Induction of Skeletal Muscle Progenitors and Stem Cells from human induced Pluripotent Stem Cells
Abstract
Induced pluripotent stem cells (iPSCs) have the potential to differentiate into various types of cells and tissues including skeletal muscle. The approach to convert these stem cells into skeletal muscle cells offers hope for patients afflicted with skeletal muscle diseases such as Duchenne muscular dystrophy (DMD). Several methods have been reported to induce myogenic differentiation with iPSCs derived from myogenic patients. An important point for generating skeletal muscle cells from iPSCs is to understand in vivo myogenic induction in development and regeneration. Current protocols of myogenic induction utilize techniques with overexpression of myogenic transcription factors such as Myod1(MyoD), Pax3, Pax7, and others, using recombinant proteins or small molecules to induce mesodermal cells followed by myogenic progenitors, and adult muscle stem cells. This review summarizes the current approaches used for myogenic induction and highlights recent improvements.
INTRODUCTION
Skeletal muscle is known to be a highly regenerative tissue in our body. If we have muscle damage, regeneration is mediated by skeletal muscle stem cells (MuSCs), known as satellite cells on myofibers because of their peripheral location [1]. These cells are destined to become skeletal muscle cells and fuse into damaged myofibers during regeneration, although MuSCs can exhibit some multipotential differentiation capabilities [2–5]. Expanded MuSCs in culture will integrate into regenerating myofibers following transplantation into damaged muscle, thus providing cell transplantation therapy for muscular dystrophies [6]. However, cell therapy for human muscular diseases has severe limitations, since only a small number of usable MuSCs are present in biopsies. In addition, poor cell survival and contribution of transplanted myogenic cells have retarded practical application in patients with muscle disease [7, 8].
The discovery of mouse or human iPSCs has marked a milestone in the field of biomedical sciences. These cells are genetically reprogrammed to the state of embryonic stem (ES) cells by forcing the activation of 4 transcription factors, Oct3/4, Sox2, c-Myc, and Klf4, which together maintain the properties of pluripotent stem cells [9, 10]. Human iPS cells (hiPSCs) can be generated from a wide variety of somatic cells, and have the ability to self-renew and be directed into various cell types. hiPSCs represent an attractive cell source for producing myogenic cells, with their ability to capture genetic diversity of DMD, or to permit development of a new pharmaceutical in an accessible cell culture system. The differentiation from ES or iPS cells follows multistep processes of embryonic development. Skeletal muscle progenitors originate from the mesodermal lineage, which gives rise to not only skeletal muscle, but also cardiac muscle, bone, connective tissue, blood cells and kidney. All myogenic progenitors in the trunk and limbs, derive from the paraxial mesoderm, from somites which are segmented compartments that form from the anterior to posterior axis of the embryo. Cells in the epithelial structure of the dorsal somite, the dermomyotome, express the paired box transcription factors Pax3 and its paralog Pax7, and can give rise to a number of different tissues such as dermis, skeletal muscle, endothelial and vascular smooth muscle cells [11]. The dermomyotome also serves as a structure for receiving and transmitting secreted signaling molecules [12]. Upon signals from the neural tube and notochord, a part of the dermomyotome initiates the expression of skeletal muscle-specific transcription factors (myogenic regulatory factors), MyoD, Myf5, Myogenin, and Myf6 (MRF4) for differentiating into myogenic cells termed myoblasts. Such myoblasts eventually fuse with each other to form embryonic myofibers [13]. These differentiation steps have been replicated to induce skeletal muscle cells for research with hiPSCs [14]. Many studies have described induction methods to overexpress myogenic transcription factors such as MyoD, Pax3/7, and others [15, 16], with the addition of recombinant proteins, or small molecules which activate or inhibit myogenic signaling during myogenesis and muscle regeneration.
Myogenic transcription factors for skeletal muscle development
The differentiation process of myogenic cells is regulated by core transcription factors that govern the formation of multinucleated myotubes and the establishment of quiescent MuSCs. Developing myogenic progenitor cells initiate the expression of Pax3 and Pax7 in the dermomyotome [17], and go on to express the myogenic regulatory factors Myf5 and MyoD in the dorsal and lateral lips of the dermomyotome. They then migrate beneath the dermomyotome to form the myotome after undergoing an epithelial-mesenchymal transition. Pax3 can directly regulate Myf5 expression to induce the myotome and other muscles that form from migrating myogenic cells [18, 19]. Subsequently, other myogenic regulatory factors, MRF4 and Myogenin, followed by structural proteins that characterize skeletal muscle cells such as myosin heavy chain (MyHC), become detectable in the myotome as it undergoes terminal differentiation [20, 21] (Fig. 1).
