Necroptosis and Alzheimer’s Disease: Pathogenic Mechanisms and Therapeutic Opportunities
Abstract
Alzheimer’s disease (AD) is considered to be the most common neurodegenerative disease, with clinical symptoms encompassing progressive memory loss and cognitive impairment. Necroptosis is a form of programmed necrosis that promotes cell death and neuroinflammation, which further mediates the pathogenesis of several neurodegenerative diseases, especially AD. Current evidence has strongly suggested that necroptosis is activated in AD brains, resulting in neuronal death and cognitive impairment. We searched the PubMed database, screening all articles published before September 28, 2022 related to necroptosis in the context of AD pathology. The keywords in the search included: “necroptosis”, “Alzheimer’s disease”, “signaling pathways”, “Aβ”, Aβo”, “Tau”, “p-Tau”, “neuronal death”, “BBB damage”, “neuroinflammation”, “microglia”, “mitochondrial dysfunction”, “granulovacuolar degeneration”, “synaptic loss”, “axonal degeneration”, “Nec-1”, “Nec-1s”, “GSK872”, “NSA”, “OGA”, “RIPK1”, “RIPK3”, and “MLKL”. Results show that necroptosis has been involved in multiple pathological processes of AD, including amyloid-β aggregation, Tau accumulation, neuronal death, and blood-brain barrier damage, etc. More importantly, existing research on AD necroptosis interventions, including drug intervention and potential gene targets, as well as its current clinical development status, was discussed. Finally, the issues pertaining to necroptosis in AD were presented. Accordingly, this review may provide further insight into clinical perspectives and challenges for the future treatment of AD by targeting the necroptosis pathway.
INTRODUCTION
Alzheimer’s disease (AD), which is known to be the most common chronic neurodegenerative disease, clinically manifests as progressive memory loss, and cognitive impairment, which ultimately affects behavior, speech, visuospatial orientation, and the motor systems [1]. According to the World Alzheimer’s Disease Report, by 2050, 115 million people worldwide have been predicted to suffer from dementia, placing a heavy burden on societies and families [2]. To date, no effective treatment to cure or slow the progression of the disease exist. Neuropathologically, AD is characterized by the extracellular amyloid-β (Aβ) deposition, intracellular hyperphosphorylated Tau (p-Tau) formation of neurofibrillary tangles (NFTs), significant neuronal loss, and severe neuroinflammation [3–6]. However, the underlying mechanisms of these pathological processes have yet to be clarified. In light of recent research progress, the relationship between these pathological processes and necroptosis has garnered increased attention from scientists.
Necroptosis cell morphology resembles necrosis and is characterized by the loss of plasma membrane integrity, organelle swelling, chromatin fragmentation, and late cell lysis [7]. The execution of necroptosis involves three core components, namely receptor-interacting protein kinases (RIPK)1, RIPK3, and mixed lineage kinase domain-like protein (MLKL). Death receptors (DRs) activate RIPK1 kinase activity under the conditions of caspase inhibition, which alongside RIPK3 heterodimerization form amyloid-structured necrosomes and activate RIPK3 via phosphorylation. Activated RIPK3 subsequently recruits and phosphorylates MLKL, which leads to oligomerization and translocation of MLKL as well as the disruption of the plasma membrane, ultimately leading to necroptosis [8]. Currently, numerous studies have evidenced the involvement of necroptosis in AD pathology both in vitro and in vivo [9], which involved Aβ aggregation [10, 11], p-Tau and NFTs [12], neuronal death [8], blood-brain barrier (BBB) damage [13], granulovacuolar degeneration (GVD) [14], synaptic loss [15], restricted O-linked β-N-acetylglucosaminylation (O-GlcNAcylation) [16, 17], mitochondrial impairment [18], and neuroinflammation [19] leading to subsequent cognitive impairment [8]. In addition, the pharmacological and genetic inhibition of RIPK1/3 and MLKL have been shown to effectively ameliorate the pathological changes and cognitive deficits in associated AD models [10, 11, 19, 20].
The present review attempts to discuss the pathway that activates necroptosis and describes the mechanism of action of MLKL as an executor of necroptosis. Next, the mechanism by which necroptosis is involved in the corresponding pathological changes in AD are explained. More importantly, existing research pertaining to AD necroptosis intervention are summarized, including drug intervention and potential gene targets, as well as the current clinical development status. Finally, issues that warrant further discussion in necroptosis in AD are presented. The findings of this review may offer insight into the clinical perspectives and challenges involved in the future treatment of AD by targeting the necroptosis pathway.
METHODS
Literature retrieval and analysis were conducted in the PubMed database. The key words included a single search and combination search of “necroptosis”, “Alzheimer’s disease”, “signaling pathways”, “Aβ”, Aβo”, “Tau”, “p-Tau”, “neuronal death”, “BBB damage”, “neuroinflammation”, “microglia”, “mitochondrial dysfunction”, “granulovacuolar degeneration”, “synaptic loss”, “axonal degeneration”, “Nec-1”, “Nec-1s”, “GSK872”, “NSA”, “OGA”, “RIPK1”, “RIPK3”, and “MLKL”. Considering the novelty of the study as well as the latest research progress in the field, the retrieval time was set to be before September 28, 2022.
ACTIVATED PATHWAY OF NECROPTOSIS
In specific conditions, necroptosis is activated by DRs, including TNF receptor 1 (TNFR1), IFN receptor (IFNR), TRAIL receptors (TRAILR), and Toll like receptor (TLR)4/3, which upon stimulation by their cognate ligands, activate RIPK1. The subsequent section briefly introduces the activation of necroptosis according to two aspects of the different signaling pathways involved as well as the execution of downstream MLKL.
Different signaling pathways involved
TNF-α-triggered necroptosis is the most widely studied mechanism of necroptosis, in which TNF-α binds primarily to two TNF receptor subtypes, TNFR1 and TNFR2 [21]. Unlike TNFR1, the latter does not contain the intracellular death domain (DD) necessary to mediate cell death responses [22]. Therefore, this review mainly discusses the TNFR1-mediated death signaling pathway (Fig. 1). Upon stimulation by TNF-α, TNFR1 interacts with TNFR1DD to recruit cellular inhibitor of apoptosis proteins (cIAP), RIPK1, TNF receptor associated factor (TRAF) and TNF receptor-associated death domain (TRADD) to the intracellular portion of TNFR1, thereby forming complex I [23]. Complex I then activates NF-κB and AP1 transcription factors in order to promote cell survival and pro-inflammatory gene expression [24]. When the ubiquitination of RIPK1 in complex I is inhibited, RIPK1 dissociates from the cell membrane and recruits TRADD and TRADD-FAS-associated DD protein (FADD) to form complex IIa, which then activates caspase-8 and leads to apoptosis [25]. When caspase activity is insufficient (via genetic ablation or pharmacological inhibition), RIPK1 and RIPK3 interact through the RIP homology-interacting domain (RHIM) to form RIPK1-RIPK3 necrosomes while phosphorylating RIPK3 (complex IIb) [26]. This is followed by p-RIPK3, which phosphorylates MLKL and facilitates its translocation to the plasma membrane, where MLKL triggers cell lysis, resulting in lytic cell death as well as the release of damage-associated molecular patterns (DAMPs) [24, 27].
IFN signaling is known as a signaling pathway that can activate necroptosis [28]. The IFN-I receptor consists of a heterodimer of two IFN-α receptor type I proteins (IFNAR1 and IFNAR2). Moreover, the binding of IFN-I to ubiquitous IFN receptor 1 (IFNAR1) activates Janus kinase (JAK), which further activates signal transducers and activators of transcription (STAT) 1/2 and interferon regulatory factor 9 (IRF9) in order to form IFN stimulation of the gene factor 3 (ISGF3) complexes (Fig. 1). The ISGF3 complex then promotes the transcriptional activation of interferon-inducible protein kinase R (PKR). Once activated, PKR recruits RIPK1 and RIPK3 to form a PKR necrosome, which phosphorylates MLKL to form RIPK1-RIPK3-MLKL to promote necroptosis [29]. Notably, PKR necrosomes are negatively regulated by FADD and caspase, and when FADD is silenced or when caspase is inhibited, RIPK kinase is overactivated, and ROS are overproduced and cannot be efficiently quenched in the mitochondria, leading to cell necroptosis [30].
Initiators of the TLR signaling pathway, including LPS-activated TLR4 signaling and TLR3 (which is activated by dsRNA in the endosome), are able to induce necroptosis following caspase inhibition via downstream TIR-domain-containing adapter-inducing interferon-β (TRIF). TRIF interacts with TLR3 or TLR4 through the RHIM domain in order to recruit RIPK1/3; when caspase is inhibited, necroptosis occurs [31].
Fig. 1
Activated pathway of necroptosis. TNF signaling: Upon stimulation by TNFα, TNFR1 interacts with TNFR1 DD to recruit cellular inhibitor of apoptosis proteins (cIAP), RIPK1, TNF receptor associated factor (TRAF), and TNF receptor-associated death domain (TRADD) to the intracellular portion of TNFR1 to form complex I and activation NF-κB. When the ubiquitination of RIPK1 in complex I is inhibited, RIPK1 dissociates from the cell membrane and recruits TRADD, TRADD-FAS-associated DD protein (FADD) to form complex IIa that activates caspase-8, and leads to apoptosis. When caspase activity is insufficient, RIPK1 and RIPK3 interact through the RIP homology-interacting domain (RHIM) to form RIPK1-RIPK3 necrosomes and phosphorylate RIPK3 (complex IIb). This is followed by p-RIPK3 which can phosphorylate MLKL, facilitating its translocation to the plasma membrane where MLKL leads to cell lysis, resulting in Ca2 + influx, Na+, K+, and Mg2 + efflux, and DAMP release. IFN signaling: Binding of IFN-I to IFNAR1 further leads to PKR necrosome formation by activating the assembly of the ISGF3 complex, which phosphorylates MLKL to form RIPK1-RIPK3-MLKL for necroptosis. TLR signaling: TLR3/4 are activated by their respective ligands, and TLRs can activate necroptosis by recruiting RIPK1-RIPK3-MLKL necrosomes by linking TRIF.
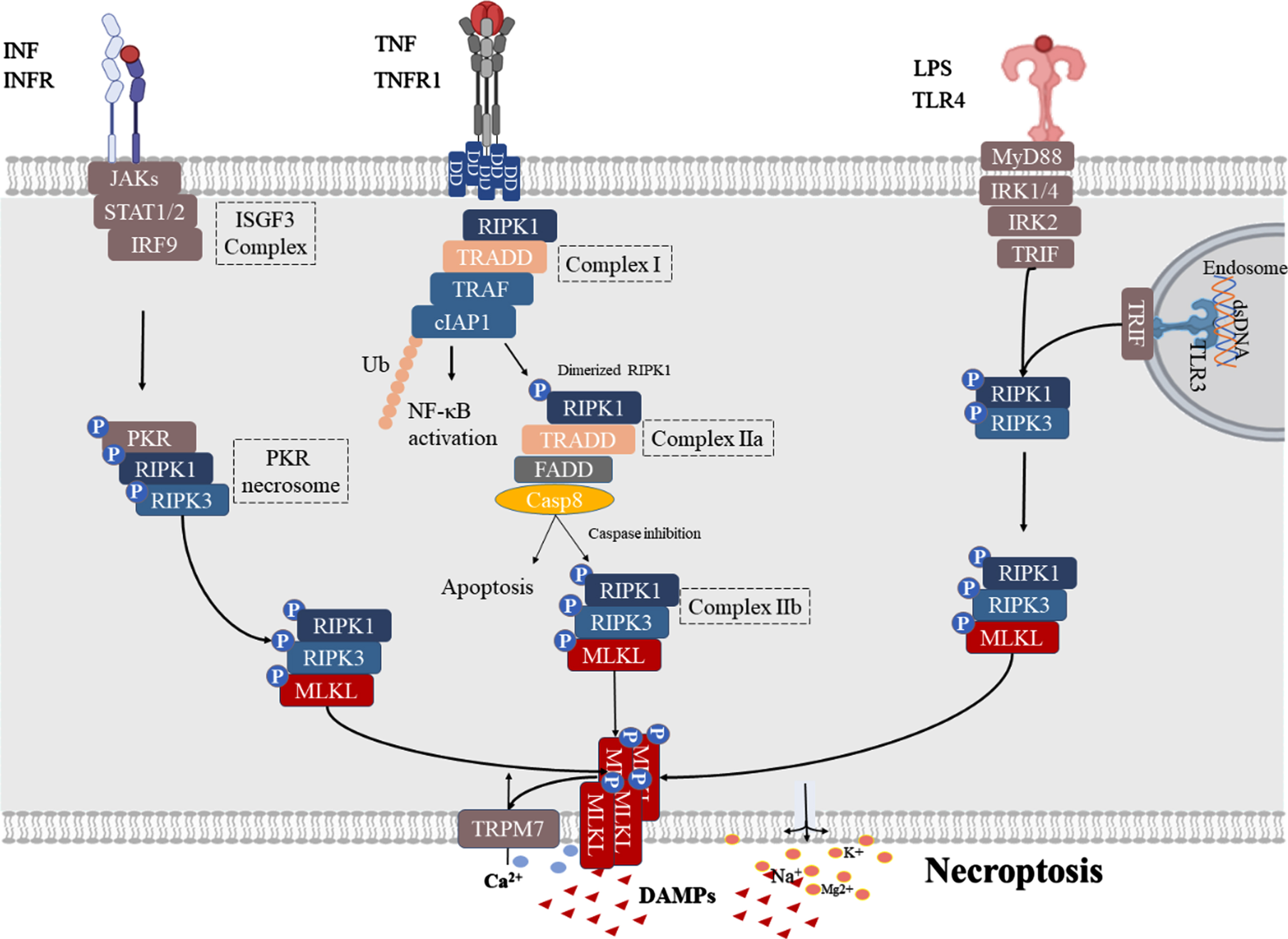
The executor of necroptosis: MLKL
To date, MLKL has been identified as a downstream target of RIPK3, which further induces cell death by perforating the cell membrane leading to leakage of cellular contents and release of inflammatory factors [32]. Specifically, MLKL contains an N-terminal 4-helix bundle (4HB) domain, an intermediate brace region composed of two helices (Brace), and a C-terminal pseudo kinase domain (PsKD). Activation of MLKL requires RIPK3 to bind PsKD and phosphorylate MLKL, thereby inducing the release of the 4HB domain and formation of homo-oligomers through the Brace [33–35]. Upon activation, MLKL binds to the cytoplasmic membrane via an affinity site that binds phosphatidylinositol phosphate (PIP), and when MLKL undergoes translocation to the cytoplasmic membrane, it disrupts membrane integrity in a dose-dependent manner [36]. During this process, when MLKL binds to the cell membrane, its 4HB domain uses a “flip” mechanism to expose additional high-affinity PIP-binding sites, which adds another layer of different PIP-binding sites, making MLKL more plasma-membrane-bound [37]. Six helices (H1-H6) in the N-terminal domain following the translocation of MLKL form a cation channel that is permeable to Mg2 +, Na+, and K+ [38]. Moreover, oligomerized MLKL binds to transient receptor potential melastatin related 7 (TRPM7), allowing to Ca2 + influx, thereby leading to cell swelling and plasma membrane rupture in order to release DAMP [39].
