Huntington’s Disease and Diabetes: Chronological Sequence of its Association
Abstract
Although Huntington’s disease (HD) is primarily considered a rare neurodegenerative disorder, it has been linked to glucose metabolism alterations and diabetes, as has been described in other neuro syndromes such as Friedreich’s ataxia or Alzheimer’s disease. This review surveys the existing literature on HD and its potential relationship with diabetes, glucose metabolism-related indexes and pancreas morphology, in humans and in animal’s models. The information is reported in chronological sequence. That is, studies performed before and after the identification of the genetic defect underlying HD (CAG: encoding glutamine ≥36 repeats located in exon 1 of the HTT gene) and with the development and evolution of HD animal models. The aim of the review is to evaluate whether impaired glucose metabolism contributes to the development of HD, and whether optimized glycemic control may ameliorate the symptoms of HD.
A GLANCE AT HUNTINGTON’S DISEASE
It was in 1872, when a report entitled “On chorea” was published by Dr. George Huntington. He characterized the disease known today, eponymously as Huntington’s disease (HD) to be an inherited condition, and described its principal clinical aspects [1]. HD is mainly considered, a rare – the USA/Western Europe/Australia prevalence is 5–10 affected per 100,000 people–genetic neurodegenerative disease, that causes progressive degeneration of the neostriatum and one that results in progressive movement disorder – chorea and dystonia–, progressive cognitive disturbance culminating in dementia, and several behavioural alterations [2]. In 1993, the HD-Collaborative-Research Group identified the genetic defect underlying this autosomal dominant pathology as a trinucleotide expansion (CAG: encoding glutamine ≥36 repeats) located in exon 1 of the HTT gene. The gene encodes the huntingtin protein, which is 3,144 amino acids long [3]. The presence of the mutated protein in the brain induces the formation of aggregates in the cytosol and nucleus causing generalized atrophy. The protein is ubiquitous in the body, though its functionality remains unknown [4].
Even though the length of the CAG-expansion is the major determining factor of the progression and pathogenesis rates, in 2015, a genome-wide association study identified genetic variants that alter the age of onset of the disease accelerating it to 6.1 years (rs146353869) or inducing a delay of 1.4 years (rs2140734) [5]. Although the onset of the phenotype can range from early childhood – Westphal variant, 4–6% – to >70 years – senile chorea, 8–10% –, the symptoms usually begin about halfway through the average human lifespan (30–50 years), evolving progressively and continuously (15–20 years) until death caused by the disease, or in some cases, suicide [6]. A definitive treatment for HD has yet to emerge [7].
BEYOND A BRAIN DISORDER
HD is not only a brain disorder, as the neuropathology is also accompanied by formation of polyglutamine inclusions in non-central nervous system tissues, resulting in widespread abnormal changes [8]. The pervasive mutant polyQ-HTT, disrupts many cellular processes such as autophagy, gene transcription and energy metabolism [9, 10], and several sources of evidence point to a role played by mitochondrial/metabolic dysfunction in HD, along with drastic weight loss, skeletal-muscle wasting and modifications in appetite, likely conducted by the hypothalamic-endocrine axis [11]. HD subjects show endocrine changes such as increased cortisol levels and reduced testosterone values [12]. Beyond that, some researches have described abnormalities of carbohydrate metabolism and increased prevalence of diabetes [13, 14]. The impact on the endocrine system has also been noticed in certain hereditary neurological diseases, where it has been suggested that the prevalence of glucose metabolic impairment is significantly higher compared to the general population [15–17]. In the last quarter of the twentieth century, a study reported that approximately 10% of individuals diagnosed with HD had diabetes [13], and by the early twenty-first century, multiple articles had found diabetes in HD murine models [18–21]. Unlike those studies, in premanifest and moderate symptomatic HD subjects, the values of classical glucose metabolic markers were similar, and a very low prevalence of serious comorbidities such as diabetes was reported [22, 23], challenging the assumption of the increased risk of diabetes among the HD population [24, 25].
This review surveys the existing literature on HD and its potential relationship with diabetes and glucose metabolic alterations, in human and animal models, aiming to determine, whether impaired glucose metabolism contributes to the development of HD, and whether optimized glycemic control may ameliorate the symptoms of HD (Fig. 1).
Fig.1
Summary of the studies carried out in HD subjects and murine models from 1957 to 2016 including the different aspects of alterations in glucose metabolism and diabetes.