Fig. 1
Schematic representation of vertebrate myogenesis as it occurs in mouse embryos. Myotomes are formed and mature following a rostrocaudal gradient on either side of the axial structures (from A to D). NT, neural tube; NC, neural crest; No, notochord.
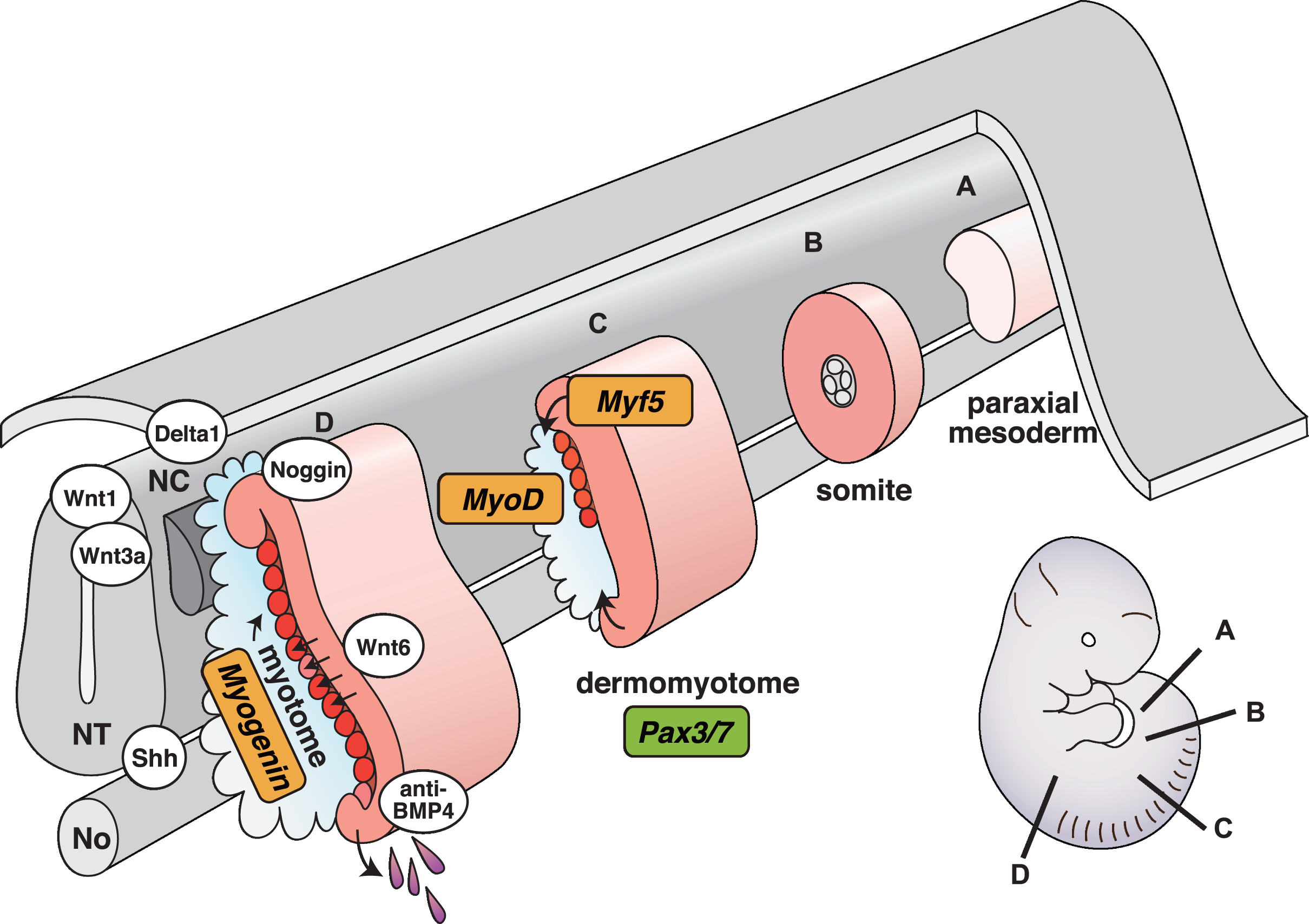
Secreted Wnt proteins derived from the dorsal neural tube, and sonic hedgehog (Shh) from the floor plate of the neural tube and notochord positively regulate myotome formation [12, 22]. Neural crest cells migrating from the dorsal neural tube are also involved in myotome formation. Migrating neural crest cells traverse the dorsomedial lip of the dermomyotome, and transiently activate Notch signaling in the dermomyotome, resulting in conversion of Pax3 + Pax7 + myogenic progenitors into MyoD + myoblasts [23, 24]. By contrast, bone morphogenetic proteins (BMPs) secreted from lateral plate mesoderm act as negative regulators of myotome formation by preventing myogenic factor expression, thus maintaining myogenic progenitors [22, 25]. Pax3 promotes myogenic progenitor cell survival and also regulates cell migration of myogenic progenitor cells from the ventrolateral lip of the dermomyotome to the limb bud because Pax3 mutant mice show severe limb muscle defects whereas trunk muscle development is relatively normal [26, 27]. Pax3 and Pax7 double knockout mutant mice display loss of myogenic cells throughout the trunk, suggesting that both factors are critical for initial embryonic myogenesis. They are considered as master regulators for the specification of myogenic progenitor cells in the body [28] (Fig. 1).
Importantly, MyoD was initially reported as the master regulator for myogenic specification because MyoD can directly reprogram non-muscle cells into the myogenic lineage by simply overexpression [29–31]. In addition, these myogenic regulatory factors are essential for the formation of skeletal muscle because the genetic ablation of members of the MyoD family in mice has been shown to cause severe myogenic developmental or regeneration defects [32–38].
Overexpression approaches with myogenic transcription factors
It has been initially described in 1992 [39], that overexpression of MyoD can induce myogenic cells from mouse ES. This method has been adopted as a standard approach for myogenic induction from pluripotent stem cells [40–42]. Myogenic cells induced from hiPSCs derived from patients should be able to provide the opportunity for therapeutic drug screening by this approach [43]. However the simple overexpression of MyoD in hES cells can induce not only myogenic but some paraxial mesodermal genes. Co-overexpression of MyoD and Smarcd3 (BAF60C), which is involved in chromatin remodeling, was then shown to induce the myogenic program, with lower expression of paraxial mesoderm genes [44]. Paraxial mesodermal gene expression induced by MyoD was artificially mediated by direct MyoD binding to their regulatory regions, indicating a novel role for MyoD in paraxial mesoderm cell induction [45]. It has been reported that transient expression of histone demethylase Kdm6b (Jmjd3), which reduces H3K27me, together with MyoD, can accelerate myogenic differentiation from hiPSCs [46]. hiPSCs have been used as a source for cell transplantation and subsequent differentiation into myofibers by overexpression of MyoD [47, 48]. This method is also now considered as an excellent in vitro model, and used to replicate human skeletal muscle diseases, for muscle functional tests, therapeutic drug screening, and genetically engineered genome-editing by exon skipping and endonucleases using CRISPR/Cas9 (Fig. 2). DMD patient-derived hiPSCs, when they undergo myogenic differentiation mediated by MyoD overexpression with a PiggyBac transposon, showed muscle functional improvement after exon skipping treatment [49], or genome-editing with TALEN and CRISPR/Cas9 systems [50]. There are some reports regarding skeletal muscle tissue engineering technologies using human pluripotent stem cells. To generate functional and contractile myotubes, 3D culture with a fibrin-based hydrogel as scaffold has been used and resulted in Ca2+ transients and vascularization in a transplanted host mouse after grafting of induced myobundles [51]. Furthermore the co-culture of MyoD induced skeletal myogenic cells with vascular endothelial cells, pericytes and motor neurons mimicked a 3D artificial skeletal muscle [52].
Fig. 2
The expression cascade of pivotal transcription factors for myogenesis. As shown in Fig. 1, segmented somites are formed. Pax3 and Pax7 are activated in the dermomyotome (Pax3 + Pax7 + MyoD-). They work as master regulators for the induction of myogenic progenitors. Myogenic regulatory factors are next upregulated in both lips of the dermomyotome and function as master regulators for myogenic specification to generate myoblasts (MyoD + Pax3/7±). Eventually, myoblasts stop cell proliferation and express Myogenin (myogenic differentiation), which induces terminal differentiation of myoblasts to form multinucleated myofibers. Adult muscle stem cells which are marked by the presence of Pax7 or Pax7 and Pax3, but not MyoD, are derived from the MyoD/Myf5-primed myogenic lineage, contribute to muscle regeneration.