NECROPTOSIS CONTRIBUTES TO AD PROGRESSION IN MULTIPLE PATHOLOGICAL PROCESSES
Necroptosis had been extensively explored in neurodegenerative diseases research, including multiple sclerosis (MS) [40, 41], AD [9, 11], Parkinson’s disease (PD), and amyotrophic lateral sclerosis (ALS) [42, 43]. The activation of necroptosis has been detected in AD brains of both humans and mice, which plays a key role in AD progression in association with multiple pathological processes (Fig. 2).
Fig. 2
Multiple influences of necroptosis activation on AD related factors, which may serve as important triggers of cognitive impairment.
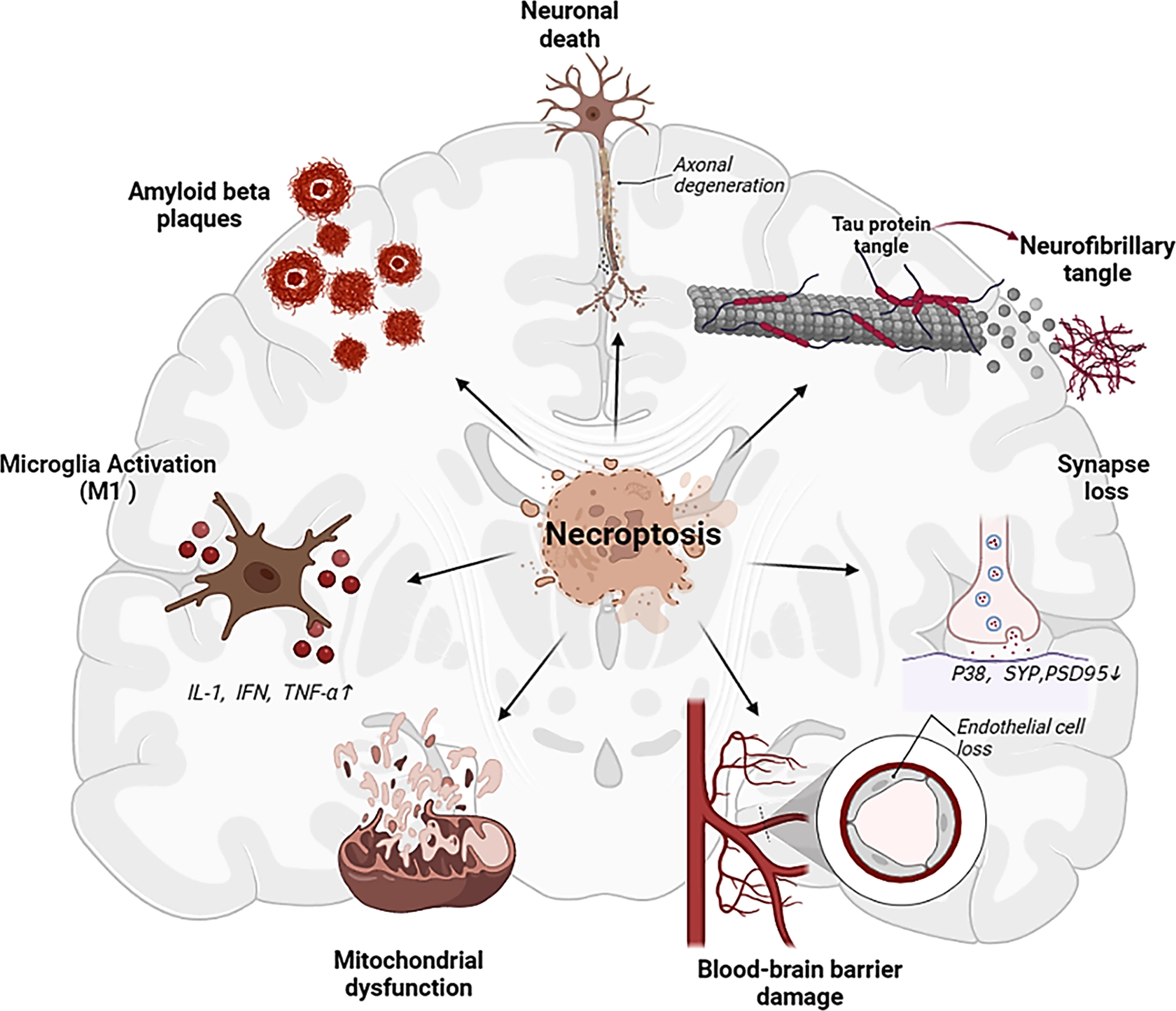
Necroptosis and Aβ aggregation
Aβ is deposited in the brain decades before the manifestation of clinical symptoms and induces senile plaque formation at a later stage [44, 45], thereby facilitating neuronal cell death and dementia [46]. Aβ is the product of hydrolysis of amyloid-β protein precursor (AβPP), which is first sorted in the endoplasmic reticulum (ER) and then transported from the Golgi apparatus to the trans-Golgi-network (TGN) [47–49]. Next, a portion of AβPP is transported as secretory vesicles to the cell surface or endosomal compartment where it is hydrolyzed by α-secretase in order to generate neuroprotective sAβPPα through a non-amyloid pathway [50–52]. The other part is cleaved by β-site amyloid cleavage enzyme 1 (BACE1) into β-N-terminal fragment (sAβPPβ) and membrane-bound C99. The latter acts on the 40/42 amino acid position of the Aβ sequence by γ secretase to produce a 39–42 amino acid peptide-Aβ and another intracellular fragment AβPP intracellular domain (AICD) [53, 54]. Aβ peptides exist in the form of monomers, oligomers (Aβo), and fibers/aggregates. Aβ monomers are relatively non-pathogenic, though they aggregate into toxic insoluble fibers that eventually assemble into amyloid plaques, of which Aβo is the most toxic type of Aβ [55–58]. Aβ insoluble fibers and oligomers often synergistically produce multiple cytotoxic effects, leading to neuronal death, immune cell activation, and inflammatory cascade [53, 59, 60].
Through the activation of necroptosis, the RIPK1/RIPK3 complex forms amyloid fibril structures [26]. These cross-β-structured amyloids result in a rise level of full-length AβPP and sAβPPβ at the cell surface [61, 62] (Fig. 3). Consistent with this, in Aβ-treated SH-SY5Y cells, RIPK1 on Aβ-induced cell death and endogenous AβPP protein stability was demonstrated [63]. Nec-1, the first RIPK1 kinase inhibitor discovered, has been shown to inhibit the downstream activation of necroptosis by binding to the hydrophobic pocket between the N- and C-termini of the RIPK1 kinase domain [64, 65]. Studies have also shown that Nec-1 small molecules can dock in several hydrophobic pockets in the Aβ fibril structure and may bind to hydrophobic sites to dissociate Aβ aggregates [11]. This finding indicated that necroptosis promotes the aggregation of Aβ through the formation of insoluble amyloid complexes [61]. Inhibiting RIPK1 kinase activity via pharmacology and genetic techniques has been found to reduce this phenomenon in APP/PS1 mice [11, 19].
Fig. 3
The interaction of necroptosis and Aβ formation and Tau. Binding of the death receptor TNF and the ligand TNFR1 stimulates the formation of necrosomes, which consist of RIPK1 and RIPK3 and allow autophosphorylation. Then, p-RIPK3 recruits and phosphorylates MLKL, promoting its translocation to the plasma membrane, leading to necroptosis. In this pathway, the RIPK1/RIPK3 complex forms amyloid fibril structures and increases cell surface levels of full-length AβPP and sAβPPβ, promoting Aβ plaque aggregation. Furthermore, Aβ plaques act as a reservoir for Aβo, and when Aβo is released, it stimulates microglia to secrete inflammatory factors, including TNF-α, and to undergo necroptosis. On the other hand, hyperphosphorylated Tau promotes necroptosis by activating the RIPK1/RIPK3/MLKL axis and simultaneously activates the NF-κB pathway, leading to M1microglial activation and neuroinflammation.
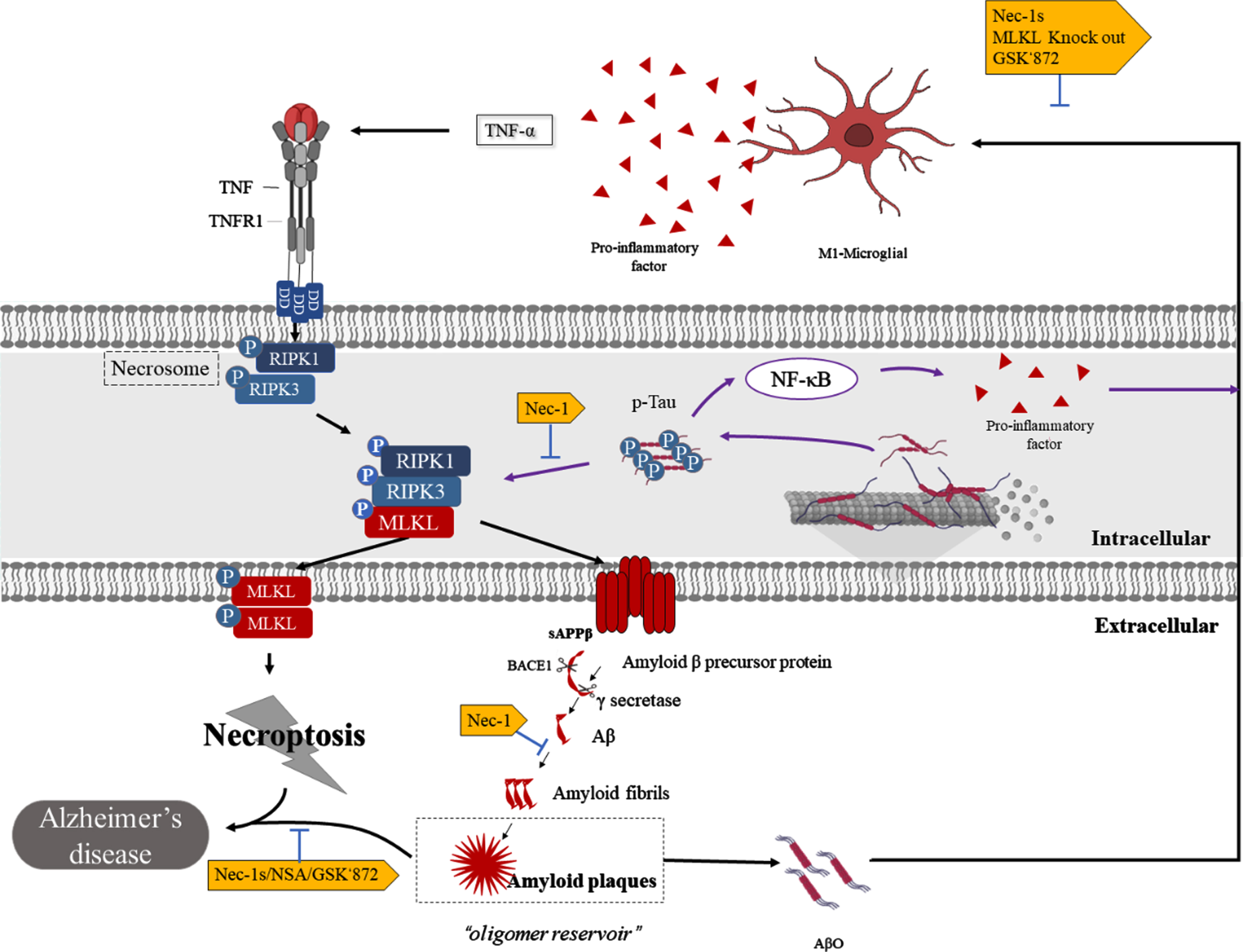
Aβ aggregates and Aβo, in turn, promote neuronal necroptosis. Studies have shown that Aβ aggregates induce neuronal necrosis through the RIPK1-MLKL axis and are inhibited by Nec-1 [11, 63]. It has been hypothesized that Aβ plaques act as a reservoir for toxic oligomers, where over time the plaques saturate and Aβo diffuses, exerting toxicity on peripheral neurons [56, 66–68]. This has been found to be consistent with the findings in which Aβ plaques frequently localize around necroptotic complexes [8]. In fact, Aβo has been shown to activate necroptosis and neurodegeneration in microglia through the promotion of TNF-α release from microglia, with Infliximab protecting it. Interestingly, no neurodegeneration has been found in neurons treated with TNF-α alone [10]. Meanwhile, the knockout of MLKL, or pharmacological inhibition of RIPK3 (GSK’872), has been shown to reverse this phenomenon and improve the cognitive impairment of Aβo-induced mice [10]. Therefore, necroptosis and Aβ may form a cascading effect in AD; that is, necroptosis complexes promote Aβ aggregation to form plaques, and over time, AβO diffusion further exacerbates neurotoxicity and disease severity.