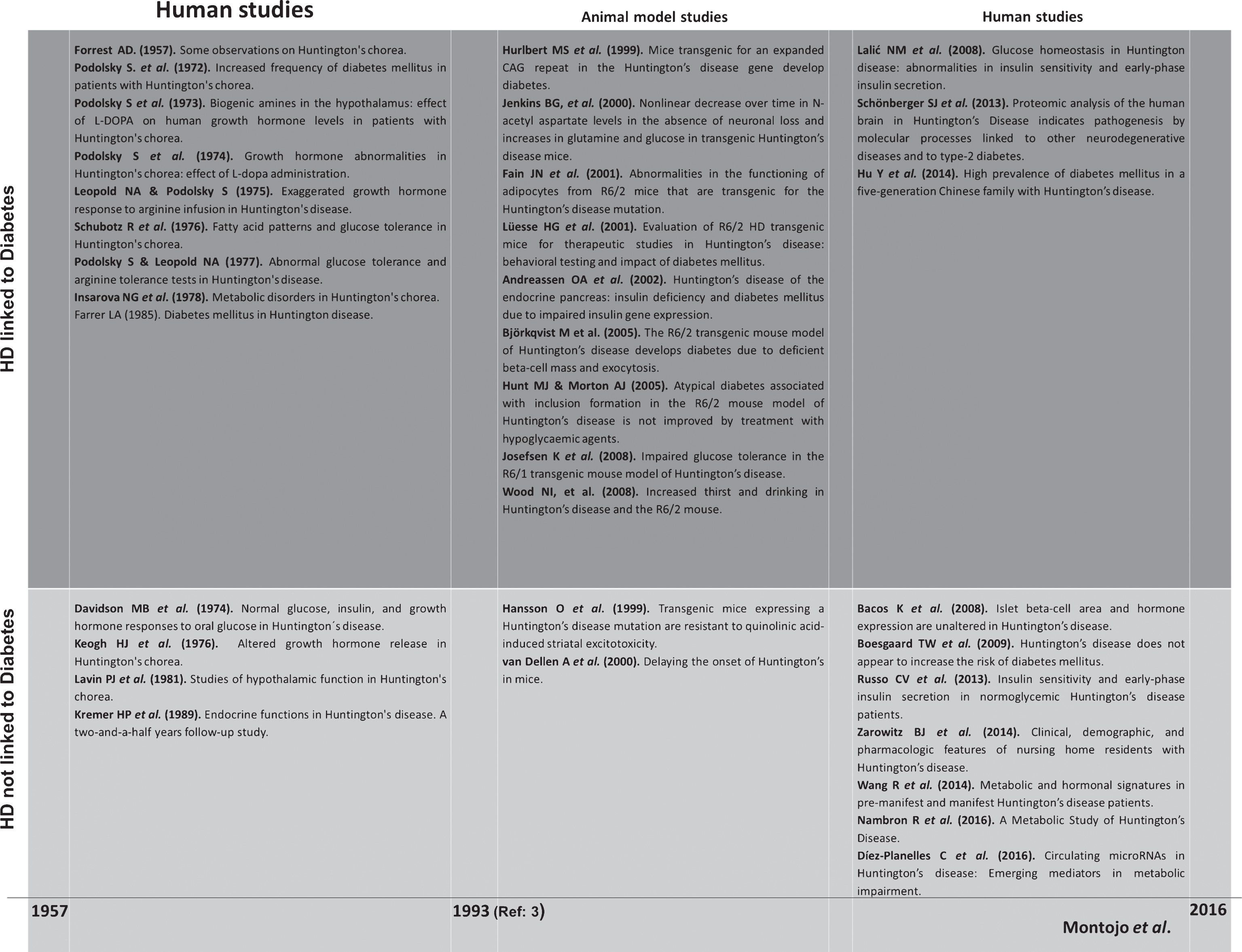
PRIOR TO THE IDENTIFICATION OF THE HD GENE (1957–1993)
In 1957, Forrest observed an increase in hyperkinetic movements among HD patients before meals, relative to movements after meals, which led him to postulate that low fasting sugar levels might be related to the disease. The examination of fasting blood sugar in six patients displayed normal levels, though an abnormal insulin tolerance curve was found in one of the two individuals examined [26].
In the 1970s, Podolsky and Leopold documented a higher incidence of carbohydrate intolerance and atypical insulin secretion, without glycosuria or a tendency to ketosis in six of 10 patients with hereditary chorea, who were clearly diabetic by the criteria of Fajans and Conn [14]. The researchers also found an off-based release of human growth hormone (GH) and a paradoxical complete suppression of the GH levels after L-DOPA administration, when compared to healthy controls. Arginine infusion provoked an elevated insulin response in those patients with a diabetic oral glucose tolerance test (OGTT), and an exaggerated GH response in the majority of the individuals They attributed these findings to pathologic involvement of the hypothalamus [27, 28]. The work of Schubot and Insarova partially supported these results, also finding an increased cholesterol concentration in serum [29, 30].
In contrast, studying 11 HD patients and 11 age-matched controls, Davidson and collaborators observed no differences in fasting insulin levels, insulin response to glucose or circulating GH levels, thereby supporting the findings of Keogh et al., who found similar outcomes related to the OGTT and no evidence of altered insulin release. Nevertheless, there is a discrepancy between the two works. An earlier elevation of GH after insulin injection, which was attributable to a change in the dopamine was detected in HD patients by Keogh, while no differences were reported by Davidson [31, 32].
In 1981, Lavin examined several hypothalamic functions in eight HD patients compared to 10 controls. As found previously by Keogh, the study reported a cessation of choreiform movements (≈60 minutes) during the insulin tolerance test. The fasting blood glucose level and glucose response to insulin were similar. However, the response of plasma cortisol and of GH to hypoglycemia was earlier in the HD patients, though peak responses were the same. These findings could be due to enhanced serotonin activity rather than an increase in dopamine activity [31, 33]. In a follow-up study, the evaluation of endocrine-function parameters in eight HD patients, failed to detect any deterioration in glucose metabolism values or GH secretion 2.5 years later [24].
In the studies described above, the participants had not been diagnosed with diabetes. Interestingly, a higher prevalence of diabetes mellitus in patients with HD – specifically among the probands under 50 years of age–was reported by a retrospective epidemiologic study conducted by Farrer. The analysis of the data obtained through questionnaires from a total of 620 affected HD individuals – 288 living, 332 deceased–resulted in the identification of 65 subjects (10.5%) diagnosed as diabetic. In 45% of these subjects, glucose levels higher than 140 mg/dl or a physician's statement of diabetes were reported; 55% were verified by examination of medical records. However, no clarification of the type of diabetes was provided. In addition, information related to the probands first- and second-degree relatives suggested that the affected relatives of a diabetic HD proband were seven times as likely to have diabetes than the proband’s non-HD relatives [13].
The relationship between carbohydrate intolerance and HD was previously evoked even before the genetic era, though with controversial results. The discovery of the CAG expansion in exon 1 of the HTT gene as the cause of the disease, made it possible to refine diagnosis, and develop models used to undertake specific studies related to glucose metabolism [3].
HD ANIMAL MODELS, IN VITRO ANALYSIS, AND HUMAN STUDIES (1996–2016)
HD murine model studies
The development of HD transgenic-mice has generated models with different expansion sizes – HD-171-82Q mouse (82 CAGs repeats), HdhCAG(150), R6/1 and R6/2 generated using the same background mouse strain but different repeat sequence (≈115 and 140 up to >400, respectively)–, which showed many features of the HD neurological phenotype [34–38]. Increased plasma levels of glucose were observed in the nondiabetic HD-171-82Q mouse, while dysregulation of gene expression parallel to changes in brain and muscle were detected in HdhCAG(150) mice, suggesting a potential connection with metabolism [36, 37]. The R6/1 mice exhibited neurological symptoms after 22–26 weeks of age and displayed different signs of impaired glucose tolerance including abnormal glucose handling and higher glucose plasma insulin levels in the glucose challenge, but did not result in manifest diabetes due to normal peripheral insulin sensitivity [39]. The R6/1 mice developed an age-dependent reduction of β-cell volume (17% and 39% lower at 10 and 38 weeks-old, respectively, compared to age-matched wild-type littermates); these reductions were not associated with diminished pancreas volume. Additionally no signs of apoptosis, necrosis, or autoimmunity were detected in the pancreas, although in both α- and β-cells huntingtin was present [39]. Interestingly, fasting plasma glucose levels were identical to the values in wild-type mice, as described by different investigators [35, 40]. Based on these results, the R6/1 mice-model was nondiabetic.