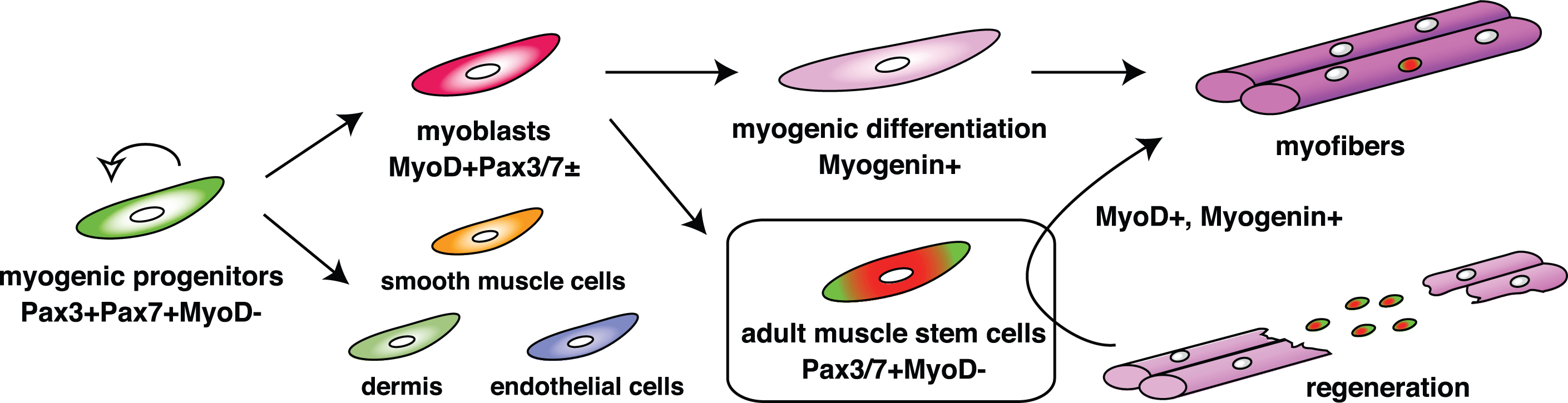
Besides the strategy with MyoD overexpression, activation of Pax3 by inducible treatment with doxycycline in mouse ES cells, can induce MyoD + Myf5+ myoblasts in embryoid body (EB) cultures [15]. These myogenic cells differentiated into MyHC+ myotubes, however, teratoma formation was observed after EB cell transplantation into regenerating myofibers of immuno deficient mice. This indicates that induced cells accelerated by Pax3 in bulk ES cell cultures still contain undifferentiated cells which caused teratomas. To overcome this problem, paraxial mesoderm cells from Pax3-induced EB cell cultures were collected by cell sorting, using antibodies against cell surface markers to isolate PDGFRα+ Kdr(Flk1)- cell populations [53]. These isolated paraxial mesodermal cells induced with Pax3 successfully contributed to regenerating muscle in DMD model mice with no teratoma formation. Both Pax3 and Pax7 can successfully control myogenic induction in hiPSCs [53, 54]. Both genes are expressed in not only myogenic progenitor cells, but also in the dorsal neural tube and in neural crest cells which also contribute to the developing heart, suggesting that further purification of the skeletal muscle cell lineage is crucial for therapeutic applications for muscle diseases including DMD. However, the overexpression of pivotal myogenic transcription factors such as MyoD or Pax3/7 has been considered as the major strategy for myogenic induction in non-skeletal muscle cells.
Myogenic stem cell induced with multiple myogenic transcription factors
Exogenous expression of MyoD exerts anti-proliferative actions and cannot indefinitely maintain the cell cycle state of proliferating skeletal muscle progenitors or stem cells in vitro [55]. Thereforeinduced cells that are simply overexpressing MyoD are not a suitable source for a number of therapeutic applications, including cell therapy with transplantation, although exogenous expression of MyoD can induce differentiated myocytes or myofibers [56]. Because transcription factors related to myogenic differentiation, such as Myogenin, might inhibit the induction of skeletal muscle progenitors or stem cells, to induce an undifferentiated subpopulation with stemness from the heterogeneous MuSC population. It was necessary to identify proper transcription factors essential for inducing myogenic progenitors or stem cells [56–59].
The transcription factors expressed in quiescent mouse muscle stem cells have been reported [60, 61], and combinations of inducible transcription factors have been identified, either Pax3/7, Mef2b, and Pitx1, for the induction of M-cadherin + skeletal muscle progenitor cells from mouse embryonic fibroblasts, or Pax7, Mef2b and MyoD for adult dermal fibroblasts, by directly reprogramming techniques [62]. Moreover, Pax3-expressing muscle progenitor or stem cells were generated most efficiently by a mixture of eight transcription factors, Egr2, HeyL, Klf4, Mef2c, MyoD, Pax3, Pax7, and Pitx2. Furthermore, it has been shown that four transcription factors, Pax3, HeyL, Klf4, and transient MyoD enhance the derivation of Pax3+ myogenic progenitors from not only fibroblasts but also hiPSCs, using medium that promotes the formation of presomitic mesoderm [63]. In this situation MyoD protein could not be detected as reported for adult quiescent MuSCs [64, 65]. Therefore the important point is to control the expression level of MyoD. As previously reported, exogenous expression of MyoD alone was not sufficient to induce myogenic progenitors or stem cells. Because overexpression of MyoD exerts cell-cycle arrest effects, the regulation of MyoD activation to generate proliferating muscle progenitors or stem cells seems to be critical. The combination of Pax3/7, other factors, and exposure to small molecules, might inhibit the anti-proliferating effects of MyoD as proliferating MuSCs or differentiating myoblasts start to express endogenous MyoD [62, 63, 66] (Fig. 3).
Fig. 3
Schematic summary of an induction strategy for muscle stem cells (Pax3 + Pax7+ cells) or myoblasts (MRFs+ cells) from non-muscle fibroblasts or iPS cells, with defined transcription factors.
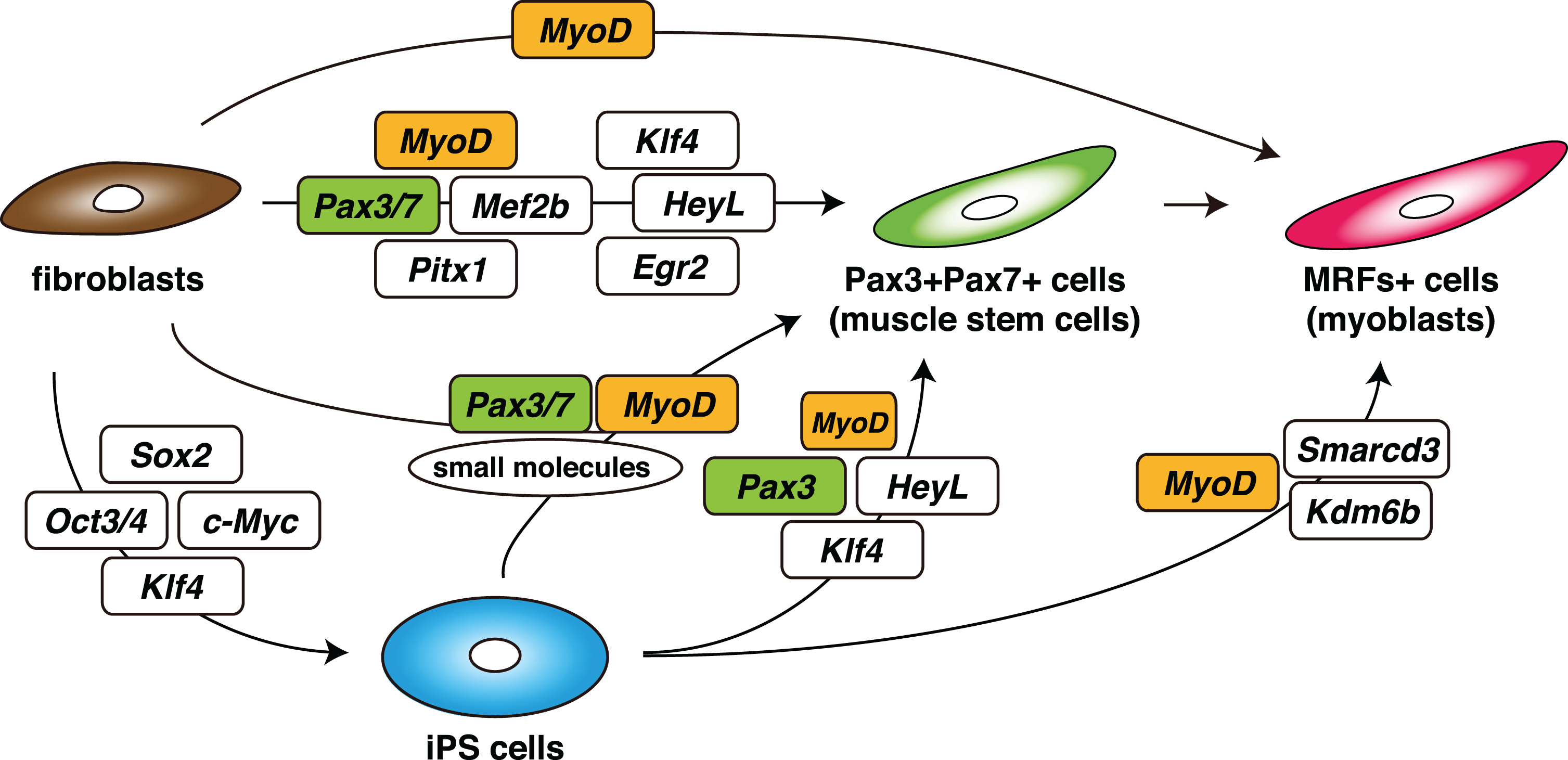
Human mesoangioblast-like stem cells, which have the potential to differentiate into skeletal muscle, have been successfully generated from hiPSCs by multistep protocols [47, 67]. They displayed similar gene expression profiles as mouse embryonic mesoangioblasts, however these induced cells do not spontaneously differentiate into skeletal muscle cells. Therefore MyoD overexpression was utilized to make them differentiate. Similar to mesoangioblasts, myogenic cells derived from mesoangioblast-like cells could be delivered to injured muscle via intramuscular and intra-arterial routes. Furthermore, these cells have been generated from hiPSCs derived from limb-girdle muscular dystrophy patients, used for genomic correction and cell transplantation experiments for potential therapeutic application.
