Superconductivity in null-scattering Ti:Zr alloy
Abstract
Null-scattering Ti:Zr alloy (Ti67.6Zr32.4) is widely used in neutron scattering for sample containers, pressure cells and gaskets since it does not produce contaminant Bragg reflections from the sample environment. Here we report superconductivity in this material with critical temperature
1.Introduction
Titanium and zirconium crystallize at 300 K both in a hexagonal close-packed structure and the two elements are fully miscible. In 1956, Sidhu et al. [8] have found that the negative and positive scattering amplitudes of Ti and Zr could be exploited to cancel the coherent scattering length of a Ti:Zr alloy containing 62 atomic% of Ti and 38 atomic% of Zr. From scattering lengths published in 1992 [7], with 52.2 mass% Ti and 47.8 mass% Zr; i.e., 67.6 mole% Ti and 32.4 mole% Zr, Ti67.6Zr32.4 is “null scattering”, i.e., does not produce Bragg reflections in neutron diffraction (the term “null-scattering” is somewhat misleading since the material indeed scatters neutrons, but only incoherently, resulting in a diffuse “background” with a weak Q-dependence). This alloy has relatively high neutron transparency, good mechanical properties, and is easily machinable provided there is a continuous supply of coolant to prevent self-ignition [1]. For all these reasons it is widely used for sample containers, in particular those with thick walls such as high-pressure cells [2]. It is also extensively used as gasket material in opposed-anvil pressure devices such as the Paris-Edinburgh cell [5], and is often simply referred to as “titanium-zirconium” (“Ti:Zr”) in the neutron community.
Whereas the mechanical properties of Ti:Zr have been investigated in much detail [5], both at high and low temperatures, its magnetic properties appear to be rather unknown. Both Ti and Zr are superconducting with critical temperatures of 0.49 K and 0.61 K, respectively, and it appears hence likely that Ti:Zr is superconducting as well. Indeed, it was demonstrated that the critical temperature of this alloy has a maximum of 1.5 K at approximately equimolar composition [4]. But so far, no further details have been published, for example values of the critical fields are unknown. More specifically, no data on the null-scattering alloy Ti:Zr are known at all, as far as we are aware of.
Here we present magnetization measurements on Ti:Zr showing that it is superconducting and giving properties such as lower and upper critical fields and the influence of a minor amount of iron in ingots of different producers. These result bear on the use of null-scattering Ti:Zr for sample containers in neutron scattering experiments under applied field.
2.Experimental details
Magnetization measurements were carried out on five different samples from at least two different providers. The first sample was cut off from a bar of 30 mm diameter provided to the ILL in 1988. The producer is believed to be UGITEC (Ugine, France). The second sample was cut-off a toroidal gasket made for use in a Paris-Edinburgh cell. The producer is either IMI Titanium Ltd. (Birmingham, U.K.) or TIMET (Henderson, USA). The three other samples are all from the TIMET Henderson Technical Laboratory, an entity working with the UGITEC research center. They were cut-off from 3 different billets of ∼20 cm (8 inch) diameter purchased in 2010 and 2019. A significant difference in these samples is the amount of a small ferromagnetic component as revealed by magnetization measurements at 300 K. X-ray fluorescence measurements using a Mo-source (
The magnetic measurements were performed with a SQUID-VSM magnetometer (Quantum Design, USA) with a 3He option. The sample with a mass of 308.4 mg (58.3 mm3) had an ellipsoidal shape with approximately 8 mm hight and a 3.3 mm width. It was mounted in a plastic straw and suspended on a sample rod in the cold gas above the 3He bath. At each measurement the sample scans through detection coils (over a length of 25 mm) and the measured signal is fitted to the theoretical response function for a small magnetic dipole from which the magnetization is determined.
3.Results and discussion
Figure 1 shows susceptibility data as a function of temperature, at three different applied fields. In all cases onset of superconductivity is found at temperatures slightly above 1.4 K and full field expulsion (Meissner effect, susceptibility reaches −1 in SI system) is seen below 1 K. Figure 2 displays a full magnetization versus field cycle recorded at 0.42 K and starting at zero field. It is seen that Ti:Zr is a type-II superconductor with a lower critical field Hc1 of approximately 100 Oe, i.e., 0.01 Tesla, if we define Hc1 as the field where the M(H) relation starts to deviate from being linear. Above this field, in the Shubnikov phase, flux-lines enter the sample and reduce the magnetization. The upper critical field is approximately 4500 Oe (0.45 Tesla), above which the magnetization is zero and the sample is fully normal-conducting.