The studies performed by Fain et al., in an R6/2 transgenic HD model (141–157 CAGs) consisting of animals of 5, 7 and 8 weeks of age, described enhanced propensity toward fat accumulation, accompanied by an increase in leptin release and serum leptin concentration. However, the animals responded normally to exogenous insulin and no differences in glucose levels were detected [41]. It is worth mentioning that other works found that around 2/3 of neurologically asymptomatic R6/2 animals (140 CAGs) at age 7 weeks exhibited higher blood glucose concentrations than age-matched controls. As the R6/2 mice developed resting tremor, irregular gait, and stereotypic and abrupt movements (9 weeks of age), polyuria was present and the severity of hyperglycemia increased in the already high-glucose-level mice, while the remaining mice were euglycemic. Moreover, pathological results were found in a glucose tolerance test after intraperitoneal injection of glucose in R6/2 mice, which exhibited no increase in blood glucose levels in diurnal profiles, suggesting latent diabetes mellitus [18, 21, 42]. Although at 12 weeks a decrease in glucose utilization is reported while a large elevation of glucose in the brain occurred, only a small number of HD animals developed manifest diabetes, and no significant differences were recorded in survival, weight loss, motor coordination, or spontaneous explorative behaviour, indicating that the metabolic dysfunction was not connected to early motor and cognitive dysfunctions, and did not correlate with motor impairment and survival [42, 43]. However, daily repeated exercise – a well-known reducer of the severity of diabetes–in the R6/2 model was the main contributing factor to onset of diabetes [21]. Stress is known to enhance hyperglycemia in altered metabolic states [44], and it induced the activation of the protein kinase (SAPK) pathway, which has been associated with impaired insulin secretion and insulin resistance [45]. Interestingly, the mutated huntingtin stimulated SAPK activity. This activation may represent a potential mechanism for which exposure of mice to chronic stress could induce diabetes [21]. In most R6/2 animals, although the development of diabetes was paralleled with the overt phenotype, diabetes is unlikely to be the cause of either the neurological phenotype or the rapid decline in general function [21]. Accordingly, plasmid vaccination against mutant huntingtin dramatically improved the diabetic phenotype without producing any overt changes in the behaviour phenotype [46].
When the number of CAGs increased in the R6/2 models – Lund colony (163–174) and Cambridge colony (247–277)– increased time drinking and volume ingested were observed at 10 weeks of age despite the difference in the length of the CAG expansion, likely as a consequence of hypothalamic dysfunction, but not due to the presence of diabetes [47]. In the Lund colony, increases in blood glucose levels and diabetes have not been reported below 12 weeks of age, while most in the Cambridge colony were diabetic by 18 weeks [47].
The diabetic states seem to be associated with the influence of the length of CAG expansion and the severity of the neurological symptoms. However, a paradoxical delay in the onset of the disease consisting of delayed appearance of neuronal intranuclear inclusions has been reported by Morton et al., who studied R6/2 mice carrying super-long expansions (350–399 and >400 CAGs repeats). The mice showed a prolonged survival, but not neuroprotective effects. Aspects related to glucose metabolism have not yet been evaluated in these HD murine models [38].
The evaluation of intranuclear inclusions, accumulated with aging in R6/2 pancreatic islets has been also described. Specifically, at three weeks of age, the nuclear inclusions could be identified either in the pancreas or neurons [48]; however, no signs of diabetes were detected. In six-week old R6/2 mice, at least 50% of pancreatic islet cells contained inclusions; while at 10 weeks, when an abnormal overt phenotype could be observed, inclusions were present in almost all pancreatic islet cells, and most mice were intolerant to a glucose bolus [21]. Corresponding to end-stage HD-murine model (around 12 weeks), a variety of studies based on pancreatic function have shown several common features, though also certain differences. Hulbert et al. reported reductions in pancreatic levels of glucagon, somatostatin, and insulin, though the islet appearance was normal [18]. As expected, dramatic reductions in the number of insulin vesicles and insulin mRNA levels were also reported [49], potentially due to the diminished expression of key regulators of insulin gene transcription (i.e., the pancreatic homeoprotein PDX-1, E2A proteins, and the coactivators CBP and p300), which would be a possible explanation for insulin deficiency and consequent diabetes [50]. In contrast, Björkqvist et al., reported that the islets appeared hypotrophic, and β-cell mass was drastically reduced in R6/2 mice, while the islet number and level of glucagon were unaffected [20].
As previously described in R6/1 mice, no indications of increased cell death in islets from R6/2 were reported; rather, a marked decrease in the replication of β-cells was observed. This reflected an impairment in the replication of islet cells, which resulted in deficient β-cell mass, though this was not sufficient to induce diabetes in the mice [51]. A separate pathologic process is likely involved. Björkqvist et al. also described that exocytosis was abolished in β-cells, and that β-cells were more prone to huntingtin accumulation than the rest of islet cell types, thus leading to a loss of hormone-containing secretory granules, which abolished stimulated insulin secretion [20]. A relevant role for the maintenance of pancreatic β-cell function was played by the insulin receptor substrate-2 (IRS-2) signalling. In fact IRS-2 deletion in pancreatic β-cells caused diabetes, though a reduced IRS-2 signalling slowed lethality in an HD-model and also protected against oxidative stress, mitochondrial dysfunction and neuropathology [52].
Treatments with hypoglycemic agents in HD murine models
The previously described link, between HD-murine models and glucose metabolic alterations, has been used for the exploration of therapeutic approaches to HD based on the effects of different hypoglycemic agents. As summarized in Table 1, those compounds induced different effects on the metabolic system (i.e., plasma glucose and insulin level, insulin sensitivity, and pancreatic morphology), and their actions were also evaluated in classical HD-features such as motor coordination and survival.