Overexpression of myogenic master transcription factors such as MyoD or Pax3/Pax7 is an excellent strategy for myogenic induction in hiPSCs, which can be utilized for in vitro muscle disease models for functional tests and drug screening, and these transgene-based induction methods appear to give rise to a more homogeneous population of muscle progenitors or stem cells. However, for safe stem cell therapy, it is essential to maintain the good cellular and genetic qualities of hiPSC-derived myogenic stem cells before transplantation. Selection of the appropriate hiPS clone with the best genomic integration sites of overexpression vectors for myogenic core transcription factors and appropriate expression control of these transgenes would be necessary for the safety of using these induced myogenic cells for therapeutic stem cell transplantation.
Stepwise induction of myogenic stem cells derived from hiPSCs
Stepwise induction protocols utilizing small molecules and growth factors have been established as alternative myogenic induction approaches and a more applicable method for therapeutic situations [14]. Current studies for myogenic differentiation with iPSCs have been designed with some growth factors and small molecules, which follow in vivo myogenic development, in combination with EB aggregation and cell sorting to separate mesodermal cells. To induce paraxial mesoderm cells from mouse ES cells, BMP4 in serum-free cultures was expressed under controlled conditions [68]. A few days after treatment with BMP4, mouse ES cells could be differentiated into mesodermal cells with charateristics of the primitive streak, however continuous treatment with BMP4 turned differentiating ES cells into osteogenic cells. Treatment with LiCl, to enhance Wnt signaling, is able to induce myogenic differentiation after treatment with BMP4 [69]. After treatment with these molecules, PDGFRα+ E-cadherin- paraxial mesodermal cells were sorted and cultured with IGF-1, HGF, and FGF to induce myogenic differentiation. Wnt3a has been used to promote efficient skeletal muscle differentiation of human ES cells [70]. It is likely that the induced cell populations from pluripotent stem cells may contain other cell types such as neural crest cells because these cells share similar transcription factors with myogenic cells, such as Pax3/Pax7, or may contain other mesodermal derivatives. To overcome this limitation, differentiation into lateral mesodermal cells can be prevented by subsequent treatment with a BMP4 inhibitor [14, 71]. By treatment with an optimum amount of GSK3β inhibitors and then BMP4 inhibitors in ES/iPS cultures, differentiation into somitic mesoderm and myogenic cells could be controlled. Current protocols have been published and describe more details for hiPSC differentiation [71].
It has been shown that hiPSCs with high concentrations of FGF2 and EGF in the form of cell aggregates, termed EZ spheres, efficiently give rise to myogenic progenitors [72, 73]. After a few weeks culture, cells expressed Pax7, MyoD, or Myogenin. However, the authors also showed that EZ spheres included high amounts of Tuj1+ neural cells. Hence the interest in the utilization of molecules for activation of mesodermal and myogenic signaling pathways such as BMPs and Wnts.
While these induction methods using growth factors and small molecules give rise to differentiated myotubes in vitro, the in vivo contribution of such myogenic progenitors has been less extensively evaluated than those obtained using transgenes, and where it has been evaluated, engraftment has not been sufficient for muscle functional testing. A major advantage of approaches with transgenes, rather than withjust growth factors and small molecules, is that they enable the scalable exponential expansion of functionally engraftable myogenic stem cells to obtain much larger quantities of engraftable cells.
Collection of myogenic cells derived from hiPSCs
It is unavoidable that induced myogenic progenitors or stem cells derived from iPSCs by various methods contain non-myogenic cells. Therefore, further purification procedures are necessary for therapeutic applications to exclude tumorigenic risks of contamination with undifferentiated non-myogenic cells. Cell sorting of mesodermal progenitor cells, mesenchymal precursor cells, or myogenic cells is a powerful tool to obtain selected myogenic populations from differentiated pluripotent cells. Induced PDGFRα+ Kdr–mesodermal progenitors, derived from hiPSCs, selected by cell sorting show myogenic differentiation [74].