Necroptosis and p-Tau
Tau protein is known to serve as a major microtubule-associated protein (MAPT) in the mammalian nervous system. In AD, abnormal post-translational modifications have been shown to give rise to hyperphosphorylation of Tau (p-Tau) [69, 70]. p-Tau accumulates in neurons in order to form NFTs at several residues, including Ser393/404, Ser202, and Thr 205 [71]. This process destroys the microtubule structure of neurons, resulting in a loss of communication between the neurons. Braak et al. demonstrated in their clinicopathological correlation study that AD brain pathological changes can be divided into six stages, which are referred to as “Braak stages”, according to the amount of NFT accumulation and regions involved [72]. In addition, p-Tau has been detected in the cerebrospinal fluid and blood of AD patients, with the amount detected correlating with the degree of cognitive impairment [73].
In a study on GVDs, the co-expression of pMLKL and p-Tau in GVD-bearing neurons has been observed in AD and pre-AD human brains, suggesting that Tau accumulation may serve as a key necroptosis-activated trigger [14], which have been confirmed by recent studies [12]. Specifically, in three cell lines (HT22 nerve cells, HEK293T cells, and SH-SY5Y cell line) transfected with p-Tau, Tau was found to mediate necroptosis and inflammation via activation of the RIPK1/RIPK3/MLKL and NF-κB pathways in promoting cell death [12] (Fig. 3). The in vitro findings were then further validated in separate in vivo studies in TauP301S mice. Remarkably, Nec-1stable (Nec-1s, a Nec-1 analog) was found to inhibit p-Tau-induced neuronal death and microglial hyperactivation in TauP301S mice, significantly downregulate cytokine expression, and ameliorate cognitive deficits in an AD model [12]. Similarly, in APP/PS1 mice, Nec-1 has been shown to reduce p-Tau by blocking p-Tau S199 and inhibiting aggregation through direct interaction [9, 11]. Furthermore, in an AlCl3-induced AD rat model, treatment with necrosulfonamide (NSA), a pharmacological inhibitor of MLKL, has been found to reduce p-Tau levels and improve associated spatial learning and memory deficits [20]. These findings suggest a close relationship between p-Tau and necroptosis. Furthermore, pharmacological intervention of RIPK1 and MLKL can reduce p-Tau-induced cell death and amend cognitive deficits in AD models.
Necroptosis and neuronal death
Neuronal death in the hippocampus serves as the main pathological hallmark of AD and is closely related to memory loss. Neuronal loss in certain regions, such as layer II of the entorhinal cortex, Meynert basal ganglia, and locus coeruleus has been shown to occur even before the onset of AD symptoms [74].
Conventional wisdom posits that neuronal death in AD is usually based on two categories: caspase-mediated apoptosis and DRs-mediated necroptosis [75, 76]. Apoptosis is characterized by cell shrinkage, membrane blebbing, as well as the formation of apoptotic bodies. In various studies, the caspase-mediated apoptosis signaling pathway has been found to be inactive in AD [8, 77, 78]. Instead, these cells exhibited a necroptotic swollen morphology and were found to be positive for DNA fragmentation, demonstrating that apoptosis does not sufficiently explain neuronal loss in AD. In addition, according to disease development, apoptosis is acute, and cells are lost within hours or days after apoptosis, while AD is a progressive process lasting decade [79, 80]. In terms of cellular immune response, apoptosis is immune silent, and its activation does not cause an inflammatory response [81–83]. Necroptosis typically results in the rupture of the plasma membrane as well as the subsequent release of cellular content into the surrounding environment, leading to a severe inflammatory response that may contribute to neuroinflammation in AD [84]. In view of these findings, apoptosis may not serve as the only factor that can lead to neuronal death as necroptosis is also involved.
Previous studies have demonstrated that necroptosis is activated in AD and that two key necroptosis proteins, RIPK1 and MLKL, are significantly increased in the AD postmortem brain, which leads to decreased neuronal survival and brain weight [8, 9]. Furthermore, TNFR1/RIPK1 signaling and altered expression of endosomal sorting complexes required for transport group III (ESCRT III) have been found to be activated in the AD postmortem brain, which may serve as a possible mechanism underlying neuronal necroptosis [8]. The latter has been identified to be an antagonist of necroptosis as well as a possible mechanism by which cells can survive after necroptosis has been triggered [85]. However, the small molecule inhibitors of RIPK1, RIPK3, and MLKL have been observed to significantly reverse this effect [5, 8, 9, 86]. These studies demonstrated that neuronal death may play a role in degeneration as an independent factor rather than a downstream product of pathogenic proteins.
In fact, we do not deny the existence of other types of programmed cell death in AD including pyroptosis, ferroptosis, oxytosis, autophagy, etc. Moreover, there may be extensive crosstalk between these mechanisms in chronic degenerative diseases, where they may interact with each other intracellularly, with the dominant mechanism ultimately leading to cell death and further giving rise to inflammation and contributing to further neurotoxicity.
Necroptosis and BBB damage
The BBB is mainly composed of continuous capillary endothelial cells (capECs), continuous basement membrane, pericytes, and astrocytes, of which endothelial cells (ECs) are the main components of the BBB. This structure has selective permeability and transport functions for peripheral substances, protecting neurons from various factors in the systemic circulation, thereby maintaining a highly regulated internal homeostasis of the central nervous system. A dynamic contrast-enhanced (DCE) MRI study of individuals with mild cognitive impairment described BBB injury in the hippocampus, suggesting that BBB damage occurs early in AD [87]. Studies have shown that BBB damage and Aβ act independently and/or synergistically in order to promote AD progression [88–91]. BBB injury is also affected by certain factors, such as genetics (i.e., carrier of apolipoprotein E (APOE ɛ4) E4 allele), cardiovascular and cerebrovascular disease (i.e., hypertension, diabetes, and dyslipidemia) [89].
ECs play a key role in the BBB, and damage to ECs results in BBB injury with concomitant infiltration. A recent study has demonstrated that electron microscopy and immunohistochemistry can reveal the selective loss of venous and capECs in APP/PS1 mice [13]. Necroptosis in venule ECs and capECs from AD mice and postmortem human AD brain samples was found to be a major contributor to EC loss and BBB leakage. Research has shown that the inhibition of RIPK1 kinase activity ameliorates TNFα-induced increases in vascular permeability in TNFα/Z-ValAla-Asp (OMe)-fluoromethyl ketone (ZVAD, a caspase inhibitor) treated mice [92]. In other acute brain injuries, Nec1 has been found to prevent brain swelling and disruption of tight junctions in subarachnoid hemorrhage as well as avert BBB injury triggered by necroptosis in mice models of cerebral ischemia/reperfusion injury [93, 94]. Accordingly, the corresponding studies confirmed that necroptosis plays an important role in BBB injury. Furthermore, previous studies have shown that necroptosis promotes the aggregation of Aβ via the formation of amyloid complexes [11], whereas Aβ promotes AD progression synergistically with BBB injury [95]. Therefore, it is necessary to elucidate how necroptosis-induced Aβ deposition leads to BBB damage in other animal models.
Necroptosis and neuroinflammation
Neuroinflammation is a common AD feature. Microglia, which constitute the main immune cells of the brain, work with oligodendrocytes and astrocytes in order to maintain central nervous system homeostasis and have been shown to coordinate neuroinflammation and play a key role [96, 97]. Through the influence of different environments, M1-type microglia usually exhibit a pro-inflammatory phenotype, whereas M2-type microglia have an anti-inflammatory phenotype. In AD, M1-type microglia release inflammatory factors, such as IL-1, IFN, and TNF-α, which have been shown to be involved in neuroinflammation and further lead to peripheral neuronal death [98, 99]. M2-type microglia is induced by IL-4 and/or IL-10 and are associated with clearance of Aβ [100, 101]. Microglial responses have been initially thought to be serendipitously triggered by Aβ amyloid plaques and dystrophic neurites. Genome-wide association studies have also shown that many AD risk genomes are highly expressed at or near genes unique to microglia [97]. Recently, due to progress in necroptosis research, numerous studies have illustrated that necroptosis activates microglial activation.
Specifically, necroptosis has been described to be active in the microglia and can induce the M1 polarization of microglia/macrophages [102–104]. In rd1 mice with retinal degeneration, as well as in mice with acute retinal nerve injury, microglia have been shown to undergo RIPK1- and RIPK3-dependent necroptosis and release multiple proinflammatory cytokines and chemokines via TLR4 signaling pathway [105]. Likewise, in the macrophages/microglia of mice, MLKL and the key necroptosis regulator Z-DNA/RNA-binding protein 1 were found to be mainly induced in M1 but not M2 macrophages/microglia [106]. Ablating RIPK3 or MLKL could switch the activation of microglia/macrophages from M1 to the M2 type in the ischemic cortex [107]. Studies have shown that RIPK1 is highly expressed in the microglia of AD patients [19]. By inhibiting RIPK1 kinase expression, a significant reduction in M1 microglia and levels of proinflammatory cytokines have been demonstrated. Specifically, the kinase activity of RIPK1 has been described to mediate the transcriptional upregulation of Cystatin 7 and Cholesterol-25-Hydroxylase, leading to the inhibition of cathepsin activity and impairment in the microglial lysosomal pathway in AD mice microglia [19]. These studies further demonstrate that the activation of necroptosis mediated the inflammatory response and phagocytic capacity damage of microglia. Therefore, targeting necroptosis can promote the transformation of M1 to M2 phenotype and restore the phagocytic function of microglia, which may potentially serve as a therapeutic strategy for AD.
Necroptosis and mitochondrial dysfunction
The brain is characterized by high energy metabolic demands and is extremely dependent on mitochondria for its energy supply; therefore, it is extremely sensitive to mitochondrial dysfunction. Extensive research has demonstrated that mitochondrial dysfunction in AD is closely related, which can manifest in mitochondrial fission-fusion, abnormal mitochondrial dynamics, and oxidative damage [108–110]. Studies have shown that mitochondria in AD have decreased activity in all respiratory chain complexes, having the most significant decrease in complex IV, which results in increased ROS production, decreased ATP production, and DNA damage. These factors contribute to the processing and aggregation of Aβ, thus triggering synaptic degeneration and cognitive decline [111–113]. p-Tau also leads to mitochondrial dysfunction, affecting the axonal transport of organelles in AD neurons and ultimately leading to neuronal dysfunction [114].
Past studies have found that necroptosis is involved in mitochondrial dysfunction. Specifically, the mitochondrial protein phosphatase PGAM5 is a component of the RIPK1/RIPK3 complex and manifests as two splice variants, PGAM5L (long form) and PGAM5S (short form). Following the induction of necroptosis, PGAM5S further induces mitochondrial fragmentation through the recruitment of dynamin-related protein 1 (Drp1) and activation of its GTPase activity by dephosphorylation, which are key steps in the subsequent execution of necroptosis [18]. On the contrary, RIPK1/MLKL/PGAM5L interaction is blocked by another necroptosis inhibitor, NSA. A recent study has reported that active necrosomes are translocated to the mitochondria in an MLKL-dependent manner, in which RIPK3 directly phosphorylates the E3 subunit of the pyruvate dehydrogenase complex (PDC) at T135, leading to PDC activation, and subsequent rise in aerobic respiration and mitochondrial reactive oxygen species production [115]. Interestingly, mitochondrial ROS in turn induces autophosphorylation of RIPK1 at Ser161, which is critical for the formation of RIPK1-RIPK3 necrosomes [116, 117]. These results may suggest that mitochondrial dysfunction is greatly involved or tends to form a feedback loop in order to induce necroptosis. Essentially, the inhibition of necroptosis was shown to aid in the recovery of mitochondrial dysfunction in neurons, ultimately alleviating cognitive impairment.
Necroptosis and granulovacuolar degeneration
GVD was first proposed in 1911. When GVD forms up to 3–5μm in diameter, a membrane-bound structure of edged vacuoles with a dense argyrophilic core is present. In AD pathology, it is often characterized by the presence of abundant GVDs in neurons with ultrastructural resemblance to lysosomes [118]. These GVDs bodies have been initially and predominantly found in hippocampal pyramidal neurons in CA1 and CA2. As AD progresses, the systemic spread of GVD lesions in the brain, such as the temporal lobe, hypothalamus, and amygdala, has been observed [119, 120]. Accordingly, in vitro induction of intracellular aggregation of Tau has been found to lead to the formation of GVDs in mouse primary neurons [121]. In the AD hippocampus, the number of neurons with GVDs increases according to the Braak stage of NFTs, which may participate in the formation of NFTs [122–125].
Koper’s team was the first to describe the relationship between necroptosis and GVDs in the context of AD-associated neuronal loss, proposing the hypothesis of GVDs as a morphological correlate of necrosome activation in AD [14]. In addition, their study examined areas with significant neuronal loss in pre-AD and AD patient, in which the presence of large numbers of necrosome-positive GVDs were found. In other words, GVD-mediated necroptosis leads to massive neuronal loss in pre-AD and AD cases. Recently, Koper et al. have found that hippocampal transactive response DNA binding protein 43 (TDP-43) inclusion bodies are closely related to GVD-mediated necroptosis [126]. TDP-43 inclusion bodies have been frequently detected in up to 57% of AD cases and have been associated with worsening brain atrophy and greater memory loss in AD patients [127, 128]. Notably, a large number of necrosome-positive GVDs were found in the hippocampus of AD patients with TDP-43 compared with AD patients without TDP-43 [126, 128]. Consistent with this, in a study of C9ORF72-mutated ALS/frontotemporal dementia (FTLD) cases, TDP-43-related necrosome-positive GVD was also observed in the post-mortem hippocampus [129]. Taken together, TDP-43 aggravates GVD-mediated necroptosis in AD and ALS/FTLD cases and leads to more severe dementia. However, further elucidation of the mechanism by which TDP-43 activates GVD-mediated necroptosis in AD is needed.