Table 1
Treatments with hypoglycemic agents in HD murine models
Weight loss | Plasma glucose level | Plasma insulin level | Insulin sensitivity | Pancreatic morphology | Motor coordination | Life span | Murine model | |
Glibenclamide | No modification | Reduced | – | – | – | No modification | No modification | R6/2 |
Rosiglitazone | No modification | No modification | – | – | – | No modification | No modification | R6/2 |
Metformin | No modification | No modification | – | – | – | Partial improvement | Increased 20.1% in males | R6/2 |
Exenatide | Increased | Reduced | Reduced | Increased | Significant improvement | Significant improvement | Increased 18% in males | N171-82Q |
GLP-1-Tf | No modification | Reduced | Increased | – | Significant improvement | Partial improvement | Increased 17% in males | N171-82Q |
Insulin | No modification | No modification | Increased | – | No modification | Reduced | No modification | N171-82Q |
Resveratrol | No modification | Reduced | – | – | – | No modification | No modification | N171-82Q |
–: No Data. Table compiled information from references [23, 63, 64, 67]. Glibenclamide – sulfonylurea, which depolarizes pancreatic β-cells by blocking ATP-sensitive potassium channels causing exocytosis of insulin–; Rosiglitazone – increases insulin sensitivity by activating the peroxisome proliferator-activated receptor γ–; metformin – a widely antidiabetic drug, and also, an inducer of insulin sensitization among other positive effects on glucose transport and hepatic glucose synthesis–; Exenatide – antidiabetic glucagon-like peptide (GLP-1) receptor agonist (58)–; GLP-1Tf – a fusion protein made up of GLP-1 and nonglycosylated form of human transferrin–; Insulin – the basic treatment for type 1 diabetes, and frequently used in long standing T2D to achieve adequate glycemic control–; and resveratrol – a naturally occurring polyphenolic compound, which targets SIRT1 (also known as NAD-dependent deacetylase sirtuin-1) protein involved in metabolic actions.
The treatments administered to R6/2 or N171-82Q mice were glibenclamide in combination with rosiglitazone, metformin, exenatide, insulin, GLP-1Tf, and resveratrol [53–55]. With the exception of exenatide, none of the compounds tested modified the extreme and uncontrolled weight loss observed in the HD model during the disease progression. Exenatide treatment exacerbated the reduction, as previously described in human subjects and animal models [56–58]. With regard to the blood glucose levels, a significant decrease was observed after the administration of glibenclamide/rosiglitazone, exenatide, GLP-1-Tf, and resveratrol, but not metformin [59]. The lack of glucose-level reduction by rosiglitazone alone suggests that insulin resistance is not a primary factor in diabetes occurring in HD mice [21]. Exenatide and GLP-1Tf were able to reduce glucose levels; however, the insulin treatment had no effect on the N171-82Q model, as opposed to the effects observed in R6/2 mice [18, 60]. Only exenatide administration reduced plasma insulin level and increased the insulin sensitivity; and both insulin and GLP-1-Tf also induced increases in insulin levels, though no information related to sensitivity to the hormone was available [60]. Neither glibenclamide, rosiglitazone nor resveratrol treatment influenced motor coordination in the animals. However, a 2-mg/ml dose of metformin decreased hind limb clasping time, while exenatide produced a reversal of motor performance decline, and actually induced a significant increment in the ability to remain on the Rota-Rod. GLP-1Tf-treatment partially attenuated performance loss in HD mice, though unlike exenatide, failed to enhance performance score. In the case of insulin administration, the hormone worsened the degree of progressive decline in motor performance, exerting no influence on life span. Nonetheless, metformin, exeantide, and GLP-1t induced a significant increase of survival (20%, 18 and 17, respectively). Both the exenatide and GLP-1Tf treatments, in the HD-transgenic model, restored close to normalization the islet size and numbers of β-cells, and also the α-cell topography of islets; while no positive modification in pancreatic morphology was induced by insulin treatment [56]. Exenatide – approved for the treatment of type-2 diabetes (T2D)–was the most beneficial therapy for diabetic and HD features (Table 1).
Large animal model studies
The value added by the large HD models – sheep, minipigs and non-human primate models- has been significant for therapeutic strategies. Recently, the plasma profiling of presymptomatic HD sheep identified significant changes in 89 metabolites compared to controls affecting amino acids, biogenic amines, acylcarnitines, sphingolipids, glycerophospholipids, and urea, though no diabetic phenotype was reported [61].
In vitro analysis
The expression of mutant huntingtin in human insulin-expressing 8321/13 beta-cells slowed intracellular trafficking, reduced the number of rapidly moving vesicles and decreased stimulated insulin release. This less efficient intracellular transport could be explained by the fact that β-tubulin interaction was stronger with mutant than with wild-type huntingtin. The mutant protein by binding the microtubules became a physical block for microtubule-dependent motors [49]. The experiments performed by Edwardson et al., in a PC12 cell line stably expressing the first exon of huntingtin with 74 CAG repeats, demonstrated an impaired expression of the exocytotic protein complexin II, which in combination with the deficient β-cells observed in the HD model could explain the development of diabetes [19, 62]. Interestingly, the glucose-stimulated induction of insulin release was significantly increased in the insulinoma cell line (INS-1E), expressing huntingtin with elongated polyglutamine stretches similar to the human range (18 and 51 CAGs, respectively) compared to cells expressing highly expanded huntingtin – 115 CAGs–.