Isolated CD73 + mesenchymal cells from human ES or iPS cells are multipotent, and can differentiate into fat, cartilage, bone, and skeletal muscle cells. It was also demonstrated that hESCs cultured on feeder cells generated a few percentages of CD73 + adult mesenchymal stem cell-like cells [75]. These CD73 + mesenchymal stem cell-like cells were expanded and then gave rise to CD56 (NCAM) + myogenic cells. Purified CD56 + myogenic cells when transplanted into immunodeficient mice contributed to regenerating muscle [76]. hiPSC-derived myogenic cells have been shown to differentiate into c-met + CD184(CXCR4) + AChR+ cells, or CD56 + CD271(NGFR)- cells, with the majority of sorted cells contain Pax7+ myogenic progenitors or stem cells [77, 78]. To identify human muscle stem cells from human muscle biopsies, CD56 + CD82+, EGFR + ITGB1+, CXCR4 + CD29 + CD56+, or CD90 + CD318+ antibodies have been also used as cell surface markers. These markers might be useful to identify human muscle stem cells derived from hiPSCs [79–83]. Mouse muscle stem cells marked by Pax7 have been sorted as SM/C-2.6+ cells from mouse iPS cells [84, 85]. These SM/C-2.6+ cells were successfully engrafted into mouse regenerating myofibers, however, the SM/C-2.6 antibody only recognizes mouse cells but not human cells [84–86]. In addition to myogenic cells derived directly ES or iPS cells, alpha-7 Integrin + VCAM + (CD106+) cells in a teratoma derived from hiPSCs formed in the tibialis anterior muscle, showed remarkable in vivo regenerative potential [87]. This comprehensive use of surface antibodies for positive and negative selections greatly enhances the efficiency of flow cytometry and effectively removes non-myogenic progenitors and stem cells. In addition to potential therapeutic applications, this advance is important for studies that require highly pure muscle stem cell samples such as transcriptional analyses, or refinement of transplantation techniques.
Conclusions
To optimize the use of skeletal muscle progenitors or stem cells for cell therapy to treat skeletal muscle disorders, standardized protocols, if possible, with transgene-free methods, which are rapidly available and at low cost, to efficiently induce transplantable cells from hiPSCs are socially desirable. Risk reduction of tumorigenesis in induced cells and systemic delivery of therapeutic cells to reach a wide range of muscle tissues are also required and they are still highly challenging. Since differentiated myotubes derived from pluripotent stem cells do not proliferate, they are not suited for cell transplantation. At present, all the induction approaches produce muscle precursors or stem cells, that are vastly less efficient at engraftment than bona fide muscle satellite cells (Table 1). The difference in myogenic potency of these induced cells compared to muscle satellite cells in vivo is striking [58, 64]. Therefore, better cell culture methods need to be established to obtain proliferating myogenic precursors or stem cells in vitro with more efficient in vivo regenerative potential, while ensuring the capacity to expand large quantities of transplantable cells.
Table 1
The summary of transplantation results with genes or chemicals
Reference | Ref No. | Species | Original cells | Additional Factors | Selection | Duration of cell culture | Transplanted cells | Results (restored Dystrophin + fibers) |
Darabi et al., Stem Cells (2011) | 53 | Mouse | ES | Embryoid body, incucible Pax3, inducible Pax7 | PDGFR+; Flk1+ | 7 days | 1.0×106 | 15%; Dys+/total TA fibers of mdx (after 4wks) |
Darabi et al., Cell Stem Cell (2012) | 54 | Human | ES/iPS | Embryoid body, inducible PAX7 | PAX7–GFP+ | 7 days | 3.0×105 | About 100 of DYS+ in TA fibers of NSG-mdx (after 4wks) |
Tedesco et al., Sci Trans Med (2012) | 47 | Mouse, human | iPS (LGMD2D) | Mesoangioblast-like cells, inducible MyoD | SSEA1–(mouse iPS) | 4 weeks | 1.0×106 | bout 300 of Sgca+ (mouse iPS), 50 of SGCA+ (human iPS) in TA fibers of Sgca-null (after 4wks) |
Chal et al., Nat Biotech (2015) | 14 | Human | ES/iPS | CHIR99021, LDN193189, FGF2, HGF, IGF-1 | Pax7+ | 4 weeks | N.D. | 23% of nuclei were PAX7+ in culture cells |
Ito et al., Sci Rep (2017) | 62 | Mouse | Fibroblast | Pax3/7, Mef2b, Pitx1 or Pax7, Mef2b, MyoD | Calcein+ | 3 weeks | 1.0×105 | About 80 of Dys+ in TA fibers of mdx (after 2wks) |
Hicks et al., Nat Cell Biol (2017) | 78 | Human | iPS | CHIR99021, FGF2, N2 supplement, SB431542 | HNK–; NCAM+; ERBB3+; NGFR+ | 50 days | 1.0×106 | About 150 of DYS+ in TA fibers of NSG-mdx (after 30days) |
Bar-Nur et al., Stem Cell Rep (2018) | 66 | Mouse | Fibroblast | inducible MyoD, Forskolin, GSK3beta, RepSox | N.D. | 14 days | 1.0×106 | About 400 of Dys+in TA fibers of mdx (after 4wks) |
Sakai-Takemura et al., Sci Rep (2018) | 73 | Human | iPS | EZ sphere, CHIR99021, LDN193189, FGF2, HGF, IGF-1 | ERBB3+; NGRF+ | 6 weeks | 1.0×105 | About 5 20 of DYS+in TA fibers of NSG-mdx (after 3wks) |
Sato et al., Stem Cell Rep (2019) | 63 | Human | Fibroblast/iPS | CHIR99021, SB431542, DMH1, inducible MYOD, PAX3, HEYL, KLF4 | PAX3-EGFP+ | 18 days | 1.0×106 | About 300 of DYS+/total TA fibers of NSG-DMD null (after 4wks) |
Chan et al., Cell Stem Cell (2019) | 87 | Mouse | ES/iPS | teratoma (2.5×105 ES or 1×106 iPS cells in TA muscle), Cardiotoxin | a7-integrin+; VCAM-1+ | 3 weeks | 4.0×104 | 80%; Dys+/total TA fibers of NSG-mdx (after 3months) |
Taken together, current myogenic induction protocols with small molecules and growth factors, with or without myogenic transcription factors, have been largely improved. In the near future, it would be crucial to standardize the myogenic induction protocols to obtain sufficient conversion of myogenic cells derived from pluripotent stem cells or by direct reprograming techniques, for efficient stem cell therapy in the treatment of DMD and other muscular dystrophies.
CONFLICT OF INTEREST
The author declares no conflict of interest.
ACKNOWLEDGMENTS
This work was supported by grants-in-aid from the Ministry of Education, Culture, Sports, Science and Technology (MEXT), Projects for Technology Development, Development of Cell Transplantation Methods for Refractory Muscle Diseases (AMED), and AMED-CREST mechanobiology.