Necroptosis and other AD pathological events
Synaptic loss
Studies have suggested that between 27% and 42% of synapses in the frontal cortex of the brain are lost in AD patients [130–135], in which the absence of key elements in interneuron communication has been thought to constitute the main morphological counterpart of cognitive deficits in AD. Cornel Iridoid Glycoside (CIG), the main component of cornus officinalis, which is widely used in China to treat age-related diseases and dementia, has been shown to increase the expression of synaptophysin, postsynaptic density-95 (PSD95), and Glutamate receptor 1 (GluR1) in the hippocampus of SAMP8 mice by inhibiting the RIPK1/MLKL pathway [15]. However, whether necroptosis inhibitors rescue AD synaptic damage has yet to be reported as there is a lack of strong morphological evidence.
Axonal degeneration
Axonal degeneration is another important pathological event of advanced AD [136]. Recent studies have found that necroptosis is a key mechanism in axonal degeneration following excitotoxicity [137]. Activation of necroptosis, mitochondrial dysfunction, and axonal degeneration has been frequently observed in neurons [136]. Moreover, the pharmacological inhibition of RIPK1 has been found to prevent key steps in the axonal degeneration cascade, including mitochondrial depolarization, opening of the permeability transition pore, and Ca2 + dysregulation in the axon. In accordance with this finding, the same effect was observed for RIPK3 and MLKL knockdown [118].
THERAPEUTIC MEASURES TARGETING THE AD BRAIN NECROPTOSIS PATHWAY
Pharmacological intervention of necroptosis in AD animal models (Table 1)
Data from in vivo experiments have indicated that several compounds inhibit necroptosis and improve cognitive impairment in AD models. These compounds include necroptosis (RIPK1/RIPK3/MLKL) inhibitors and other drugs.
Table 1
Pharmacological intervention of necroptosis in AD animal models
Animal model | Drug | Administration route | Duration and dosage | Neuron survival | Abnormal protein | Cognitive function | Other Molecular mechanisms | Necroptosis protein | REF |
APP/PS1 mice | Nec-1 | IV | 12 weeks (6.25 mg/kg, 2 times /week) | ↑ | ↓soluble and insoluble Aβ1 - 42 p-Tau and p-S199 | Improve | ↑ Bcl-2 ↓Cytochrome-c and cleaved-Caspase3 | ↓pRIPK3 | [11] |
Aluminum induced AD mice | Nec-1 | ICV | 2μl of Nec-1 at the concentrations of 2 mM, 4 mM and 8 mM were bilaterally injected into each cerebral ventricle | ↑ | ↓Aβ and Tau | Improve | ↑mGluR2 and mGluR5 ↓Caspase-3 and LC3-□ | ↓RIPK1 | [139] |
APP/PS1 mice | Nec-1 | IV | 4 weeks (6.25 mg/kg, 2 times/week) | ↑ | ↓Aβ plaques and p-Tau | N/A | ↑Bcl-2 ↓Bax | ↓pRIPK3 | [159] |
Prediabetic rat | Nec-1 | Gavage | 8 weeks (1.65 mg/kg/day) | N/A | ↓p-Tau and Tau | Improve | ↓p-NF-kB/NF-kB | ↓pRIPK1, RIPK1, and pRIPK3 | [141] |
Aluminum induced zebrafish AD model | Nec-1 | Add Nec-1 to zebrafish culture fluid | 30 days (15μmoL/L) | ↑ | N/A | Improve | ↑ACh Levels | ↓RIPK1, RIPK3, PARP2, Bmf1, and Rab25 | [140] |
APP/PS1 mice | Nec-1s | PO | 30 days (2.5–5 mg/d) | N/A | ↓Aβ, soluble /insoluble Aβ1 - 42 | Improve | N/A | N/A | [19] |
TauP301S mice | Nec-1s | Intraperitoneal injection | 12 weeks (2 times/week, 6.25 mg/kg) | ↑ | ↓p-Tau | Improve | ↓IBa1, CD68, IL-6, IFNβ, CCL5 ↑ TMEM119 | ↓RIPK1, RIPK3, and MLKL | [12] |
5xFAD mice | Nec-1s | Intraperitoneal injection plus po | A single intraperitoneal injection (10 mg/kg) followed by oral (0.5 mg/ mL) administration for 21 days | ↑ | N/A | N/A | N/A | ↓MLKL and pMLKL | [9] |
APP/PS1 mice | Nec-1s | PO | 4 weeks (2.5–5 mg/day) | N/A | N/A | N/A | ↑vECs and capECs ↓VCAM1 and ICAM1 | N/A | [13] |
AβO induced AD mice | GSK’872 | Intraperitoneal injection | GSK ’872 was intraperitoneally injected (1 mg/kg) on days 0 and 7 and 14 after intraventricular injection of AβO. | ↑ | N/A | Improve | N/A | ↓pMLKL | [10] |
5xFAD mice | OGA inhibitor, thiamet-G | Intraperitoneal injection | 3 days (50 mg/kg) | ↑ | ↓Aβ plaques, soluble/insoluble Aβ40 and Aβ42 | Improve | ↑Synaptophysin | ↓pRIPK1 pRIPK3, and pMLKL | [17] |
AlCl3 induced AD rat | NSA | Intraperitoneal injection | 6 weeks (1.65 mg/kg/day) | ↑ | ↓Aβ plaques and p-Tau | N/A | ↑Acetylcholine ↓BACE1, GSK-3β, TNF-α, and acetylcholinesterase | ↓pMLKL | [20] |
Aged SAMP8 mice | CIG | Gavage | 2 months (200 mg/kg/d) | ↑ | N/A | Improve | ↑PSD95, Synaptophysin, GluR1, ADAM10, and sAβPPα | ↓RIPK1, pMLKL, and MLKL | [15] |
Aβ1 - 42 induced AD rat | EGb761 | Gavage | 23 days (20 mg/kg daily) | ↑ | ↓Aβ1 - 42 | Improve | ↓p-JNK/JNK, p-STAT3 (Ser 727) and t-STAT3 | ↓RIPK1, MLKL, and pMLKL | [150] |
Aβ25 - 35-induced mice | CE | Intragastric injections | 77 days (10 or 20 mg/kg/d) | ↑ | ↓Aβ1 - 42 | Improve | ↑BDNF, FGF2 TrkB/Akt signaling axis (TrkB, Akt, Bcl-2), IL-10 ↓TNF-α, IL-1β, IL-6 | ↓RIPK1 RIPK1, RIPK3, pMLKL ↑TBK1 | [151] |
IV, intravenous injection; PO, oral; ICV, lateral ventricle injection; Bcl-2, B-cell lymphoma-2; p-RIPK3, phosphorylated receptor-interacting serine-threonine kinase 3; mGluR2/5, metabotropic glutamate receptors2/5; LC3II, microtubule-associated proteins 3II; NF-κB, nuclear factor-κB; pRIPK1, phosphorylated receptor-interacting serine-threonine kinase 1; ACh, acetylcholine; Bax, pro-apoptotic gene; PARP2, poly adenosine diphosphate ribose polymerase; Bmf1, BCL2 modifying factor 1; Rab25, member RAS oncogene family 25; IL-6, interleukin 6; IFNβ, interferon beta; CCL5, C-C motif chemokine ligand 5; TMEM119, transmembrane protein 119; MLKL, mixed lineage kinase domain like pseudokinase; vECs, venous ECs; capECs, capillary ECs; VCAM1, vascular cell adhesion molecule 1; ICAM1, intercellular cell adhesion molecule-1; BACE1, β-site amyloid cleavage enzyme 1; GSK-3β, glycogen synthase kinase 3 beta; PSD95, postsynaptic density-95; GluR1, Glutamate receptor 1; ADAM10, ADAM metallopeptidase domain 10; JNK, c-Jun N-terminal kinase; p-JNK, phosphorylated amino-terminal protein kinase; STAT3, signal transducer and activator of transcription 3; p-STAT3 (Ser 727), phosphorylated signal transducer and activator of transcription; t-STAT3, total STAT3; FGF2, Fibroblast growth factor 2; BDNF, brain-derived neurotrophic factor; TrKB, Tyrosine Kinase receptor B; AKT, Protein Kinase B, PKB; TBK1, TANK-binding kinase 1; CIG, Cornel Iridoid Glycoside; EGb761, Gingko biloba extract 761; CE, Coeloglossum viride var. bracteatum extract; Mouse Transmembrane protein 119.
RIPK1 inhibitors: Nec-1 is a classic inhibitor in targeting RIPK1 kinase [138]. To date, Nec-1 has been shown to have an ameliorating effect on multiple AD/cognitive deficit-related animal models, including APP/PS1 mice [11], aluminum induced-AD mice [139], aluminum-zebrafish AD models [140], and prediabetic rats [141]. However, the specificity of Nec-1 in inhibiting RIPK1 kinase has been widely debated [142]. This may be due to its inhibitory effect on indoleamine-2,3-dioxygenase (IDO) [142]. IDO has been found to be upregulated in inflammation and plays a major immunomodulatory role [143]; hence, the dual response to RIPK1 and IDO may have important in vivo effects. Nec-1s is a more specific RIPK1 inhibitor that lacks the IDO-targeting effect [142]. The corresponding findings suggested that Nec-1s improves cognitive deficits in SAMP8 mice [144], TauP301s mice [12], and APP/PS1 mice [19]. RIPK3 inhibitors: GSK’872 is a RIPK3 inhibitor that binds to the RIPK3 kinase domain with high affinity and inhibits kinase activity, which has also been shown to ameliorate cognitive decline in an AβO-induced AD model [10]. Although GSK872 has limited research data in terms of AD animal models, its potential in improving cognitive deficits from in vitro experiments can be appreciated [8, 106]. For example, GSK’872 was added to human iPSC-derived glutamatergic neurons and M1 macrophages induced by TNF-α, SMAC mimic, and ZVAD-fmk (TSZ, a commonly used in vitro method to induce necroptosis), which significantly reduced cytotoxicity and increased neuronal survival [8, 106]. MLKL inhibitors: NSA is a specific inhibitor of necroptosis that targets MLKL. The study of Motawi and colleagues showed that NSA reduced the pathological changes associated with cognitive decline in an AlCl3-induced AD rat model given oral administration of AlCl3 (17 mg/kg/day) for 6 consecutive weeks [20]. In future studies, the therapeutic effect of NSA must be validated in further AD models. Recently, GSK’074 and TAK-632 have been identified as dual inhibitors of RIPK1 and RIPK3, both of which exhibited extremely low cytotoxicity [145–147]. Although dual inhibitors have advantages in blocking RIPK1-dependent and -independent forms of necroptosis, how effective these dual inhibitors are in AD must be clarified.
Other related studies have demonstrated that O-GlcNAcase (OGA) inhibitors can increase O-GlcNAcylation [17, 148]. Interestingly, O-GlcNAcylation of RIPK3 has been shown to inhibit the phosphorylation of RIPK3 as well as its interaction with RIPK1, thereby further inhibiting the activation of necroptosis [16, 17, 149]. Notably, OGA inhibitors have also been found to ameliorate cognitive impairment in Tau/APP [16] and 5xFAD mice model [17] through this effect.
Regarding other drug treatments, CIG has been reported to increase the expression of synapse-associated proteins by inhibiting necroptosis and ultimately improve cognition in aged SAMP8 mice [15]. Gingko biloba extract 761 has also been shown to improve cognitive impairment in a Aβ1 - 42-induced AD rat model by attenuating RIPK1-mediated mitochondrial dysfunction and ROS [150]. Coeloglossum viride var. bracteatum extract (CE) is a plant that grows on snowy plateaus at an average altitude of 4000 meters and possesses antioxidant and anti-inflammatory properties. CE ameliorates cognitive impairment in Aβ25 - 35 induced AD mice by modulating RIPK1-driven inflammation and necroptosis [151].
Potential gene targets of necroptosis in AD animal model (Table 2)
Recently, the potential gene targets of necroptosis in AD have been gradually revealed, and data from in vivo studies have demonstrated its ability in improving cognitive impairment in the AD animal models [10, 13, 19, 152]. For example, knockout of RIPK1D138N kinase in APP/PS1 mice has been found to ameliorate cognitive impairment by reducing microglia-associated inflammation [19]. Furthermore, knockout of MLKL improved cognition as well as learning and memory in Aβo-induced AD mice models [10]. Murine N-acetyltransferase 1 (mNat1) has been shown to play an important role in preventing insulin resistance-mediated endothelial necroptosis, with its deficiency leading ECs vulnerable to necroptosis [13]. The selective restoration of mNat1 expression in ECs can inhibit EC necroptosis and ameliorate BBB damage and cognitive impairment in APP/PS1 mice [13]. UVRAG serves as a key regulator in several steps of autophagic flux. Interestingly, UVRAG overexpression has been found to inhibit necroptosis by regulating autophagy, ultimately alleviating cognitive impairment in APP/PS1 mice [152]. Previous studies have also identified p62/SQSTM1, which is a molecule responsible for the clearance of aggregated proteins, in which RIPK1 directly interacts to activate necroptosis [163]. Xu et al. showed that p62 is highly expressed in hippocampal CA1 cells of APP/PS1 mice as well as in the cortical neurons of AD patients. In contrast, p62 knockdown in APP/PS1 mice has been found to inhibit necroptosis and alleviate cognitive impairment [152]. In addition, various genes play important roles in AD necroptosis, such as: A20 [13, 153, 154], TRAF2 [155], and low-density lipoprotein receptor-related protein 1 [13]. Given that information on these genes is admittedly limited in in vitro studies, further research is needed in order to demonstrate their potential as a target for inhibiting necroptosis in AD.