Human studies
Lalic et al. reported significantly higher insulin levels in 29 normoglycemic patients with clinically and genetically verified late-stage HD – without prescribed medication–, compared to 22 matched controls. No differences in plasma glucose levels were observed during the OGTT [63]. Contrary to this study, three works with comparable numbers of subjects found equal fasting glucose and insulin levels in pre-manifest and manifest HD patients compared to controls. A blunted insulin response has been detected in advanced HD patients, though the difference did not achieve statistical significance. Although one of the studies reported glucose regulation abnormalities (i.e., flat glucose curve and delayed insulin peak after oral glucose load) there was no significant difference in homeostatic model assessment in the evaluated groups. The GH levels were decreased in manifest HD subjects, and also associated with clinical severity, whereas elevated ghrelin values were only reported in the premanifest-group [23, 64–66]. Diminished fasting and postprandial leptin levels were revealed in manifest HD patients by Wang et al., but not by Nambrom and collaborators [23, 64]. The disparity among studies may stem from difficulties associated with measuring metabolism in humans – food intake, lighting conditions, sleep/wake routine, and sampling-settings–which influence metabolic profile [64, 67, 68]. Recently, increased levels of microRNAs related to cholesterol metabolism, have been found in HD patients, pointing to a possible impairment of this pathway, though none related to glucose metabolism [69]. However, the proteomic analysis of seven HD human brains identified, in the middle frontal gyrus, and in the visual cortex, twenty-nine proteins differentially expressed compared to matched controls. Most of the proteins play roles in cell stress responses, apoptosis, the ubiquitin-proteasome system, protein trafficking/endocytosis or metabolic regulation linked to T2D [70].
Regarding pancreatic related studies, Boesgaard et al. did not described any major β-cell defect in any of the 14 adult-onset-patients with typical HD-symptoms (36 up to 51 CAGs) [25]. Moreover, a morphological study performed in the pancreas of HD patients reported that immunostaining patterns for the main islet hormones and islet β-cells, and levels of insulin transcripts were unchanged. Compared to controls, no differences in islet β-cell area, extracellular amyloid deposits, or inclusions in either endocrine or exocrine pancreatic cells was detected [71].
The prevalence of HD in residents of US skilled nursing facilities revealed that of a total of 249,811 residents, there were 340 (0.14%) with a diagnosis of HD, and only one (0.003%) showed diabetes (type of diabetes not reported) as a concomitant illness [22]; however, many HD individuals were on medications such as olanzapine (25.7%), which has been involved in the onset of diabetes in susceptible patients [72].
Interestingly, in Asia, where the prevalence of HD is significantly lower, a five-generation Chinese family (30 members) included eight members afflicted by both HD and T2D. The prevalence of diabetes in HD subjects (8/11) was drastically high (72.7%), while the prevalence of diabetes (13/30) was much higher than the estimated 3.9% reported in China in 2009. No data were available concerning the influence of antidiabetic treatment (metformin alone or in combination with insulin) in promoting or delaying the symptoms of HD [73].
A curious case reported the onset of HD symptoms in a patient with diabetes and 39 CAGs, at least 7 years earlier than her identical monozygotic twin. It was suggested that the disease may have been initiated by environmental factors, which in this case were quite significant, though diabetes was not a major contributor to the HD phenotype [74]. On the other hand, although hyperglycemia-induced-chorea-ballism is predominantly observed in T2D and very rare in type 1 diabetes (T1D) and diabetic ketoacidosis (DKA) a unique case of HD un-masked by an episode of chorea-ballism associated with DKA and T1D also has been reported [75].
In addition to HD, other neurodegenerative genetic syndromes based on trinucleotide repeat expanded mutation, have also been linked to glucose metabolic alterations and diabetes including Friedreich ataxia (FA), myotonic dystrophy 1 (MD1) and spinocerebellar ataxia (SCA), type 1, 3 and 6 [15, 76]. Although insulin resistance and altered insulin secretion were reported in patients diagnosed with FA, MD1 and SCA1, impaired mitochondrial function – a potential feature of common T2D–was only detected in FA and MD1. A closer examination of the available bibliography revealed numerous articles related to metabolic aspects in FA [15], several of which clearly support the presence of diabetes, while others show inconclusive results [77]. However, the information on SCA is scant, and it should be noted that no association with metabolic alterations has been reported in fragile X syndrome, Kennedy's disease or dentatorubral-pallidoluysian atrophy (DRPLA). However, strong evidence demonstrated a relevant link of diabetes with Alzheimer's disease, Down or Turner syndrome, which are not associate with a triplet expansion mutation [15].
CONCLUSION
Based on several early human studies, and partially supported by the later murine-model assays, HD is associated with glucose alterations and diabetes, as occurs in other neurological diseases. However, the studies conducted before the HD-genetic diagnosis provided limited outcomes due to a very low number of participants, lack of clinical information and, potentially, an uncertain diagnosis. In addition, although the works carried out in HD-mice models reveal a significant association with glucose metabolism alterations and β-cell dysfunction, the development of diabetes was not indicated unanimously, being also irregular, the effects displayed by the hypoglycemic agents. Conversely, in the most recent publications with clinically and genetically substantiated HD participants, a very low prevalence of diabetes was recorded and no statistical differences in glucose metabolism related indexes or pancreas morphology were found, with the exception of one study performed in a well-characterized cohort of late-stage HD with a low BMI and normoglycemic levels. Follow-up of these patients would have provided useful information on the evolution of their glucose homeostatic parameters and the establishment, or not, of a diabetic state; however, the metabolic disturbances described in premanifest and manifest HD subjects appear not to translate to a clear diabetic phenotype.
The estimated percentage of diagnosed and undiagnosed diabetes in the general population among adults aged 45–65 years is 12,7% (National Diabetes Statistics Report, 2017; National Institutes of Health). To date, it seems that diabetes is less frequent in the HD population.
In this scenario, further approaches in humanized mouse models – resembling human HD–, large animal models such as sheep, minipigs and non-human primates – considering species limitations–along with human studies performed in larger samples with an exhaustive follow-up, may be useful for examining whether impaired glucose metabolism contributes to the development of HD and complicates efforts to treat the patients. There is also a need to obtain reliable statistics of diabetes prevalence in this neurological disease.
CONFLICT OF INTEREST
The authors declare that there is no conflict of interest that would prejudice the impartiality of the reported research.
ACKNOWLEDGMENTS
The authors thank Oliver Shaw for editing the manuscript. Nieves González is supported by Instituto de Salud Carlos III (MSII14/00031).