REFERENCES
[1] | Brack AS , Rando TA . Tissue-specific stem cells: Lessons from the skeletal muscle satellite cell. Cell Stem Cell. (2012) ;10: (5):504–14. |
[2] | Seale P , Asakura A , Rudnicki MA . The potential of muscle stem cells. Dev Cell. (2001) ;1: (3):333–42. |
[3] | Brack AS , Conboy MJ , Roy S , Lee M , Kuo CJ , Keller C , et al. Increased Wnt Signaling During Aging Alters Muscle Stem Cell Fate and Increases Fibrosis. Science. (2007) ;317: (5839):807–10. |
[4] | Yin H , Pasut A , Soleimani VD , Bentzinger CF , Antoun G , Thorn S , et al. MicroRNA-133 Controls Brown Adipose Determination in Skeletal Muscle Satellite Cells by Targeting Prdm16. Cell Metab. (2013) ;17: (2):210–24. |
[5] | Pessina P , Kharraz Y , Jardi M , Fukada S , Serrano AL , Perdiguero E , et al. Fibrogenic Cell Plasticity Blunts Tissue Regeneration and Aggravates Muscular Dystrophy. Stem Cell Rep. (2015) ;4: (6):1046–60. |
[6] | Cerletti M , Jurga S , Witczak CA , Hirshman MF , Shadrach JL , Goodyear LJ , et al. Highly efficient, functional engraftment of skeletal muscle stem cells in dystrophic muscles. Cell. (2008) ;134: (1):37–47. |
[7] | Skuk D , Tremblay JP . Cell therapy in muscular dystrophies: Many promises in mice and dogs, few facts in patients. Expert OpinBiolTher. (2015) ;15: (9):1307–19. |
[8] | Negroni E , Bigot A , Butler-Browne GS , Trollet C , Mouly V . Cellular Therapies for Muscular Dystrophies: Frustrations and Clinical Successes. Hum Gene Ther. (2016) ;27: (2):117–26. |
[9] | Takahashi K , Yamanaka S . Induction of pluripotent stem cells from mouse embryonic and adult fibroblast cultures by defined factors. Cell. (2006) ;126: (4):663–76. |
[10] | Takahashi K , Tanabe K , Ohnuki M , Narita M , Ichisaka T , Tomoda K , Yamanaka S . Induction of pluripotent stem cells from adult human fibroblasts by defined factors. Cell. (2007) ;131: (5):861–72. |
[11] | Buckingham M , Relaix F . The role of Pax genes in the development of tissues and organs: Pax3 and Pax7 regulate muscle progenitor cell functions. Annu Rev Cell Dev Biol. (2007) ;23: :645–73. |
[12] | Münsterberg AE , Kitajewski J , Bumcrot DA , McMahon AP , Lassar AB . Combinatorial signaling by Sonic hedgehog and Wnt family members induces myogenic bHLH gene expression in the somite. Genes Dev. (1995) ;9: (23):2911–22. |
[13] | Zammit PS . Function of the myogenic regulatory factors Myf5, MyoD, Myogenin and MRF4 in skeletal muscle, satellite cells and regenerative myogenesis. Semin Cell Dev Biol. (2017) ;72: :19–32. |
[14] | Chal J , Oginuma M , Al Tanoury Z , Gobert B , Sumara O , Hick A , et al. Differentiation of pluripotent stem cells to muscle fiber to model Duchenne muscular dystrophy. Nat Biotechnol. (2015) ;33: (9):962–9. |
[15] | Darabi R , Gehlbach K , Bachoo RM , Kamath S , Osawa M , Kamm KE , et al. Functional skeletal muscle regeneration from differentiating embryonic stem cells. Nat Med. (2008) ;14: (2):134–43. |
[16] | Pourquié O , Al Tanoury Z , Chal J . The Long Road to Making Muscle In Vitro. Curr Top Dev Biol. (2018) ;129: :123–42. |
[17] | Lagha M , Sato T , Bajard L , Daubas P , Esner M , Montarras D , et al. Regulation of skeletal muscle stem cell behavior by Pax3 and Pax7. Cold Spring HarbSymp Quant Biol. (2008) ;73: :307–15. |
[18] | Carvajal JJ , Keith A , Rigby PW . Global transcriptional regulation of the locus encoding the skeletal muscle determination genes Mrf4 and Myf5. Genes Dev. (2008) ;22: (2):265–76. |
[19] | Daubas P , Duval N , Bajard L , Langa Vives F , Robert B , Mankoo BS , et al. Fine-tuning the onset of myogenesis by homeobox proteins that interact with the Myf5 limb enhancer. Biol Open. (2015) ;4: (12):1614–24. |
[20] | Braun T , Arnold HH . Inactivation of Myf-6 and Myf-5 genes in mice leads to alterations in skeletal muscle development. EMBO J. (1995) ;14: (6):1176–86. |
[21] | Kassar-Duchossoy L , Gayraud-Morel B , Gomès D , Rocancourt D , Buckingham M , Shinin V , et al. Mrf4 determines skeletal muscle identity in Myf5:Myod double-mutant mice. Nature. (2004) ;431: (7007):466–71. |
[22] | Marcelle C , Stark MR , Bronner-Fraser M . Coordinate actions of BMPs, Wnts, Shh and noggin mediate patterning of the dorsal somite. Development. (1997) ;124: (20):3955–63. |
[23] | Rios AC , Serralbo O , Salgado D , Marcelle C . Neural crest regulates myogenesis through the transient activation of NOTCH. Nature. (2011) ;473: (7348):532–5. |
[24] | Nitzan E , Kalcheim C . Neural crest and somitic mesoderm as paradigms to investigate cell fate decisions during development. Dev Growth Differ. (2013) ;55: (1):60–78. |
[25] | Linker C , Lesbros C , Stark MR , Marcelle C . Intrinsic signals regulate the initial steps of myogenesis in vertebrates. Development. (2003) ;130: (20):4797–807. |
[26] | Bajard L , Relaix F , Lagha M , Rocancourt D , Daubas P , Buckingham ME . A novel genetic hierarchy functions during hypaxial myogenesis: Pax3 directly activates Myf5 in muscle progenitor cells in the limb. Genes Dev. (2006) ;20: (17):2450–64. |
[27] | Sato T , Rocancourt D , Marques L , Thorsteinsdóttir S , Buckingham M . A Pax3/Dmrt2/Myf5 regulatory cascade functions at the onset of myogenesis. PLoS Genet. (2010) ;6: (4):e1000897. |
[28] | Relaix F , Rocancourt D , Mansouri A , Buckingham M . A Pax3/Pax7-dependent population of skeletal muscle progenitor cells. Nature. (2005) ;435: (7044):948–53. |
[29] | Lassar AB , Paterson BM , Weintraub H . Transfection of a DNA locus that mediates the conversion of 10T1/2 fibroblasts to myoblasts. Cell. (1986) ;47: (5):649–56. |
[30] | Davis RL , Weintraub H , Lassar AB . Expression of a single transfected cDNA converts fibroblasts to myoblasts. Cell. (1987) ;51: (6):987–1000. |
[31] | Tapscott SJ , Davis RL , Thayer MJ , Cheng PF , Weintraub H , Lassar AB . MyoD1: A nuclear phosphoprotein requiring a Myc homology region to convert fibroblasts to myoblasts. Science. (1988) ;242: (4877):405–11. |
[32] | Rudnicki MA , Braun T , Hinuma S , Jaenisch R . Inactivation of MyoD in mice leads to up-regulation of the myogenic HLH gene Myf-5 and results in apparently normal muscle development. Cell. (1992) ;71: (3):383–90. |