We have repeated the same measurements with samples from other providers, i.e., those with the higher ferromagnetic impurities (85 and 25 ppm, see above). The critical temperatures are found to be exactly the same in both samples, but in the first specimen the lower and upper critical fields are somewhat higher (i.e., approximately 120 Oe and 6000 Oe, respectively). This leads naturally to a smaller field dependence of the susceptibility as a function of temperature (Fig. 1). Whilst this reflects most likely an increased flux-pinning, it may not be related to the ferromagnetic impurity but rather on other factors, such as a different microstructure due to a different thermal or mechanical treatment.
Apart from the academic value of our data related to superconductivity, these findings have some practical importance for neutron scattering experiments which investigate materials under applied field and using Ti:Zr as a sample container. Typical examples are chiral magnets like MnSi where skyrmions and spirals are investigated with polarized beams under hydrostatic pressure [3,6]. An investigation of the pressure dependence of such phases below 1.4 K would be seriously hampered if the pressure cell is made of Ti:Zr, at least for applied field below 0.45 T.
Fig. 1.
Meissner effect in Ti:Zr, at three different applied fields. Note that in SI units, a susceptibility value of −1 corresponds to full field expulsion.
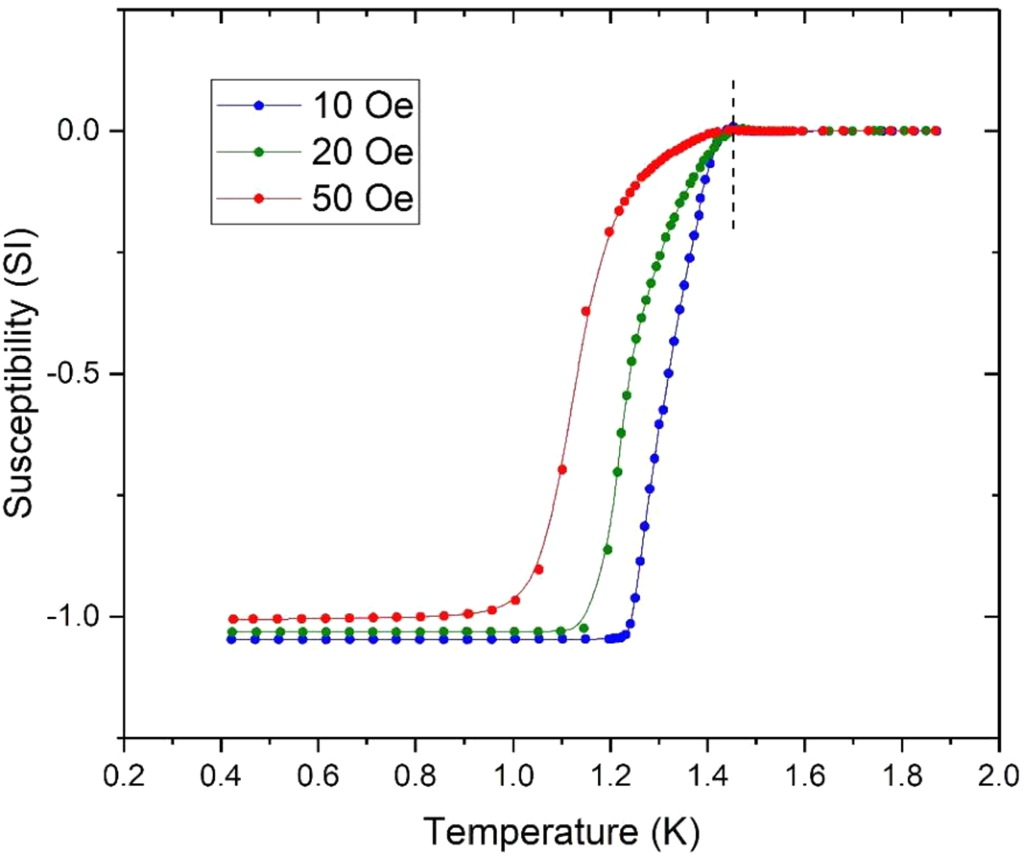
Fig. 2.