Table 2
Potential genetic targets of necroptosis in AD animal models
Animal model | Genetic intervention | Neuron survival | Abnormal protein | Cognitive function | Other molecular mechanisms | Necroptosis protein | REF |
APP/PS1 mice | RIPK1D138N kinase Knock out | N/A | ↓Aβ, soluble and insoluble level of Aβ1 - 42 | Improve | N/A | ↓p-S166 RIPK1, RIPK1 | [19] |
APP/PS1 mice | RIPK1D138N kinase Knock out | N/A | N/A | N/A | ↓EC VCAM1 and ICAM1 | N/A | [13] |
MLKL Knock out mice | MLKL Knock out | ↑ | N/A | Improve | N/A | ↓pMLKL | [10] |
APP/PS1 mice | shRNA-TNFR1 | N/A | N/A | N/A | N/A | ↓pMLKL | [152] |
APP/PS1mice | mNat1 overexpression | ↑ | ↓Aβ plaques and levels of Aβ1–42 | Improve | ↑LRP1β and A20 | ↓pRIPK3, pMLKL | [13] |
APP/PS1 mice | UVRAG overexpression | ↑ | N/A | Improve | ↓P62 | ↓pMLKL | [152] |
APP/PS1 mice | shRNA-P62 AVV | ↑ | N/A | N/A | N/A | ↓RIPK1, pRIPK1, pRIPK3, MLKL, pMLKL | [152] |
EC, Endothelial cells; VCAM1, vascular cell adhesion molecule 1; ICAM1, intercellular cell adhesion molecule-1; LRP1, low-density lipoprotein receptor-related protein 1; mNat1, murine N-acetyltransferase 1; TSZ, TNF-α, SMAC mimetic, ZVAD-fmk; UVRAG, ultraviolet irradiation resistance-associated gene; LC3, microtubule-associated proteins, the ratio of LC3II/I can estimate the level of autophagy; P62, Sequestosome 1; shRNA, short hairpin RNA.
CLINICAL TRIALS TARGETING AD NECROPTOSIS
Drug developers are now working to move drug candidates into preclinical studies or clinical trials by targeting necroptosis. Currently, RIPK1 inhibitors, RIPK3 inhibitors, MLKL inhibitors, and OGA inhibitors related to necroptosis have been gradually suggested for the treatment of neurodegenerative diseases such as AD. Therefore, clinical trials regarding these inhibitors were searched for using the Informa Pharma Intelligence database (https://citeline.informa.com/), where five RIPK1 inhibitors and four OGA inhibitors were found for AD (Table 3). However, it is disappointing that no RIPK3 and MLKL inhibitors have entered clinical trials so far.
Table 3
Clinical trials for AD
Generic Drug Name | Sponsor | Trial Phase | Trial state | Target |
DNL104 | Denali Therapeutics | Phase I Clinical Trial | Ceased | Receptor interacting serine/threonine kinase 1 |
DNL747 | Denali Therapeutics | Phase I Clinical Trial | Ceased | Receptor interacting serine/threonine kinase 1 |
DNL788 | Denali Therapeutics | Phase II Clinical Trial | Active | Receptor interacting serine/threonine kinase 1 |
SIR-2446 | Sironax | Phase I Clinical Trial | Active | Receptor interacting serine/threonine kinase 1 |
VRN-04-1 (VRN-04) | Voronoi | Phase I Clinical Trial | Active | Receptor interacting serine/threonine kinase 1 |
ASN-561 | Asceneuron | Phase I Clinical Trial | Active | O-GlcNAcase |
BIIB-113 | Biogen | Phase I Clinical Trial | Active | O-GlcNAcase |
ASN-51 | Asceneuron | Phase I Clinical Trial | Active | O-GlcNAcase |
LY-3372689 | Eli Lilly | Phase II Clinical Trial | Active | O-GlcNAcase |
The above data comes from Informa Pharma Intelligence.
Clinical trials targeting RIPK1
DNL104 commenced trials in AD/ALS in September 2016, though further studies have been discontinued due to post-dose hepatotoxicity that was observed in six subjects (37.5%) in the multiple escalating dose group. SAR443060 (DNL747) is a selective, orally bioavailable, and central nervous system (CNS)-penetrant inhibitor of RIPK1. In two early-stage clinical trials (NCT03757325 and NCT03757351) in healthy subjects and in patients with AD or ALS, DNL747 has been shown to be distributed in cerebrospinal fluid after oral administration and decreased pRIPK1 in human peripheral blood mononuclear cells. However, the development of DNL747 has been discontinued due to long-term nonclinical toxicology findings [156]. Another BBB-penetrating RIPK1 inhibitor, SAR443820 (DNL788), demonstrated potent interactions with its target at well-tolerated doses in Phase 1 clinical trials (NCT04982991), which may have potential in certain neurological diseases, such as ALS, MS, and AD. It is now planned for Phase II clinical trials for both ALS and MS (NCT05237284). Importantly, the US FDA has granted Fast Track designation to SAR443820 for the treatment of ALS. SIR-2446 is an oral RIPK1 inhibitor being developed by Sironax for the treatment of AD and MS, which is currently in Phase I clinical trials. VRN-04-1 (VRN-04) is another oral RIPK1 inhibitor developed by Voronoi for the treatment of various autoimmune diseases and neuroinflammation diseases, such as AD, PD, ALS, and MS, which in preclinical trials.
Clinical trials targeting OGA
ASN120290 (ASN-561 or ASN90, developed by Asceneuron) is used for the treatment of progressive supranuclear palsy, AD, and tauopathies, which has already completed testing in three Phase I studies in healthy young and elderly subjects. Preclinical data has shown that daily oral administration of ASN120290 prevents the development of Tau tangles, functional deficits in motor behavior and breathing, and increased survival [157]. BIIB-113 (developed by Biogen) is currently in a Phase I clinical trial for AD to evaluate the safety and tolerability of single- and multiple-ascending oral BIIB113 in healthy participants (NCT05195008). ASN-51 (developed by Asceneuron) has also entered Phase I clinical trials for AD. The safety, tolerability, pharmacokinetics, and pharmacodynamics of ASN51 have been evaluated in healthy subjects and AD subjects (NCT04759365). LY-3372689 (developed by Eli Lilly and Company) for the treatment of AD has completed safety, tolerability, and pharmacokinetic evaluations in 23 healthy subjects (NCT03819270, NCT04106206, NCT03944031, and NCT04392271). It was well tolerated, with no serious adverse events reported and is currently undergoing recruitment for a Phase II clinical trial (NCT05063539).
CONCLUSION AND FUTURE DIRECTIONS
Due to the rapidly aging population, novel disease-modifying therapies and treatment strategies are urgently needed for AD. In fact, for decades, researchers have characterized several aspects of AD pathology as separate components that may now be associated with susceptibility to necroptosis. The existing evidence has demonstrated that necroptosis is involved in the neuropathology of AD [8–10, 14, 19, 91, 158]. For these reasons, there is indeed a broad correlation between AD neuropathology and the necroptosis pathway. Therefore, the role of necroptosis in AD is multifaceted, and inhibiting the activation of necroptosis may serve as a multi-targeted therapeutic approach for AD.
However, several outstanding questions regarding AD necroptosis remain to be further addressed. 1) The above studies show a strong correlation between necroptosis and AD pathological changes. However, as AD is a slowly progressive disease, it is necessary to clarify the degree of necroptosis in AD animal models at different pathological stages and to find the optimal treatment time. 2) Nec-1 targets the dissociation of Aβ aggregates; however, current evidence only supports a role in the pre-AD stage. Thus, more studies are warranted to investigate whether other dual inhibitors have binding sites for Aβ, and whether the treatment is better than Nec-1. 3) The hypothesis of GVDs as the morphological counterpart of AD necrosome needs to be further validated in vivo. Furthermore, the mechanism by which TDP-43 activates GVD-mediated necroptosis in AD should be elucidated. 4) Synapse loss is considered to be the main morphological counterpart of cognitive deficits and memory impairment in AD. Although the inhibition of necroptosis signaling improves the expression of synapse-related proteins, more robust morphological evidence is required. In conclusion, this review put forward evidence in regard to the involvement of necroptosis in AD progression according to AD neuropathology. However, the role of necroptosis in AD may only be confirmed when further necroptosis-based interventions successfully complete clinical trials. Authors’ disclosures available online (https://www.j-alz.com/manuscript-disclosures/22-0809r2).
ACKNOWLEDGMENTS
This work is supported by grants from the Wu Jieping medical foundation clinical research project (320.6750.2022-08-3). The authors would like to thank Zibo Yimore Translation CO. LTD for providing English proofreading services for this paper.
REFERENCES
[1] | Hodson R ((2018) ) Alzheimer’s disease. Nature 559: , S1. |
[2] | Scheltens P , Blennow K , Breteler MM , de Strooper B , Frisoni GB , Salloway S , Van der Flier WM ((2016) ) Alzheimer’s disease. Lancet 388: , 505–517. |
[3] | Nelson PT , Alafuzoff I , Bigio EH , Bouras C , Braak H , Cairns NJ , Castellani RJ , Crain BJ , Davies P , Del Tredici K , Duyckaerts C , Frosch MP , Haroutunian V , Hof PR , Hulette CM , Hyman BT , Iwatsubo T , Jellinger KA , Jicha GA , Kövari E , Kukull WA , Leverenz JB , Love S , Mackenzie IR , Mann DM , Masliah E , McKee AC , Montine TJ , Morris JC , Schneider JA , Sonnen JA , Thal DR , Trojanowski JQ , Troncoso JC , Wisniewski T , Woltjer RL , Beach TG ((2012) ) Correlation of Alzheimer disease neuropathologic changes with cognitive status: A review of the literature. J Neuropathol Exp Neurol 71: , 362–381. |
[4] | Scheff SW , Price DA , Schmitt FA , Mufson EJ ((2006) ) Hippocampal synaptic loss in early Alzheimer’s disease and mild cognitive impairment. Neurobiol Aging 27: , 1372–1384. |
[5] | Alzheimer A , Stelzmann RA , Schnitzlein HN , Murtagh FR ((1995) ) An English translation of Alzheimer’s 1907 paper, “Uber eine eigenartige Erkankung der Hirnrinde”. Clin Anat 8: , 429–431. |
[6] | Querfurth HW , LaFerla FM ((2010) ) Alzheimer’s disease. N Engl J Med 362: , 329–344. |
[7] | Vanden Berghe T , Grootjans S , Goossens V , Dondelinger Y , Krysko DV , Takahashi N , Vandenabeele P ((2013) ) Determination of apoptotic and necrotic cell death in vitro and in vivo. Methods 61: , 117–129. |
[8] | Jayaraman A , Htike TT , James R , Picon C , Reynolds R ((2021) ) TNF-mediated neuroinflammation is linked to neuronal necroptosis in Alzheimer’s disease hippocampus. Acta Neuropathol Commun 9: , 159. |
[9] | Caccamo A , Branca C , Piras IS , Ferreira E , Huentelman MJ , Liang WS , Readhead B , Dudley JT , Spangenberg EE , Green KN , Belfiore R , Winslow W , Oddo S ((2017) ) Necroptosis activation in Alzheimer’s disease. Nat Neurosci 20: , 1236–1246. |
[10] | Salvadores N , Moreno-Gonzalez I , Gamez N , Quiroz G , Vegas-Gomez L , Escandón M , Jimenez S , Vitorica J , Gutierrez A , Soto C , Court FA ((2022) ) Aβ oligomers trigger necroptosis-mediated neurodegeneration via microglia activation in Alzheimer’s disease. Acta Neuropathol Commun 10: , 31. |
[11] | Yang SH , Lee DK , Shin J , Lee S , Baek S , Kim J , Jung H , Hah JM , Kim Y ((2017) ) Nec-1 alleviates cognitive impairment with reduction of Aβ and tau abnormalities in APP/PS1 mice. EMBO Mol Med 9: , 61–77. |
[12] | Dong Y , Yu H , Li X , Bian K , Zheng Y , Dai M , Feng X , Sun Y , He Y , Yu B , Zhang H , Wu J , Yu X , Wu H , Kong W ((2022) ) Hyperphosphorylated tau mediates neuronal death by inducing necroptosis and inflammation in Alzheimer’s disease. J Neuroinflammation 19: , 205. |
[13] | Zou C , Mifflin L , Hu Z , Zhang T , Shan B , Wang H , Xing X , Zhu H , Adiconis X , Levin JZ , Li F , Liu CF , Liu JS , Yuan J ((2020) ) Reduction of mNAT1/hNAT2 contributes to cerebral endothelial necroptosis and Aβ accumulation in Alzheimer’s disease. Cell Rep 33: , 108447. |