[33] | Braun T , Rudnicki MA , Arnold HH , Jaenisch R . Targeted inactivation of the muscle regulatory gene Myf-5 results in abnormal rib development and perinatal death. Cell. (1992) ;71: (3):369–82. |
[34] | Rudnicki MA , Schnegelsberg PN , Stead RH , Braun T , Arnold HH , Jaenisch R . MyoD or Myf-5 is required for the formation of skeletal muscle. Cell. (1993) ;75: (7):1351–9. |
[35] | Hasty P , Bradley A , Morris JH , Edmondson DG , Venuti JM , Olson EN , et al. Muscle deficiency and neonatal death in mice with a targeted mutation in the myogenin gene. Nature. (1993) ;364: (6437):501–6. |
[36] | Nabeshima Y , Hanaoka K , Hayasaka M , Esumi E , Li S , Nonaka I , et al. Myogenin gene disruption results in perinatal lethality because of severe muscle defect. Nature. (1993) ;364: (6437):532–5. |
[37] | Braun T , Arnold HH . Inactivation of Myf-6 and Myf-5 genes in mice leads to alterations in skeletal muscle development. EMBO J. (1995) ;14: (6):1176–86. |
[38] | Kassar-Duchossoy L , Gayraud-Morel B , Gomès D , Rocancourt D , Buckingham M , Shinin V , et al. Mrf4 determines skeletal muscle identity in Myf5:Myod double-mutant mice. Nature. (2004) ;431: (7007):466–71. |
[39] | Dekel I , Magal Y , Pearson-White S , Emerson CP , Shani M . Conditional conversion of ES cells to skeletal muscle by an exogenous MyoD1 gene. New Biol. (1992) ;4: (3):217–24. |
[40] | Ozasa S , Kimura S , Ito K , Ueno H , Ikezawa M , Matsukura M , et al. Efficient conversion of ES cells into myogenic lineage using the gene-inducible system. BiochemBiophys Res Commun. (2007) ;357: (4):957–63. |
[41] | Warren L , Manos PD , Ahfeldt T , Loh YH , Li H , Lau F , et al. Highly efficient reprogramming to pluripotency and directed differentiation of human cells with synthetic modified mRNA. Cell Stem Cell. (2010) ;7: (5):618–30. |
[42] | Tanaka A , Woltjen K , Miyake K , Hotta A , Ikeya M , Yamamoto T , et al. Efficient and reproducible myogenic differentiation from human iPS cells: Prospects for modeling Miyoshi Myopathy in vitro. PLoS One. (2013) ;8: (4):e61540. |
[43] | Abujarour R , Bennett M , Valamehr B , Lee TT , Robinson M , Robbins D , et al. Myogenic differentiation of muscular dystrophy-specific induced pluripotent stem cells for use in drug discovery. Stem Cells Transl Med. (2014) ;3: (2):149–60. |
[44] | Albini S , Coutinho P , Malecova B , Giordani L , Savchenko A , Forcales SV , et al. Epigenetic reprogramming of human embryonic stem cells into skeletal muscle cells and generation of contractile myospheres. Cell Rep. (2013) ;3: (3):661–70. |
[45] | Gianakopoulos PJ , Mehta V , Voronova A , Cao Y , Yao Z , Coutu J , et al. MyoD directly up-regulates premyogenic mesoderm factors during induction of skeletal myogenesis in stem cells. J Biol Chem. (2011) ;286: (4):2517–25. |
[46] | Akiyama T , Wakabayashi S , Soma A , Sato S , Nakatake Y , Oda M , et al. Transient ectopic expression of the histone demethylase JMJD3 accelerates the differentiation of human pluripotent stem cells. Development. (2016) ;143: :3674–85. |
[47] | Tedesco FS , Gerli MF , Perani L , Benedetti S , Ungaro F , Cassano M , et al. Transplantation of genetically corrected human iPSC-derived progenitors in mice with limb-girdle muscular dystrophy. Sci TranslMed. (2012) ;4: (140):140ra89. |
[48] | Maffioletti SM , Gerli MFM , Ragazzi M , Dastidar S , Benedetti S , Loperfido M , et al. Efficient derivation and inducible differentiation of expandable skeletal myogenic cells from human ES and patient-specific iPS cells. Nat Protoc. (2015) ;10: (7):941–58. |
[49] | Shoji E , Sakurai H , Nishino T , Nakahata T , Heike T , Awaya T , et al. Early pathogenesis of Duchenne muscular dystrophy modelled in patient-derived human induced pluripotent stem cells. Sci Rep. (2015) ;5: :12831. |
[50] | Li HL , Fujimoto N , Sasakawa N , Shirai S , Ohkame T , Sakuma T , et al. Precise correction of the dystrophin gene in Duchenne muscular dystrophy patient induced pluripotent stem cells by TALEN and CRISPR-Cas9. Stem Cell Reports. (2015) ;4: (1):143–54. |
[51] | Rao L , Qian Y , Khodabukus A , Ribar T , Bursac N . Engineering human pluripotent stem cells into a functional skeletal muscle tissue. Nat Commun. (2018) ;9: :126. |
[52] | Maffioletti SM , Sarcar S , Henderson ABH , Mannhardt I , Pinton L , Moyle LA , et al. Three-dimensional human iPSC-derived artificial skeletal muscles model muscular dystrophies and enable multilineage tissue engineering. Cell Rep. (2018) ;23: (3):899–908. |
[53] | Darabi R , Santos FN , Filareto A , Pan W , Koene R , Rudnicki MA , et al. Assessment of the myogenic stem cell compartment following transplantation of Pax3/Pax7-induced embryonic stem cell-derived progenitors. Stem Cells. (2011) ;29: (5):777–90. |
[54] | Darabi R , Arpke RW , Irion S , Dimos JT , Grskovic M , Kyba M , et al. Human ES- and iPS-derived myogenic progenitors restore DYSTROPHIN and improve contractility upon transplantation in dystrophic mice. Cell Stem Cell. (2012) ;10: (5):610–9. |
[55] | Thorburn AM , Walton PA , Feramisco JR . MyoD induced cell cycle arrest is associated with increased nuclear affinity of the Rb protein. MolBiol Cell. (1993) ;4: (7):705–13. |
[56] | Lattanzi L , Salvatori G , Coletta M , Sonnino C , Cusella De Angelis MG , Gioglio L , et al. High efficiency myogenic conversion of human fibroblasts by adenoviral vector-mediated MyoD gene transfer. An alternative strategy for ex vivo gene therapy of primary myopathies. J Clin Invest. (1998) ;101: (10):2119–28. |
[57] | Collins CA , Olsen I , Zammit PS , Heslop L , Petrie A , Partridge TA , et al. Stem cell function, self-renewal, and behavioral heterogeneity of cells from the adult muscle satellite cell niche. Cell. (2005) ;122: (2):289–301. |
[58] | Sacco A , Doyonnas R , Kraft P , Vitorovic S , Blau HM . Self-renewal and expansion of single transplanted muscle stem cells. Nature. (2008) ;456: (7221):502–6. |
[59] | Zammit PS . All muscle satellite cells are equal, but are some more equal than others? J Cell Sci. (2008) ;121: (Pt 18):2975–82. |
