Hattori N , Kim KS , Chang S , Seol W ((2008) ) LRRK2 regulates synaptic vesicle endocytosis. Exp Cell Res 314: , 2055–2065. |
[298] | Connor-RobsonN, BoothH, MartinJG, GaoB, LiK, DoigN, VowlesJ, BrowneC, KlingerL, JuhaszP, KleinC, CowleySA, BolamP, HirstW, Wade-MartinsR ((2019) ) An integrated transcriptomics and proteomics analysis reveals functional endocytic dysregulation caused by mutations in LRRK2. Neurobiol Dis 127: , 512–526. |
[299] | Renard HF , Simunovic M , Lemiere J , Boucrot E , Garcia-Castillo MD , Arumugam S , Chambon V , Lamaze C , Wunder C , Kenworthy AK , Schmidt AA , McMahon HT , Sykes C , Bassereau P , Johannes L ((2015) ) Endophilin-A2 functions in membrane scission in clathrin-independent endocytosis. Nature 517: , 493–496. |
[300] | Furlong RM , Lindsay A , Anderson KE , Hawkins PT , Sullivan AM , O’Neill C ((2019) ) The Parkinson’s disease gene PINK1 activates Akt via PINK1 kinase-dependent regulation of the phospholipid PI(3,4,5)P(3). J Cell Sci 132: , jcs233221. |
[301] | Ge P , Dawson VL , Dawson TM ((2020) ) PINK1 and Parkin mitochondrial quality control: A source of regional vulnerability in Parkinson’s disease. Mol Neurodegener 15: , 20. |
[302] | Valente EM , Abou-Sleiman PM , Caputo V , Muqit MM , Harvey K , Gispert S , Ali Z , Del Turco D , Bentivoglio AR , Healy DG , Albanese A , Nussbaum R , González-Maldonado R , Deller T , Salvi S , Cortelli P , Gilks WP , Latchman DS , Harvey RJ , Dallapiccola B , Auburger G , Wood NW ((2004) ) Hereditary early-onset Parkinson’s disease caused by mutations in PINK1. Science 304: , 1158–1160. |
[303] | Kasten M , Hartmann C , Hampf J , Schaake S , Westenberger A , Vollstedt EJ , Balck A , Domingo A , Vulinovic F , Dulovic M , Zorn I , Madoev H , Zehnle H , Lembeck CM , Schawe L , Reginold J , Huang J , König IR , Bertram L , Marras C , Lohmann K , Lill CM , Klein C ((2018) ) Genotype-phenotype relations for the Parkinson’s disease genes parkin, PINK1, DJ1: MDSGene systematic review. Mov Disord 33: , 730–741. |
[304] | Yang Y , Gehrke S , Haque ME , Imai Y , Kosek J , Yang L , Beal MF , Nishimura I , Wakamatsu K , Ito S , Takahashi R , Lu B ((2005) ) Inactivation of Drosophila DJ-1 leads to impairments of oxidative stress response and phosphatidylinositol 3-kinase/Akt signaling. Proc Natl Acad Sci U S A 102: , 13670–13675. |
[305] | Wang X , Petrie TG , Liu Y , Liu J , Fujioka H , Zhu X ((2012) ) Parkinson’s disease-associated DJ-1 mutations impair mitochondrial dynamics and cause mitochondrial dysfunction. J Neurochem 121: , 830–839. |
[306] | Strobbe D , Robinson AA , Harvey K , Rossi L , Ferraina C , de Biase V , Rodolfo C , Harvey RJ , Campanella M ((2018) ) Distinct mechanisms of pathogenic DJ-1 mutations in mitochondrial quality control. Front Mol Neurosci 11: , 68. |
[307] | Canet-Aviles RM , Wilson MA , Miller DW , Ahmad R , McLendon C , Bandyopadhyay S , Baptista MJ , Ringe D , Petsko GA , Cookson MR ((2004) ) The Parkinson’s disease protein DJ-1 is neuroprotective due to cysteine-sulfinic acid-driven mitochondrial localization. Proc Natl Acad Sci U S A 101: , 9103–9108. |
[308] | Bonifati V , Rizzu P , van Baren MJ , Schaap O , Breedveld GJ , Krieger E , Dekker MC , Squitieri F , Ibanez P , Joosse M , van Dongen JW , Vanacore N , van Swieten JC , Brice A , Meco G , van Duijn CM , Oostra BA , Heutink P ((2003) ) Mutations in the DJ-1 gene associated with autosomal recessive early-onset parkinsonism. Science 299: , 256–259. |
[309] | Carlton J , Bujny M , Peter BJ , Oorschot VM , Rutherford A , Mellor H , Klumperman J , McMahon HT , Cullen PJ ((2004) ) Sorting nexin-1 mediates tubular endosome-to-TGN transport through coincidence sensing of high- curvature membranes and 3-phosphoinositides. Curr Biol 14: , 1791–1800. |
[310] | Zhou F , Zou S , Chen Y , Lipatova Z , Sun D , Zhu X , Li R , Wu Z , You W , Cong X , Zhou Y , Xie Z , Gyurkovska V , Liu Y , Li Q , Li W , Cheng J , Liang Y , Segev N ((2017) ) A Rab5 GTPase module is important for autophagosome closure. PLoS Genet 13: , e1007020. |
[311] | Hammerling BC , Najor RH , Cortez MQ , Shires SE , Leon LJ , Gonzalez ER , Boassa D , Phan S , Thor A , Jimenez RE , Li H , Kitsis RN , Dorn GW II , Sadoshima J , Ellisman MH , Gustafsson Å B ((2017) ) A Rab5 endosomal pathway mediates Parkin-dependent mitochondrial clearance. Nat Commun 8: , 14050. |