Magnetization M versus field H at 0.42 K, over a full M-H cycle. The inset shows an enlarged view of the low-field region. The red line corresponds to a susceptibility
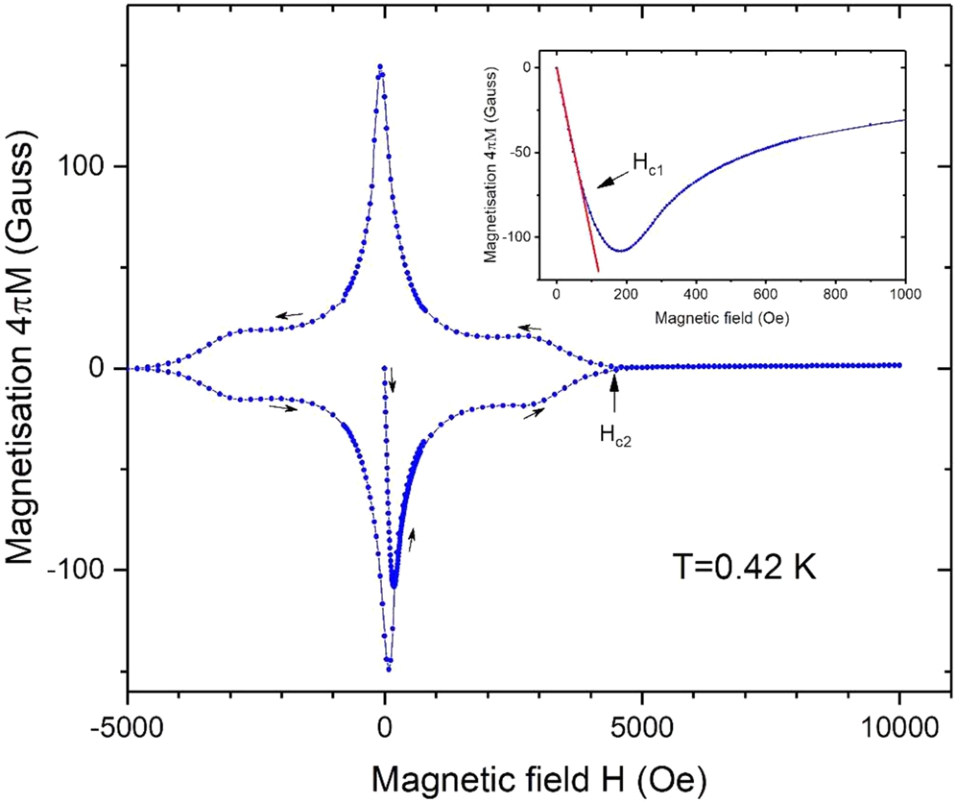
References
[1] | |
[2] | https://www.ill.eu/users/support-labs-infrastructure/sample-environment/equipment/high-pressures. |
[3] | L.J. Bannenberg, R. Sadykov, R.M. Dalgliesh et al., Phys. Rev. B 100: ((2019) ), 054447. doi:10.1103/PhysRevB.100.054447. |
[4] | J.K. Hulm and R.D. Blaugher, Phys. Rev. 123: ((1961) ), 1569–1580. doi:10.1234/JN-2005-102-203. |
[5] | S. Klotz, Techniques in High Pressure Neutron Scattering, CRC Press, Taylor and Francis, Boca Raton, (2013) . |
[6] | R. Sadykov, C. Pappas, L.J. Bannenberg et al., J. Neutron Res. 20: ((2018) ), 25–33. doi:10.3233/JNR-180056. |
[7] | V.F. Sears, Neutron News 3: ((1992) ), 26–37. doi:10.1080/10448639208218770. |
[8] | S.S. Sidhu, L. Heaton, D.D. Zauberis and F.P. Campos, J. Appl. Phys. 27: ((1956) ), 1040–1042. doi:10.1063/1.1722538. |