[60] | Fukada S , Uezumi A , Ikemoto M , Masuda S , Segawa M , Tanimura N , et al. Molecular signature of quiescent satellite cells in adult skeletal muscle. Stem Cells. (2007) ;25: (10):2448–59. |
[61] | Pallafacchina G , François S , Regnault B , Czarny B , Dive V , Cumano A , et al. An adult tissue-specific stem cell in its niche: A gene profiling analysis of in vivo quiescent and activated muscle satellite cells. Stem Cell Res. (2010) ;4: (2):77–91. |
[62] | Ito N , Kii I , Shimizu N , Tanaka H , Takeda S . Direct reprogramming of fibroblasts into skeletal muscle progenitor cells by transcription factors enriched in undifferentiated subpopulation of satellite cells. Sci Rep. (2017) ;7: (1):8097. |
[63] | Sato T , Higashioka K , Sakurai H , Yamamoto T , Goshima N , Ueno M , et al. Core Transcription Factors Promote Induction of PAX3-Positive Skeletal Muscle Stem Cells. Stem Cell Reports. (2019) ;13: (2):352–65. |
[64] | Montarras D , Morgan J , Collins C , Relaix F , Zaffran S , Cumano A , et al. Direct isolation of satellite cells for skeletal muscle regeneration. Science. (2005) ;309: (5743):2064–7. |
[65] | Sato T , Yamamoto T , Sehara-Fujisawa A . miR-195/497 induce postnatal quiescence of skeletal muscle stem cells. Nat Commun. (2014) ;5: :4597. |
[66] | Bar-Nur O , Gerli MFM , Di Stefano B , Almada AE , Galvin A , Coffey A , et al. Direct Reprogramming of Mouse Fibroblasts into Functional Skeletal Muscle Progenitors. Stem Cell Reports. (2018) ;10: (5):1505–21. |
[67] | Gerli MF , Maffioletti SM , Millet Q , Tedesco FS . Transplantation of induced pluripotent stem cell-derived mesoangioblast-like myogenic progenitors in mouse models of muscle regeneration. J Vis Exp. (2014) ;(83):e50532. |
[68] | Sakurai H , Inami Y , Tamamura Y , Yoshikai T , Sehara-Fujisawa A , Isobe K . Bidirectional induction toward paraxial mesodermal derivatives from mouse ES cells in chemically defined medium. Stem Cell Res. (2009) ;3: (2-3):157–69. |
[69] | Sakurai H , Sakaguchi Y , Shoji E , Nishino T , Maki I , Sakai H , et al. In vitro modeling of paraxial mesodermal progenitors derived from induced pluripotent stem cells. PLoS One. (2012) ;7: (10):e47078. |
[70] | Hwang Y , Suk S , Shih YR , Seo T , Du B , Xie Y , et al. WNT3A promotes myogenesis of human embryonic stem cells and enhances in vivo engraftment. Sci Rep. (2014) ;4: :5916. |
[71] | Chal J , Al Tanoury Z , Hestin M , Gobert B , Aivio S , Hick A , et al. Generation of human muscle fibers and satellite-like cells from human pluripotent stem cells in vitro. Nat Protoc. (2016) ;11: (10):1833–50. |
[72] | Hosoyama T , McGivern JV , Van Dyke JM , Ebert AD , Suzuki M . Derivation of myogenic progenitors directly from human pluripotent stem cells using a sphere-based culture. Stem Cells Transl Med. (2014) ;3: (5):564–74. |
[73] | Sakai-Takemura F , Narita A , Masuda S , Wakamatsu T , Watanabe N , Nishiyama T , et al. , Premyogenic progenitors derived from human pluripotent stem cells expand in floating culture and differentiate into transplantable myogenic progenitors. Sci Rep. (2018) ;8: :6555. |
[74] | Sakurai H , Okawa Y , Inami Y , Nishio N , Isobe K . Paraxial mesodermal progenitors derived from mouse embryonic stem cells contribute to muscle regeneration via differentiation into muscle satellite cells. Stem Cells. (2008) ;26: (7):1865–73. |
[75] | Barberi T , Bradbury M , Dincer Z , Panagiotakos G , Socci ND , Studer L . Derivation of engraftable skeletal myoblasts from human embryonic stem cells. Nat Med. (2007) ;13: (5):642–8. |
[76] | Barberi T , Willis LM , Socci ND , Studer L . Derivation of multipotent mesenchymal precursors from human embryonic stem cells. PLoS Med. (2005) ;2: (6):e161. |
[77] | Borchin B , Chen J , Barberi T . Derivation and FACS-mediated purification of PAX3+/PAX7+skeletal muscle precursors from human pluripotent stem cells. Stem Cell Reports. (2013) ;1: (6):620–31. |
[78] | Hicks MR , Hiserodt J , Paras K , Fujiwara W , Eskin A , Jan M , et al. ERBB3 and NGFR mark a distinct skeletal muscle progenitor cell in human development and hPSCs. Nat Cell Biol. (2018) ;20: (1):46–57. |
[79] | Alexander MS , Rozkalne A , Colletta A , Spinazzola JM , Johnson S , Rahimov F , et al. CD82 Is a Marker for Prospective Isolation of Human Muscle Satellite Cells and Is Linked to Muscular Dystrophies. Cell Stem Cell. (2016) ;19: (6):800–7. |
[80] | Yamanaka Y , Takenaka N , Sakurai H , Ueno M , Kinoshita S , Sotozono C , et al. Human Skeletal Muscle Cells Derived from the Orbicularis Oculi Have Regenerative Capacity for Duchenne Muscular Dystrophy. Int J Mol Sci. (2019) ;20: (14). pii:E3456. |
[81] | Charville GW , Cheung TH , Yoo B , Santos PJ , Lee GK , Shrager JB , et al. Ex Vivo Expansion and In Vivo Self-Renewal of Human Muscle Stem Cells. Stem Cell Reports. (2015) ;5: (4):621–32. |
[82] | Garcia SM , Tamaki S , Lee S , Wong A , Jose A , Dreux J , et al. High-Yield Purification, Preservation, and Serial Transplantation of Human Satellite Cells. Stem Cell Reports. (2018) ;10: (3):1160–74. |
[83] | Tey SR , Robertson S , Lynch E , Suzuki M . Coding Cell Identity of Human Skeletal Muscle Progenitor Cells Using Cell Surface Markers: Current Status and Remaining Challenges for Characterization and Isolation. Front Cell Dev Biol. (2019) ;7: :284. |
[84] | Chang H , Yoshimoto M , Umeda K , Iwasa T , Mizuno Y , Fukada S , et al. Generation of transplantable, functional satellite-like cells from mouse embryonic stem cells. FASEB J. (2009) Jun;23: (6):1907–19. |
[85] | Mizuno Y , Chang H , Umeda K , Niwa A , Iwasa T , Awaya T , et al. Generation of skeletal muscle stem/progenitor cells from murine induced pluripotent stem cells. FASEB J. (2010) ;24: (7):2245–53. |
[86] | Fukada S , Higuchi S , Segawa M , Koda K , Yamamoto Y , Tsujikawa K , et al. Purification and cell-surface marker characterization of quiescent satellite cells from murine skeletal muscle by a novel monoclonal antibody. Exp Cell Res. (2004) ;296: (2):245–55. |
[87] | Chan SS , Arpke RW , Filareto A , Xie N , Pappas MP , Penaloza JS , et al. Skeletal Muscle Stem Cells from PSC-Derived Teratomas Have Functional Regenerative Capacity. Cell Stem Cell. (2018) ;23: (1):74–85. |