REFERENCES
[1] | Huntington G . On chorea. Med Surg Report Philadelphia. (1872) ;26: :317–21. |
[2] | Rüb U , Vonsattel JP , Heinsen H , Korf HW . The neuropathology of Huntington's disease: Classical findings, recent developments and correlation to functional neuroanatomy. Adv Anat Embryol Cell Biol. (2015) ;217: :1–146. |
[3] | A novel gene containing a trinucleotide repeat that is expanded and unstable on Huntington’s disease chromosomes. The Huntington’s Disease Collaborative Research Group. Cell. (1993) ;72: :971–83. (http://www.ncbi.nlm.nih.gov/pubmed/8458085) (accessed June 14, 2016). |
[4] | Davies SW , Turmaine M , Cozens BA , DiFiglia M , Sharp AH , Ross CA , et al. Formation of neuronal intranuclear inclusions underlies the neurological dysfunction in mice transgenic for the HD mutation. Cell. (1997) ;90: :537–48. |
[5] | Genetic Modifiers of Huntington’s Disease (GeM-HD) Consortium. Identification of Genetic factors that modify clinical onset of Huntington’s disease. Cell. (2015) ;162: :516–26. |
[6] | Hubers AA , van Duijn E , Roos RA , Craufurd D , Rickards H , Bernhard Landwehrmeyer G , et al. Suicidal ideation in a European Huntington’s disease population. J Affect Disord. (2013) ;151: :248–58. |
[7] | Shannon KM . Pridopidine for the treatment of Huntington’s disease. Expert Opin Investig Drugs. (2016) ;25: :485–92. |
[8] | Sathasivam K , Hobbs C , Turmaine M , Mangiarini L , Mahal A , Bertaux F , et al. Formation of polyglutamine inclusions in non-CNS tissue. Hum Mol Genet. (1999) ;8: :813–22. |
[9] | Morea V , Bidollari E , Colotti G , Fiorillo A , Rosati J , De Filippis L , et al. Glucose transportation in the brain and its impairment in Huntington disease: One more shade of the energetic metabolism failure? Amino Acids. (2017) ;49: :1147–57. |
[10] | Browne SE , Beal MF . Oxidative damage in Huntington’s disease pathogenesis. Antioxid Redox Signal. (2006) ;8: (11-12):2061–73. |
[11] | Petersén A , Björkqvist M . Hypothalamic-endocrine aspects in Huntington’s disease. Eur J Neurosci. (2006) ;24: :961–7. |
[12] | Saleh N , Moutereau S , Durr A , Krystkowiak P , Azulay JP , Tranchant C , et al. Neuroendocrine disturbances in Huntington’s disease. PLoS One. (2009) ; 4 e4962. |
[13] | Farrer LA . Diabetes mellitus in Huntington disease. Clin Genet. (1985) ;27: :62–7. |
[14] | Podolsky S , Leopold NA , Sax DS . Increased frequency of diabetes mellitus in patients with Huntington’s chorea. Lancet. (1972) ;1: :1356–8. |
[15] | Ristow M . Neurodegenerative disorders associated with diabetes mellitus. J Mol Med. (2004) ;82: :510–29. |
[16] | Leibson CL , Rocca WA , Hanson VA , Cha R , Kokmen E , O’Brien PC , et al. Risk of dementia among persons with diabetes mellitus: A population-based cohort study. Am J Epidemiol. (1997) ;145: :301–8. |
[17] | Höybye C , Hilding A , Jacobsson H , Thorén M . Metabolic profile and body composition in adults with Prader-Willi syndrome and severe obesity. J Clin Endocrinol Metab. (2002) ;87: :3590–7. |
[18] | Hurlbert MS , Zhou W , Wasmeier C , Kaddis FG , Hutton JC , Freed CR . Mice transgenic for an expanded CAG repeat in the Huntington’s disease gene develop diabetes. Diabetes. (1999) ;48: :649–51. |
[19] | Andreassen OA , Dedeoglu A , Stanojevic V , Hughes DB , Browne SE , Leech CA , et al. Huntington’s disease of the endocrine pancreas: Insulin deficiency and diabetes mellitus due to impaired insulin gene expression. Neurobiol Dis. (2002) ;11: :410–24. |
[20] | Björkqvist M , Fex M , Renström E , Wierup N , Petersén A , Gil J , et al. The R6/2 transgenic mouse model of Huntington’s disease develops diabetes due to deficient beta-cell mass and exocytosis. Hum Mol Genet. (2005) ;14: :565–74. |
[21] | Hunt MJ , Morton AJ . Atypical diabetes associated with inclusion formation in the R6/2 mouse model of Huntington’s disease is not improved by treatment with hypoglycaemic agents. Exp Brain Res. (2005) ;166: :220–9. |
[22] | Zarowitz BJ , O’Shea T , Nance M . Clinical, demographic, and pharmacologic features of nursing home residents with Huntington’s disease. J Am Med Dir Assoc. (2014) ;15: :423–8. |
[23] | Wang R , Ross CA , Cai H , Cong WN , Daimon CM , Carlson OD , et al. Metabolic and hormonal signatures in pre-manifest and manifest Huntington’s disease patients. Front Physiol. (2014) ;5: :231. |
[24] | Kremer HP , Roos RA , Frölich M , Radder JK , Kruseman N , Van der Velde A , et al. Endocrine functions in Huntington’s disease. A two-and-a-half years follow-up study. J Neurol Sci. (1989) ;90: :335–44. |
[25] | Boesgaard TW , Nielsen TT , Josefsen K , Hansen T , Jørgensen T , Pedersen O , et al. Huntington’s disease does not appear to increase the risk of diabetes mellitus. J Neuroendocrinol. (2009) ;21: :770–6. |
[26] | Forrest AD . Some observations on Huntington’s chorea. J Ment Sci. (1957) ;103: :507–13. |
[27] | Podolsky S , Leopold NA . Biogenic amines in the hypothalamus: Effect of L-DOPA on human growth hormone levels in patients with Huntington’s chorea. Prog Brain Res. (1973) ;39: :225–35. |
[28] | Podolsky S , Leopold NA . Growth hormone abnormalities in Huntington’s chorea: Effect of L-dopa administration. J Clin Endocrinol Metab. (1974) ;39: :36–9. |