[14] | Koper MJ , Van Schoor E , Ospitalieri S , Vandenberghe R , Vandenbulcke M , von Arnim CAF , Tousseyn T , Balusu S , De Strooper B , Thal DR ((2020) ) Necrosome complex detected in granulovacuolar degeneration is associated with neuronal loss in Alzheimer’s disease. Acta Neuropathol 139: , 463–484. |
[15] | Ma D , Li Y , Zhu Y , Wei W , Zhang L , Li Y , Li L , Zhang L ((2021) ) Cornel iridoid glycoside ameliorated Alzheimer’s disease-like pathologies and necroptosis through RIPK1/MLKL pathway in young and aged SAMP8 mice. Evid Based Complement Alternat Med 2021: , 9920962. |
[16] | Yuzwa SA , Shan X , Jones BA , Zhao G , Woodward ML , Li X , Zhu Y , McEachern EJ , Silverman MA , Watson NV , Gong CX , Vocadlo DJ ((2014) ) Pharmacological inhibition of O-GlcNAcase (OGA) prevents cognitive decline and amyloid plaque formation in bigenic tau/APP mutant mice. Mol Neurodegener 9: , 42. |
[17] | Park J , Ha HJ , Chung ES , Baek SH , Cho Y , Kim HK , Han J , Sul JH , Lee J , Kim E , Kim J , Yang YR , Park M , Kim SH , Arumugam TV , Jang H , Seo SW , Suh PG , Jo DG ((2021) ) O-GlcNAcylation ameliorates the pathological manifestations of Alzheimer’s disease by inhibiting necroptosis. , eabd. Sci Adv 7: , 3207. |
[18] | Wang Z , Jiang H , Chen S , Du F , Wang X ((2012) ) The mitochondrial phosphatase PGAM5 functions at the convergence point of multiple necrotic death pathways. Cell 148: , 228–243. |
[19] | Ofengeim D , Mazzitelli S , Ito Y , DeWitt JP , Mifflin L , Zou C , Das S , Adiconis X , Chen H , Zhu H , Kelliher MA , Levin JZ , Yuan J ((2017) ) RIPK1 mediates a disease-associated microglial response in Alzheimer’s disease. Proc Natl Acad Sci U S A 114: , E8788–e8797. |
[20] | Motawi TMK , Abdel-Nasser ZM , Shahin NN ((2020) ) Ameliorative effect of necrosulfonamide in a rat model of Alzheimer’s disease: Targeting mixed lineage kinase domain-like protein-mediated necroptosis. ACS Chem Neurosci 11: , 3386–3397. |
[21] | Bradley JR , Pober JS ((2001) ) Tumor necrosis factor receptor-associated factors (TRAFs). Oncogene 20: , 6482–6491. |
[22] | Cabal-Hierro L , Lazo PS ((2012) ) Signal transduction by tumor necrosis factor receptors. Cell Signal 24: , 1297–1305. |
[23] | Sedger LM , McDermott MF ((2014) ) TNF and TNF-receptors: From mediators of cell death and inflammation to therapeutic giants –past, present and future. Cytokine Growth Factor Rev 25: , 453–472. |
[24] | Moujalled D , Strasser A , Liddell JR ((2021) ) Molecular mechanisms of cell death in neurological diseases. Cell Death Differ 28: , 2029–2044. |
[25] | Liu L , Lalaoui N ((2021) ) 25 years of research put RIPK1 in the clinic. Semin Cell Dev Biol 109: , 86–95. |
[26] | Li J , McQuade T , Siemer AB , Napetschnig J , Moriwaki K , Hsiao YS , Damko E , Moquin D , Walz T , McDermott A , Chan FK , Wu H ((2012) ) The RIP1/RIP3 necrosome forms a functional amyloid signaling complex required for programmed necrosis. Cell 150: , 339–350. |
[27] | Rodriguez DA , Weinlich R , Brown S , Guy C , Fitzgerald P , Dillon CP , Oberst A , Quarato G , Low J , Cripps JG , Chen T , Green DR ((2016) ) Characterization of RIPK3-mediated phosphorylation of the activation loop of MLKL during necroptosis. Cell Death Differ 23: , 76–88. |
[28] | Christofferson DE , Yuan J ((2010) ) Necroptosis as an alternative form of programmed cell death. Curr Opin Cell Biol 22: , 263–268. |
[29] | McComb S , Cessford E , Alturki NA , Joseph J , Shutinoski B , Startek JB , Gamero AM , Mossman KL , Sad S ((2014) ) Type-I interferon signaling through ISGF3 complex is required for sustained Rip3 activation and necroptosis in macrophages. Proc Natl Acad Sci U S A 111: , E3206–3213. |
[30] | Thapa RJ , Nogusa S , Chen P , Maki JL , Lerro A , Andrake M , Rall GF , Degterev A , Balachandran S ((2013) ) Interferon-induced RIP1/RIP3-mediated necrosis requires PKR and is licensed by FADD and caspases. Proc Natl Acad Sci U S A 110: , E3109–E3118. |
[31] | Buchrieser J , Oliva-Martin MJ , Moore MD , Long JCD , Cowley SA , Perez-Simón JA , James W , Venero JL ((2018) ) RIPK1 is a critical modulator of both tonic and TLR-responsive inflammatory and cell death pathways in human macrophage differentiation. Cell Death Dis 9: , 973. |
[32] | Zhang J , Yang Y , He W , Sun L ((2016) ) Necrosome core machinery: MLKL. Cell Mol Life Sci 73: , 2153–2163. |
[33] | Davies KA , Tanzer MC , Griffin MDW , Mok YF , Young SN , Qin R , Petrie EJ , Czabotar PE , Silke J , Murphy JM ((2018) ) The brace helices of MLKL mediate interdomain communication and oligomerisation to regulate cell death by necroptosis. Cell Death Differ 25: , 1567–1580. |
[34] | Hildebrand JM , Tanzer MC , Lucet IS , Young SN , Spall SK , Sharma P , Pierotti C , Garnier JM , Dobson RC , Webb AI , Tripaydonis A , Babon JJ , Mulcair MD , Scanlon MJ , Alexander WS , Wilks AF , Czabotar PE , Lessene G , Murphy JM , Silke J ((2014) ) Activation of the pseudokinase MLKL unleashes the four-helix bundle domain to induce membrane localization and necroptotic cell death. Proc Natl Acad Sci U S A 111: , 15072–15077. |
[35] | Petrie EJ , Czabotar PE , Murphy JM ((2019) ) The structural basis of necroptotic cell death signaling. Trends Biochem Sci 44: , 53–63. |
[36] | Wang H , Sun L , Su L , Rizo J , Liu L , Wang LF , Wang FS , Wang X ((2014) ) Mixed lineage kinase domain-like protein MLKL causes necrotic membrane disruption upon phosphorylation by RIP3. Mol Cell 54: , 133–146. |
[37] | Quarato G , Guy CS , Grace CR , Llambi F , Nourse A , Rodriguez DA , Wakefield R , Frase S , Moldoveanu T , Green DR ((2016) ) Sequential engagement of distinct MLKL phosphatidylinositol-binding sites executes necroptosis. Mol Cell 61: , 589–601. |
[38] | Xia B , Fang S , Chen X , Hu H , Chen P , Wang H , Gao Z ((2016) ) MLKL forms cation channels. Cell Res 26: , 517–528. |
[39] | Cai Z , Jitkaew S , Zhao J , Chiang HC , Choksi S , Liu J , Ward Y , Wu LG , Liu ZG ((2014) ) Plasma membrane translocation of trimerized MLKL protein is required for TNF-induced necroptosis. Nat Cell Biol 16: , 55–65. |
[40] | Ofengeim D , Ito Y , Najafov A , Zhang Y , Shan B , DeWitt JP , Ye J , Zhang X , Chang A , Vakifahmetoglu-Norberg H , Geng J , Py B , Zhou W , Amin P , Berlink Lima J , Qi C , Yu Q , Trapp B , Yuan J ((2015) ) Activation of necroptosis in multiple sclerosis. Cell Rep 10: , 1836–1849. |
[41] | Zelic M , Pontarelli F , Woodworth L , Zhu C , Mahan A , Ren Y , LaMorte M , Gruber R , Keane A , Loring P , Guo L , Xia TH , Zhang B , Orning P , Lien E , Degterev A , Hammond T , Ofengeim D ((2021) ) RIPK1 activation mediates neuroinflammation and disease progression in multiple sclerosis. Cell Rep 35: , 109112. |
[42] | Ito Y , Ofengeim D , Najafov A , Das S , Saberi S , Li Y , Hitomi J , Zhu H , Chen H , Mayo L , Geng J , Amin P , DeWitt JP , Mookhtiar AK , Florez M , Ouchida AT , Fan JB , Pasparakis M , Kelliher MA , Ravits J , Yuan J ((2016) ) RIPK1 mediates axonal degeneration by promoting inflammation and necroptosis in ALS. Science 353: , 603–608. |
[43] | Wang T , Perera ND , Chiam MDF , Cuic B , Wanniarachchillage N , Tomas D , Samson AL , Cawthorne W , Valor EN , Murphy JM , Turner BJ ((2020) ) Necroptosis is dispensable for motor neuron degeneration in a mouse model of ALS. Cell Death Differ 27: , 1728–1739. |
[44] | Gouras GK , Tsai J , Naslund J , Vincent B , Edgar M , Checler F , Greenfield JP , Haroutunian V , Buxbaum JD , Xu H , Greengard P , Relkin NR ((2000) ) Intraneuronal Abeta42 accumulation in human brain. Am J Pathol 156: , 15–20. |
[45] | Selkoe DJ ((1998) ) The cell biology of beta-amyloid precursor protein and presenilin in Alzheimer’s disease. Trends Cell Biol 8: , 447–453. |
[46] | Hardy JA , Higgins GA ((1992) ) Alzheimer’s disease: The amyloid cascade hypothesis. Science 256: , 184–185. |
[47] | Greenfield JP , Tsai J , Gouras GK , Hai B , Thinakaran G , Checler F , Sisodia SS , Greengard P , Xu H ((1999) ) Endoplasmic reticulum and trans-Golgi network generate distinct populations of Alzheimer beta-amyloid peptides. Proc Natl Acad Sci U S A 96: , 742–747. |
[48] | Hartmann T , Bieger SC , Brühl B , Tienari PJ , Ida N , Allsop D , Roberts GW , Masters CL , Dotti CG , Unsicker K , Beyreuther K ((1997) ) Distinct sites of intracellular production for Alzheimer’s disease A beta40/42 amyloid peptides. Nat Med 3: , 1016–1020. |
[49] | Xu H , Sweeney D , Wang R , Thinakaran G , Lo AC , Sisodia SS , Greengard P , Gandy S ((1997) ) Generation of Alzheimer beta-amyloid protein in the trans-Golgi network in the apparent absence of vesicle formation. Proc Natl Acad Sci U S A 94: , 3748–3752. |
[50] | Nalivaeva NN , Turner AJ ((2013) ) The amyloid precursor protein: A biochemical enigma in brain development, function and disease. FEBS Lett 587: , 2046–2054. |
[51] | Caporaso GL , Takei K , Gandy SE , Matteoli M , Mundigl O , Greengard P , De Camilli P ((1994) ) Morphologic and biochemical analysis of the intracellular trafficking of the Alzheimer beta/A4 amyloid precursor protein. J Neurosci 14: , 3122–3138. |
[52] | Gralle M , Botelho MG , Wouters FS ((2009) ) Neuroprotective secreted amyloid precursor protein acts by disrupting amyloid precursor protein dimers. J Biol Chem 284: , 15016–15025. |
[53] | Nikolaev A , McLaughlin T , O’Leary DD , Tessier-Lavigne M ((2009) ) APP binds DR6 to trigger axon pruning and neuron death via distinct caspases. Nature 457: , 981–989. |
[54] | Zhang X , Song W ((2013) ) The role of APP and BACE1 trafficking in APP processing and amyloid-β generation. Alzheimers Res Ther 5: , 46. |
[55] | Cline EN , Bicca MA , Viola KL , Klein WL ((2018) ) The amyloid-β oligomer hypothesis: Beginning of the third decade. J Alzheimers Dis 64: , S567–s610. |
[56] | Esparza TJ , Zhao H , Cirrito JR , Cairns NJ , Bateman RJ , Holtzman DM , Brody DL ((2013) ) Amyloid-β oligomerization in Alzheimer dementia versus high-pathology controls. Ann Neurol 73: , 104–119. |
[57] | Viola KL , Klein WL ((2015) ) Amyloid β oligomers in Alzheimer’s disease pathogenesis, treatment, and diagnosis. Acta Neuropathol 129: , 183–206. |
[58] | Lacor PN , Buniel MC , Furlow PW , Clemente AS , Velasco PT , Wood M , Viola KL , Klein WL ((2007) ) Abeta oligomer-induced aberrations in synapse composition, shape, and density provide a molecular basis for loss of connectivity in Alzheimer’s disease. J Neurosci 27: , 796–807. |
[59] | Heneka MT , Carson MJ , El Khoury J , Landreth GE , Brosseron F , Feinstein DL , Jacobs AH , Wyss-Coray T , Vitorica J , Ransohoff RM , Herrup K , Frautschy SA , Finsen B , Brown GC , Verkhratsky A , Yamanaka K , Koistinaho J , Latz E , Halle A , Petzold GC , Town T , Morgan D , Shinohara ML , Perry VH , Holmes C , Bazan NG , Brooks DJ , Hunot S , Joseph B , Deigendesch N , Garaschuk O , Boddeke E , Dinarello CA , Breitner JC , Cole GM , Golenbock DT , Kummer MP ((2015) ) Neuroinflammation in Alzheimer’s disease. Lancet Neurol 14: , 388–405. |
[60] | Busche MA , Hyman BT ((2020) ) Synergy between amyloid-β and tau in Alzheimer’s disease. Nat Neurosci 23: , 1183–1193. |
[61] | Heredia L , Lin R , Vigo FS , Kedikian G , Busciglio J , Lorenzo A ((2004) ) Deposition of amyloid fibrils promotes cell-surface accumulation of amyloid beta precursor protein. Neurobiol Dis 16: , 617–629. |
[62] | Ohgami T , Kitamoto T , Tateishi J ((1992) ) Alzheimer’s amyloid precursor protein accumulates within axonal swellings in human brain lesions. Neurosci Lett 136: , 75–78. |