[29] | Insarova NG , Korshunova TS , Mzhel’skaia TI , Borisova TV . Metabolic disorders in Huntington’s chorea. Zh Nevropatol Psikhiatr Im S S Korsakova. (1978) ;78: :489–95. |
[30] | Schubotz R , Hausmann L , Kaffarnik H , Zehner J , Oepen H . Fatty acid patterns and glucose tolerance in Huntington’s chorea. Res Exp Med (Berl). (1976) ;167: :203–15. |
[31] | Keogh HJ , Johnson RH , Nanda RN , Sulaiman WR . Altered growth hormone release in Huntington’s chorea. J Neurol Neurosur Psychiatry. (1976) ;39: :244–8. |
[32] | Davidson MJ , Green MB . Normal glucose, insulin, and growth hormone responses to oral glucose in Huntington's disease. J Lab Clin Med. (1974) ;81: :807–12. |
[33] | Lavin PJ , Bone I , Sheridan P . Studies of hypothalamic function in Huntington’s chorea. J Neurol Neurosurg Psychiatry. (1981) ;44: :414–8. |
[34] | Mangiarini L , Sathasiva K , Seller M , Cozens B , Harper A , Hetherington C , et al. Exon 1 of the HD gene with an expanded CAG repeat is sufficient to cause a progressive neurological phenotype in transgenic mice. Cell. (1996) ;87: :493–506. |
[35] | Hansson O , Petersén A , Leist M , Nicotera P , Castilho RF , Brundin P . Transgenic mice expressing a Huntington’s disease mutation are resistant to quinolinic acid-induced striatal excitotoxicity. Proc Natl Acad Sci USA. (1999) ;96: :8727–32. |
[36] | Duan W , Guo Z , Jiang H , Ware M , Li XJ , Mattson MP . Dietary restriction normalizes glucose metabolism and BDNF levels, slows disease progression, and increases survival in huntingtin mutant mice. Proc Natl Acad Sci USA. (2003) ;100: :2911–6. |
[37] | Luthi-Carter R , Hanson SA , Strand AD , Bergstrom DA , Chun W , Peters NL , et al. Dysregulation of gene expression in the R6/2 model of polyglutamine disease: Parallel changes in muscle and brain. Hum Mol Genet. (2002) ;11: :1911–26. |
[38] | Morton AJ , Glynn D , Leavens W , Zheng Z , Faull RLM , Skepper JN , et al. Paradoxical delay in the onset of disease caused by super-long CAG repeat expansions in R6/2 mice. Neurobiol Dis. (2009) ;33: :331–41. |
[39] | Josefsen K , Nielsen MD , Jørgensen KH , Bock T , Nørremølle A , Sørensen SA , et al. Impaired glucose tolerance in the R6/1 transgenic mouse model of Huntington’s disease. J Neuroendocrinol. (2008) ;20: :165–72. |
[40] | van Dellen A , Blakemore C , Deacon R , York D , Hannan AJ . Delaying the onset of Huntington’s in mice. Nature. (2000) ;404: :721–2. |
[41] | Fain JN , Del Mar NA , Meade CA , Reiner A , Goldowitz D . Abnormalities in the functioning of adipocytes from R6/2 mice that are transgenic for the Huntington’s disease mutation. Hum Mol Genet. (2001) ;10: :145–52. |
[42] | Lüesse HG , Schiefer J , Spruenken A , Puls C , Block F , Kosinski CM . Evaluation of R6/2 HD transgenic mice for therapeutic studies in Huntington’s disease: Behavioral testing and impact of diabetes mellitus. Behav Brain Res. (2001) ;126: :185–95. |
[43] | Jenkins BG , Klivenyi P , Kustermann E , Andreassen OA , Ferrante RJ , Rosen BR , et al. Nonlinear decrease over time in N-acetyl aspartate levels in the absence of neuronal loss and increases in glutamine and glucose in transgenic Huntington’s disease mice. J Neurochem. (2000) ;74: :2108–19. |
[44] | Surwit RS , Schneider MS , Feinglos MN . Stress and diabetes mellitus. Diabetes Care. (1992) ;15: :1413–22. |
[45] | Evans JL , Goldfine ID , Maddux BA , Grodsky GM . Oxidative stress and stress-activated signaling pathways: A unifying hypothesis of type 2 diabetes. Endocr Rev. (2002) ;23: :599–622. |
[46] | Miller TW , Shirley TL , Wolfgang WJ , Kang X , Messer A . DNA vaccination against mutant huntingtin ameliorates the HDR6/2 diabetic phenotype. Mol Ther. (2003) ;7: :572–9. |
[47] | Wood NI , Goodman AOG , van der Burg JMM , Gazeau V , Brundin P , Björkqvist M , et al. Increased thirst and drinking in Huntington’s disease and the R6/2 mouse. Brain Res Bull. (2008) ;76: :70–9. |
[48] | Morton AJ , Lagan MA , Skepper JN , Dunnett SB . Progressive formation of inclusions in the striatum and hippocampus of mice transgenic for the human Huntington’s disease mutation. J Neurocytol. (2000) ;29: :679–702. |
[49] | Smith R , Bacos K , Fedele V , Soulet D , Walz HA , Obermüller S , et al. Mutant huntingtin interacts with beta-tubulin and disrupts vesicular transport and insulin secretion. Hum Mol Genet. (2009) ;18: :3942–54. |
[50] | Andreassen OA , Ferrante RJ , Huang HM , Dedeoglu A , Park L , Ferrante KL , et al. Dichloroacetate exerts therapeutic effects in transgenic mouse models of Huntington’s disease. Ann Neurol. (2001) ;50: :112–7. |
[51] | Weir GC , Laybutt DR , Kaneto H , Bonner-Weir S , Sharma A . Beta-cell adaptation and decompensation during the progression of diabetes. Diabetes. (2001) ;50: (Suppl 1):S154–9. h |
[52] | Sadagurski M , Cheng Z , Rozz A , Palazzolo I , Kelley GR , Dong X , et al. IRS2 increases mitochondrial dysfunction and oxidative stress in a mouse model of Huntington disease. J Clin Invest. (2011) ;121: :4070–81. |
[53] | Kim BJ , Zhou J , Martin B , Carlson OD , Maudsley S , Greig NH , et al. Transferrin fusion technology: A novel approach to prolonging biological half-life of insulinotropic peptides. J Pharmacol Exp Ther. (2010) ;334: :682–92. |
[54] | Ho DJ , Calingasan NY , Wille E , Dumont M , Beal MF . Resveratrol protects against peripheral deficits in a mouse model of Huntington’s disease. Exp Neurol. (2010) ;225: :74–84. |
[55] | Chaudhury A , Duvoor C , Reddy-Dendi VS , Kraleti S , Chada A , Ravilla R , et al. Clinical review of antidiabetic drugs: Implications for type 2 diabetes mellitus management. Front Endocrinol. (2017) ;8: :6. |
[56] | Martin B , Golden E , Carlson OD , Pistell P , Zhou J , Kim W , et al. Exendin-4 improves glycemic control, ameliorates brain and pancreatic pathologies, and extends survival in a mouse model of Huntington’s disease. Diabetes. (2009) ;58: :318–28. |
[57] | Egan JM , Clocquet AR , Elahi D . The insulinotropic effect of acute exendin-4 administered to humans: Comparison of nondiabetic state to type 2 diabetes. J Clin Endocrinol Metab. (2002) ;87: :1282–90. |
[58] | Elfers CT , Simmons JH , Roth CL . Glucagon-like peptide-1 agonist exendin-4 leads to reduction of weight and caloric intake in a rat model of hypothalamic obesity. Horm Res. Pædiatrics. (2012) ;78: :47–53. |
[59] | Ma TC , Buescher JL , Oatis B , Funk JA , Nash AJ , Carrier RL , et al. Metformin therapy in a transgenic mouse model of Huntington’s disease. Neurosci Lett. (2007) ;411: :98–103. |
[60] | Martin B , Chadwick W , Cong W , Pantaleo N , Daimon CM , Golden EJ , et al. Euglycemic agent-mediated hypothalamic transcriptomic manipulation in the N171-82Q model of Huntington disease is related to their physiological efficacy. J Biol Chem. (2012) ;287: :31766–82. |
[61] | Skene DJ , Middleton B , Fraser CK , Pennings JLA , Kuchel TR , Rudiger RS , et al. Metabolic profiling of presymptomatic Huntington’s disease sheep reveals novel biomarkers. Sci Rep. (2017) ;7: :43030. |
[62] | Edwardson JM , Wang CT , Gong B , Wyttenbach A , Bai J , Jackson MB , et al. Expression of mutant huntingtin blocks exocytosis in PC12 cells by depletion of complexin II. J Biol Chem. (2003) ;278: :30849–53. |
[63] | Lalić NM , Marić J , Svetel M , Jotić A , Stefanova E , Lalić K , et al. Glucose homeostasis in Huntington disease: Abnormalities in insulin sensitivity and early-phase insulin secretion. Arch Neurol. (2008) ;65: :476–80. |
[64] | Nambron R , Silajdžić E , Kalliolia E , Ottolenghi C , Hindmarsh P , Hill NR , et al. A Metabolic study of Huntington’s disease. PLoS One. (2016) ;11: :e0146480. |
[65] | Russo CV , Salvatore E , Saccá F , Tucci T , Rinaldi C , Sorrentino P , et al. Insulin sensitivity and early-phase insulin secretion in normoglycemic Huntington’s disease patients. J Huntingtons Dis. (2013) ;2: :501–7. |
[66] | Aziz NA , Pijl H , Frölich M , Schröder-van der Elst JP , van der Bent C , et al. Growth hormone and ghrelin secretion are associated with clinical severity in Huntington’s disease. Eur J Neurol. (2010) ;17: :280–8. |
[67] | Davies SK , Ang JE , Revell VL , Holmes B , Mann A , Robertson FP , et al. Effect of sleep deprivation on the human metabolome. Proc Natl Acad Sci USA. (2014) ;111: :10761–6. |
[68] | Cheng ML , Chang KH , Wu YR , Chen CM . Metabolic disturbances in plasma as biomarkers for Huntington’s disease. J Nut. Biochem. (2016) ;31: :38–44. |
[69] | Díez-Planelles C , Sánchez-Lozano P , Crespo MC , Gil-Zamorano J , Ribacoba R , González N , et al. Circulating microRNAs in Huntington’s disease: Emerging mediators in metabolic impairment. Pharmacol Res. (2016) ;108: :102–10. |
[70] | Schönberger SJ , Jezdic D , Faull RLM , Cooper GJS . Proteomic analysis of the human brain in Huntington’s Disease indicates pathogenesis by molecular processes linked to other neurodegenerative diseases and to type-2 diabetes. J Huntingtons Dis. (2013) ;2: :89–99. |
[71] | Bacos K , Björkqvist M , Petersén A , Luts L , Maat-Schieman MLC , Roos RAC , et al. Islet beta-cell area and hormone expression are unaltered in Huntington’s disease. Histochem Cell Biol. (2008) ;129: :623–9. |
[72] | Koller EA , Doraiswamy PM . Olanzapine-associated diabetes mellitus. Pharmacotherapy. (2002) ;22: :841–52. |
[73] | Hu Y , Liang J , Yu S . High prevalence of diabetes mellitus in a five-generation Chinese family with Huntington’s disease. J Alzheimers Dis. (2014) ;40: :863–8. |
[74] | Friedman JH , Trieschmann ME , Myers RH , Fernandez HH . Monozygotic twins discordant for Huntington disease after 7 years. Arch Neurol. (2005) ;62: :995–7. |
[75] | Hashimoto K , Ito Y , Tanahashi H , Hayashi M , Yamakita N , Yasuda K . Hyperglycemic chorea-ballism or acute exacerbation of Huntington’s chorea? Huntington’s disease unmasked by diabetic ketoacidosis in type 1 diabetes mellitus. J Clin Endocrinol Metab. (2012) ;97: :3016–20. |
[76] | Lalić NM , Dragasević N , Stefanova E , Jotić A , Lalić K , Milicić T , et al. Impaired insulin sensitivity and secretion in normoglycemic patients with spinocerebellar ataxia type 1. Mov. Disord. (2010) ;25: :1976–80. |
[77] | Dupont S , Dubois D , Vionnet N , Boitard C , Caillat-Zucman S , Timsit J , et al. No association between the Friedreich’s ataxia gene and NIDDM in the French population. Diabetes. (1998) ;47: :1654–6. |