[63] | Chan HH , Leong CO , Lim CL , Koh RY ((2022) ) Roles of receptor-interacting protein kinase 1 in SH-SY5Y cells with beta amyloid-induced neurotoxicity. J Cell Mol Med 26: , 1434–1444. |
[64] | Vandenabeele P , Grootjans S , Callewaert N , Takahashi N ((2013) ) Necrostatin-1 blocks both RIPK1 and IDO: Consequences for the study of cell death in experimental disease models. Cell Death Differ 20: , 185–187. |
[65] | Xie T , Peng W , Liu Y , Yan C , Maki J , Degterev A , Yuan J , Shi Y ((2013) ) Structural basis of RIP1 inhibition by necrostatins. Structure 21: , 493–499. |
[66] | Brody DL , Jiang H , Wildburger N , Esparza TJ ((2017) ) Non-canonical soluble amyloid-beta aggregates and plaque buffering: Controversies and future directions for target discovery in Alzheimer’s disease. Alzheimers Res Ther 9: , 62. |
[67] | Koffie RM , Meyer-Luehmann M , Hashimoto T , Adams KW , Mielke ML , Garcia-Alloza M , Micheva KD , Smith SJ , Kim ML , Lee VM , Hyman BT , Spires-Jones TL ((2009) ) Oligomeric amyloid beta associates with postsynaptic densities and correlates with excitatory synapse loss near senile plaques. Proc Natl Acad Sci U S A 106: , 4012–4017. |
[68] | Hong S , Ostaszewski BL , Yang T , O’Malley TT , Jin M , Yanagisawa K , Li S , Bartels T , Selkoe DJ ((2014) ) Soluble Aβ oligomers are rapidly sequestered from brain ISF in vivo and bind GM1 ganglioside on cellular membranes. Neuron 82: , 308–319. |
[69] | Binder LI , Frankfurter A , Rebhun LI ((1985) ) The distribution of tau in the mammalian central nervous system. J Cell Biol 101: , 1371–1378. |
[70] | Rojas JC , Boxer AL ((2016) ) Neurodegenerative disease in 2015: Targeting tauopathies for therapeutic translation. Nat Rev Neurol 12: , 74–76. |
[71] | Duka V , Lee JH , Credle J , Wills J , Oaks A , Smolinsky C , Shah K , Mash DC , Masliah E , Sidhu A ((2013) ) Identification of the sites of tau hyperphosphorylation and activation of tau kinases in synucleinopathies and Alzheimer’s diseases. PLoS One 8: , e75025. |
[72] | Braak H , Braak E ((1991) ) Neuropathological stageing of Alzheimer-related changes. Acta Neuropathol 82: , 239–259. |
[73] | Fiandaca MS , Kapogiannis D , Mapstone M , Boxer A , Eitan E , Schwartz JB , Abner EL , Petersen RC , Federoff HJ , Miller BL , Goetzl EJ ((2015) ) Identification of preclinical Alzheimer’s disease by a profile of pathogenic proteins in neurally derived blood exosomes: A case-control study. Alzheimers Dement 11: , 600–607.e601. |
[74] | Chi H , Chang HY , Sang TK ((2018) ) Neuronal cell death mechanisms in major neurodegenerative diseases. Int J Mol Sci 19: , 3082. |
[75] | Gastard MC , Troncoso JC , Koliatsos VE ((2003) ) Caspase activation in the limbic cortex of subjects with early Alzheimer’s disease. Ann Neurol 54: , 393–398. |
[76] | Theofilas P , Ehrenberg AJ , Nguy A , Thackrey JM , Dunlop S , Mejia MB , Alho AT , Paraizo Leite RE , Rodriguez RD , Suemoto CK , Nascimento CF , Chin M , Medina-Cleghorn D , Cuervo AM , Arkin M , Seeley WW , Miller BL , Nitrini R , Pasqualucci CA , Filho WJ , Rueb U , Neuhaus J , Heinsen H , Grinberg LT ((2018) ) Probing the correlation of neuronal loss, neurofibrillary tangles, and cell death markers across the Alzheimer’s disease Braak stages: A quantitative study in humans. Neurobiol Aging 61: , 1–12. |
[77] | Lassmann H , Bancher C , Breitschopf H , Wegiel J , Bobinski M , Jellinger K , Wisniewski HM ((1995) ) Cell death in Alzheimer’s disease evaluated by DNA fragmentation in situ. Acta Neuropathol 89: , 35–41. |
[78] | Lucassen PJ , Chung WC , Kamphorst W , Swaab DF ((1997) ) DNA damage distribution in the human brain as shown by in situ end labeling; area-specific differences in aging and Alzheimer disease in the absence of apoptotic morphology. J Neuropathol Exp Neurol 56: , 887–900. |
[79] | Zhu X , Raina AK , Perry G , Smith MA ((2006) ) Apoptosis in Alzheimer disease: A mathematical improbability. Curr Alzheimer Res 3: , 393–396. |
[80] | Morsch R , Simon W , Coleman PD ((1999) ) Neurons may live for decades with neurofibrillary tangles. J Neuropathol Exp Neurol 58: , 188–197. |
[81] | Ferguson TA , Herndon J , Elzey B , Griffith TS , Schoenberger S , Green DR ((2002) ) Uptake of apoptotic antigen-coupled cells by lymphoid dendritic cells and cross-priming of CD8(+) T cells produce active immune unresponsiveness. J Immunol 168: , 5589–5595. |
[82] | Green DR , Ferguson T , Zitvogel L , Kroemer G ((2009) ) Immunogenic and tolerogenic cell death. Nat Rev Immunol 9: , 353–363. |
[83] | Griffith TS , Yu X , Herndon JM , Green DR , Ferguson TA ((1996) ) CD95-induced apoptosis of lymphocytes in an immune privileged site induces immunological tolerance. Immunity 5: , 7–16. |
[84] | Pasparakis M , Vandenabeele P ((2015) ) Necroptosis and its role in inflammation. Nature 517: , 311–320. |
[85] | Gong YN , Guy C , Olauson H , Becker JU , Yang M , Fitzgerald P , Linkermann A , Green DR ((2017) ) ESCRT-III acts downstream of MLKL to regulate necroptotic cell death and its consequences. Cell 169: , 286–300.e216. |
[86] | Hoque A , Hossain MI , Ameen SS , Ang CS , Williamson N , Ng DC , Chueh AC , Roulston C , Cheng HC ((2016) ) A beacon of hope in stroke therapy-Blockade of pathologically activated cellular events in excitotoxic neuronal death as potential neuroprotective strategies. Pharmacol Ther 160: , 159–179. |
[87] | van de Haar HJ , Burgmans S , Jansen JF , van Osch MJ , van Buchem MA , Muller M , Hofman PA , Verhey FR , Backes WH ((2017) ) Blood-brain barrier leakage in patients with early Alzheimer disease. Radiology 282: , 615. |
[88] | Zlokovic BV ((2011) ) Neurovascular pathways to neurodegeneration in Alzheimer’s disease and other disorders. Nat Rev Neurosci 12: , 723–738. |
[89] | Sweeney MD , Sagare AP , Zlokovic BV ((2015) ) Cerebrospinal fluid biomarkers of neurovascular dysfunction in mild dementia and Alzheimer’s disease. J Cereb Blood Flow Metab 35: , 1055–1068. |
[90] | Zhao Z , Nelson AR , Betsholtz C , Zlokovic BV ((2015) ) Establishment and dysfunction of the blood-brain barrier. Cell 163: , 1064–1078. |
[91] | Bowman GL , Dayon L , Kirkland R , Wojcik J , Peyratout G , Severin IC , Henry H , Oikonomidi A , Migliavacca E , Bacher M , Popp J ((2018) ) Blood-brain barrier breakdown, neuroinflammation, and cognitive decline in older adults. Alzheimers Dement 14: , 1640–1650. |
[92] | Zelic M , Roderick JE , O’Donnell JA , Lehman J , Lim SE , Janardhan HP , Trivedi CM , Pasparakis M , Kelliher MA ((2018) ) RIP kinase 1-dependent endothelial necroptosis underlies systemic inflammatory response syndrome. J Clin Invest 128: , 2064–2075. |
[93] | Fang Y , Gao S , Wang X , Cao Y , Lu J , Chen S , Lenahan C , Zhang JH , Shao A , Zhang J ((2020) ) Programmed cell deaths and potential crosstalk with blood-brain barrier dysfunction after hemorrhagic stroke. Front Cell Neurosci 14: , 68. |
[94] | Chen J , Jin H , Xu H , Peng Y , Jie L , Xu D , Chen L , Li T , Fan L , He P , Ying G , Gu C , Wang C , Wang L , Chen G ((2019) ) The neuroprotective effects of necrostatin-1 on subarachnoid hemorrhage in rats are possibly mediated by preventing blood-brain barrier disruption and RIP3-mediated necroptosis. Cell Transplant 28: , 1358–1372. |
[95] | Yamazaki Y , Kanekiyo T ((2017) ) Blood-brain barrier dysfunction and the pathogenesis of Alzheimer’s disease. Int J Mol Sci 18: , 1965. |
[96] | DeTure MA , Dickson DW ((2019) ) The neuropathological diagnosis of Alzheimer’s disease. Mol Neurodegener 14: , 32. |
[97] | Hemonnot AL , Hua J , Ulmann L , Hirbec H ((2019) ) Microglia in Alzheimer disease: Well-known targets and new opportunities. Front Aging Neurosci 11: , 233. |
[98] | Neniskyte U , Fricker M , Brown GC ((2016) ) Amyloid β induces microglia to phagocytose neurons via activation of protein kinase Cs and NADPH oxidase. Int J Biochem Cell Biol 81: , 346–355. |
[99] | Boche D , Perry VH , Nicoll JA ((2013) ) Review: Activation patterns of microglia and their identification in the human brain. Neuropathol Appl Neurobiol 39: , 3–18. |
[100] | Mandrekar-Colucci S , Landreth GE ((2010) ) Microglia and inflammation in Alzheimer’s disease. CNS Neurol Disord Drug Targets 9: , 156–167. |
[101] | Hong S , Beja-Glasser VF , Nfonoyim BM , Frouin A , Li S , Ramakrishnan S , Merry KM , Shi Q , Rosenthal A , Barres BA , Lemere CA , Selkoe DJ , Stevens B ((2016) ) Complement and microglia mediate early synapse loss in Alzheimer mouse models. Science 352: , 712–716. |
[102] | D’Mello C , Le T , Swain MG ((2009) ) Cerebral microglia recruit monocytes into the brain in response to tumor necrosis factoralpha signaling during peripheral organ inflammation. J Neurosci 29: , 2089–2102. |
[103] | Dopp JM , Mackenzie-Graham A , Otero GC , Merrill JE ((1997) ) Differential expression, cytokine modulation, and specific functions of type-1 and type-2 tumor necrosis factor receptors in rat glia. J Neuroimmunol 75: , 104–112. |
[104] | Habbas S , Santello M , Becker D , Stubbe H , Zappia G , Liaudet N , Klaus FR , Kollias G , Fontana A , Pryce CR , Suter T , Volterra A ((2015) ) Neuroinflammatory TNFα impairs memory via astrocyte signaling. Cell 163: , 1730–1741. |
[105] | Huang Z , Zhou T , Sun X , Zheng Y , Cheng B , Li M , Liu X , He C ((2018) ) Necroptosis in microglia contributes to neuroinflammation and retinal degeneration through TLR4 activation. Cell Death Differ 25: , 180–189. |
[106] | Hao Q , Kundu S , Kleam J , Zhao ZJ , Idell S , Tang H ((2021) ) Enhanced RIPK3 kinase activity-dependent lytic cell death in M1 but not M2 macrophages. Mol Immunol 129: , 86–93. |
[107] | Yang J , Zhao Y , Zhang L , Fan H , Qi C , Zhang K , Liu X , Fei L , Chen S , Wang M , Kuang F , Wang Y , Wu S ((2018) ) RIPK3/MLKL-mediated neuronal necroptosis modulates the M1/M2 polarization of microglia/macrophages in the ischemic cortex. Cereb Cortex 28: , 2622–2635. |
[108] | Wilkins HM , Swerdlow RH ((2021) ) Mitochondrial links between brain aging and Alzheimer’s disease. Transl Neurodegener 10: , 33. |
[109] | Maruszak A , Żekanowski C ((2011) ) Mitochondrial dysfunction and Alzheimer’s disease. Prog Neuropsychopharmacol Biol Psychiatry 35: , 320–330. |
[110] | Bock FJ , Tait SWG ((2020) ) Mitochondria as multifaceted regulators of cell death. Nat Rev Mol Cell Biol 21: , 85–100. |
[111] | Dragicevic N , Mamcarz M , Zhu Y , Buzzeo R , Tan J , Arendash GW , Bradshaw PC ((2010) ) Mitochondrial amyloid-beta levels are associated with the extent of mitochondrial dysfunction in different brain regions and the degree of cognitive impairment in Alzheimer’s transgenic mice. J Alzheimers Dis 20: (Suppl 2), S535–550. |
[112] | Devi L , Ohno M ((2012) ) Mitochondrial dysfunction and accumulation of the β-secretase-cleaved C-terminal fragment of APP in Alzheimer’s disease transgenic mice. Neurobiol Dis 45: , 417–424. |
[113] | Reddy PH , Manczak M , Yin X ((2017) ) Mitochondria-division inhibitor 1 protects against amyloid-β induced mitochondrial fragmentation and synaptic damage in Alzheimer’s disease. J Alzheimers Dis 58: , 147–162. |
[114] | Wang W , Zhao F , Ma X , Perry G , Zhu X ((2020) ) Mitochondria dysfunction in the pathogenesis of Alzheimer’s disease: Recent advances. Mol Neurodegener 15: , 30. |
[115] | Yang Z , Wang Y , Zhang Y , He X , Zhong CQ , Ni H , Chen X , Liang Y , Wu J , Zhao S , Zhou D , Han J ((2018) ) RIP3 targets pyruvate dehydrogenase complex to increase aerobic respiration in TNF-induced necroptosis. Nat Cell Biol 20: , 186–197. |
[116] | Zhang Y , Su SS , Zhao S , Yang Z , Zhong CQ , Chen X , Cai Q , Yang ZH , Huang D , Wu R , Han J ((2017) ) RIP1 autophosphorylation is promoted by mitochondrial ROS and is essential for RIP3 recruitment into necrosome. Nat Commun 8: , 14329. |
[117] | Lin Y , Choksi S , Shen HM , Yang QF , Hur GM , Kim YS , Tran JH , Nedospasov SA , Liu ZG ((2004) ) Tumor necrosis factor-induced nonapoptotic cell death requires receptor-interacting protein-mediated cellular reactive oxygen species accumulation. J Biol Chem 279: , 10822–10828. |
[118] | Arrázola MS , Saquel C , Catalán RJ , Barrientos SA , Hernandez DE , Martínez NW , Catenaccio A , Court FA ((2019) ) Axonal degeneration is mediated by necroptosis activation. J Neurosci 39: , 3832–3844. |
[119] | Tomlinson BE , Kitchener D ((1972) ) Granulovacuolar degeneration of hippocampal pyramidal cells. J Pathol 106: , 165–185. |
[120] | Thal DR , Del Tredici K , Ludolph AC , Hoozemans JJ , Rozemuller AJ , Braak H , Knippschild U ((2011) ) Stages of granulovacuolar degeneration: Their relation to Alzheimer’s disease and chronic stress response. Acta Neuropathol 122: , 577–589. |
[121] | Wiersma VI , van Ziel AM , Vazquez-Sanchez S , Nölle A , Berenjeno-Correa E , Bonaterra-Pastra A , Clavaguera F , Tolnay M , Musters RJP , van Weering JRT , Verhage M , Hoozemans JJM , Scheper W ((2019) ) Granulovacuolar degeneration bodies are neuron-selective lysosomal structures induced by intracellular tau pathology. Acta Neuropathol 138: , 943–970. |
[122] | Hondius DC , Koopmans F , Leistner C , Pita-Illobre D , Peferoen-Baert RM , Marbus F , Paliukhovich I , Li KW , Rozemuller AJM , Hoozemans JJM , Smit AB ((2021) ) The proteome of granulovacuolar degeneration and neurofibrillary tangles in Alzheimer’s disease. Acta Neuropathol 141: , 341–358. |
[123] | Ghoshal N , García-Sierra F , Wuu J , Leurgans S , Bennett DA , Berry RW , Binder LI ((2002) ) Tau conformational changes correspond to impairments of episodic memory in mild cognitive impairment and Alzheimer’s disease. Exp Neurol 177: , 475–493. |
[124] | Hoozemans JJ , van Haastert ES , Nijholt DA , Rozemuller AJ , Eikelenboom P , Scheper W ((2009) ) The unfolded protein response is activated in pretangle neurons in Alzheimer’s disease hippocampus. Am J Pathol 174: , 1241–1251. |
[125] | Lund H , Gustafsson E , Svensson A , Nilsson M , Berg M , Sunnemark D , von Euler G ((2014) ) MARK4 and MARK3 associate with early tau phosphorylation in Alzheimer’s disease granulovacuolar degeneration bodies. Acta Neuropathol Commun 2: , 22. |
[126] | Koper MJ , Tomé SO , Gawor K , Belet A , Van Schoor E , Schaeverbeke J , Vandenberghe R , Vandenbulcke M , Ghebremedhin E , Otto M , von Arnim CAF , Balusu S , Blaschko MB , De Strooper B , Thal DR ((2022) ) LATE-NC aggravates GVD-mediated necroptosis in Alzheimer’s disease. Acta Neuropathol Commun 10: , 128. |
[127] | Buciuc M , Whitwell JL , Tosakulwong N , Weigand SD , Murray ME , Boeve BF , Knopman DS , Parisi JE , Petersen RC , Dickson DW , Josephs KA ((2020) ) Association between transactive response DNA-binding protein of 43 kDa type and cognitive resilience to Alzheimer’s disease: A case-control study. Neurobiol Aging 92: , 92–97. |
[128] | Meneses A , Koga S , O’Leary J , Dickson DW , Bu G , Zhao N ((2021) ) TDP-43 pathology in Alzheimer’s disease. Mol Neurodegener 16: , 84. |
[129] | Van Schoor E , Koper MJ , Ospitalieri S , Dedeene L , Tomé SO , Vandenberghe R , Brenner D , Otto M , Weishaupt J , Ludolph AC , Van Damme P , Van Den Bosch L , Thal DR ((2021) ) Necrosome-positive granulovacuolar degeneration is associated with TDP-43 pathological lesions in the hippocampus of ALS/FTLD cases. Neuropathol Appl Neurobiol 47: , 328–345. |
[130] | Masliah E , Terry R ((1993) ) The role of synaptic proteins in the pathogenesis of disorders of the central nervous system. Brain Pathol 3: , 77–85. |
[131] | Terry RD , Masliah E , Salmon DP , Butters N , DeTeresa R , Hill R , Hansen LA , Katzman R ((1991) ) Physical basis of cognitive alterations in Alzheimer’s disease: Synapse loss is the major correlate of cognitive impairment. Ann Neurol 30: , 572–580. |
[132] | Masliah E , Mallory M , Hansen L , DeTeresa R , Terry RD ((1993) ) Quantitative synaptic alterations in the human neocortex during normal aging. Neurology 43: , 192–197. |
[133] | Scheff SW , Price DA ((1993) ) Synapse loss in the temporal lobe in Alzheimer’s disease. Ann Neurol 33: , 190–199. |
[134] | Masliah E , Hansen L , Albright T , Mallory M , Terry RD ((1991) ) Immunoelectron microscopic study of synaptic pathology in Alzheimer’s disease. Acta Neuropathol 81: , 428–433. |
[135] | Masliah E , Terry RD , DeTeresa RM , Hansen LA ((1989) ) Immunohistochemical quantification of the synapse-related protein synaptophysin in Alzheimer disease. Neurosci Lett 103: , 234–239. |
[136] | Pereira JB , Janelidze S , Ossenkoppele R , Kvartsberg H , Brinkmalm A , Mattsson-Carlgren N , Stomrud E , Smith R , Zetterberg H , Blennow K , Hansson O ((2021) ) Untangling the association of amyloid-β and tau with synaptic and axonal loss in Alzheimer’s disease. Brain 144: , 310–324. |
[137] | Hernández DE , Salvadores NA , Moya-Alvarado G , Catalán RJ , Bronfman FC , Court FA ((2018) ) Axonal degeneration induced by glutamate excitotoxicity is mediated by necroptosis. J Cell Sci 131: , jcs214684. |
[138] | Degterev A , Hitomi J , Germscheid M , Ch’en IL , Korkina O , Teng X , Abbott D , Cuny GD , Yuan C , Wagner G , Hedrick SM , Gerber SA , Lugovskoy A , Yuan J ((2008) ) Identification of RIP1 kinase as a specific cellular target of necrostatins. Nat Chem Biol 4: , 313–321. |
[139] | Qinli Z , Meiqing L , Xia J , Li X , Weili G , Xiuliang J , Junwei J , Hailan Y , Ce Z , Qiao N ((2013) ) Necrostatin-1 inhibits the degeneration of neural cells induced by aluminum exposure. Restor Neurol Neurosci 31: , 543–555. |
[140] | Gao X , Zhang P , Chen J , Zhang L , Shang N , Chen J , Fan R , Wang Y , Huang T , Niu Q , Zhang Q ((2022) ) Necrostatin-1 relieves learning and memory deficits in a zebrafish model of Alzheimer’s disease induced by aluminum. Neurotox Res 40: , 198–214. |
[141] | Jinawong K , Apaijai N , Wongsuchai S , Pratchayasakul W , Chattipakorn N , Chattipakorn SC ((2020) ) Necrostatin-1 mitigates cognitive dysfunction in prediabetic rats with no alteration in insulin sensitivity. Diabetes 69: , 1411–1423. |
[142] | Takahashi N , Duprez L , Grootjans S , Cauwels A , Nerinckx W , DuHadaway JB , Goossens V , Roelandt R , Van Hauwermeiren F , Libert C , Declercq W , Callewaert N , Prendergast GC , Degterev A , Yuan J , Vandenabeele P ((2012) ) Necrostatin-1 analogues: Critical issues on the specificity, activity and in vivo use in experimental disease models. Cell Death Dis 3: , e437. |
[143] | Soliman H , Mediavilla-Varela M , Antonia S ((2010) ) Indoleamine 2,3-dioxygenase: Is it an immune suppressor? Cancer J 16: , 354–359. |
[144] | Zhang J , Liu D , Fu P , Liu ZQ , Lai C , Yang CQ , Chen K , Bao WD , Hu F , Du HY , Yang W , Wang J , Man HY , Lu Y , Zhu LQ (2022) Social isolation reinforces aging-related behavioral inflexibility by promoting neuronal necroptosis in basolateral amygdala. Mol Psychiatry. doi: 10.1038/s41380-022-01694-5. |
[145] | Zhou T , Wang Q , Phan N , Ren J , Yang H , Feldman CC , Feltenberger JB , Ye Z , Wildman SA , Tang W , Liu B ((2019) ) Identification of a novel class of RIP1/RIP3 dual inhibitors that impede cell death and inflammation in mouse abdominal aortic aneurysm models. Cell Death Dis 10: , 226. |
[146] | Chen X , Zhuang C , Ren Y , Zhang H , Qin X , Hu L , Fu J , Miao Z , Chai Y , Liu ZG , Zhang H , Cai Z , Wang HY ((2019) ) Identification of the Raf kinase inhibitor TAK-632 and its analogues as potent inhibitors of necroptosis by targeting RIPK1 and RIPK3. Br J Pharmacol 176: , 2095–2108. |
[147] | Zhang H , Xu L , Qin X , Chen X , Cong H , Hu L , Chen L , Miao Z , Zhang W , Cai Z , Zhuang C ((2019) ) N-(7-Cyano-6-(4-fluoro-3-(2-(3-(trifluoromethyl)phenyl)acetamido)phenoxy)benzo[d]thiazol-2-yl)cyclopropanecarboxamide (TAK-632) analogues as novel necroptosis inhibitors by targeting receptor-interacting protein kinase 3 (RIPK3): Synthesis, structure-activity relationships, and in vivo efficacy. J Med Chem 62: , 6665–6681. |
[148] | Park J , Lai MKP , Arumugam TV , Jo DG ((2020) ) O-GlcNAcylation as a therapeutic target for Alzheimer’s disease. Neuromolecular Med 22: , 171–193. |
[149] | Pan D , Gu JH , Zhang J , Hu Y , Liu F , Iqbal K , Cekic N , Vocadlo DJ , Dai CL , Gong CX ((2021) ) Thiamme2-G, a novel O-GlcNAcase inhibitor, reduces tau hyperphosphorylation and rescues cognitive impairment in mice. J Alzheimers Dis 81: , 273–286. |
[150] | Tu JL , Chen WP , Cheng ZJ , Zhang G , Luo QH , Li M , Liu X ((2020) ) EGb761 ameliorates cell necroptosis by attenuating RIP1-mediated mitochondrial dysfunction and ROS production in both in vivo and in vitro models of Alzheimer’s disease. Brain Res 1736: , 146730. |
[151] | Li XX , Lang XY , Ren TT , Wang J , Lan R , Qin XY ((2022) ) Coeloglossum viride var. bracteatum extract attenuates Aβ-induced toxicity by inhibiting RIP1-driven inflammation and necroptosis. J Ethnopharmacol 282: , 114606. |
[152] | Xu C , Wu J , Wu Y , Ren Z , Yao Y , Chen G , Fang EF , Noh JH , Liu YU , Wei L , Chen X , Sima J ((2021) ) TNF-α-dependent neuronal necroptosis regulated in Alzheimer’s disease by coordination of RIPK1-p62 complex with autophagic UVRAG. Theranostics 11: , 9452–9469. |
[153] | Perga S , Martire S , Montarolo F , Navone ND , Calvo A , Fuda G , Marchet A , Leotta D , Chiò A , Bertolotto A ((2017) ) A20 in multiple sclerosis and Parkinson’s disease: Clue to a common dysregulation of anti-inflammatory pathways? Neurotox Res 32: , 1–7. |
[154] | Gurung P , Man SM , Kanneganti TD ((2015) ) A20 is a regulator of necroptosis. Nat Immunol 16: , 596–597. |
[155] | Petersen SL , Chen TT , Lawrence DA , Marsters SA , Gonzalvez F , Ashkenazi A ((2015) ) TRAF2 is a biologically important necroptosis suppressor. Cell Death Differ 22: , 1846–1857. |
[156] | Vissers M , Heuberger J , Groeneveld GJ , Oude Nijhuis J , De Deyn PP , Hadi S , Harris J , Tsai RM , Cruz-Herranz A , Huang F , Tong V , Erickson R , Zhu Y , Scearce-Levie K , Hsiao-Nakamoto J , Tang X , Chang M , Fox BM , Estrada AA , Pomponio RJ , Alonso-Alonso M , Zilberstein M , Atassi N , Troyer MD , Ho C ((2022) ) Safety, pharmacokinetics and target engagement of novel RIPK1 inhibitor SAR443060 (DNL747) for neurodegenerative disorders: Randomized, placebo-controlled, double-blind phase I/Ib studies in healthy subjects and patients. Clin Transl Sci 15: , 2010–2023. |
[157] | Ryan JM , Quattropani A , Abd-Elaziz K , Daas Id , Schneider M , Ousson S , Neny M , Sand A , Hantson J , Permanne B , Wiessner C , Beher D ((2018) ) Phase 1 study in healthy volunteers of the O-glcnacase inhibitor ASN120290 as a novel therapy for progressive supranuclear palsy and related tauopathies. Alzheimers Dement 14: , P251. |
[158] | Li S , Qu L , Wang X , Kong L ((2022) ) Novel insights into RIPK1 as a promising target for future Alzheimer’s disease treatment. Pharmacol Ther 231: , 107979. |
[159] | Yang SH , Shin J , Shin NN , Hwang JH , Hong SC , Park K , Lee JW , Lee S , Baek S , Kim K , Cho I , Kim Y ((2019) ) A small molecule Nec-1 directly induces amyloid clearance in the brains of aged APP/PS1 mice. Sci Rep 9: , 4183. |