Metal Nanoparticles in Alzheimer’s Disease
Abstract
Nanotechnology has emerged in different fields of biomedical application, including lifestyle diseases like diabetes, hypertension, and chronic kidney disease, neurodegenerative diseases like Alzheimer’s disease (AD), Parkinson’s disease, and different types of cancers. Metal nanoparticles are one of the most used drug delivery systems due to the benefits of their enhanced physicochemical properties as compared to bulk metals. Neurodegenerative diseases are the second most cause affecting mortality worldwide after cancer. Hence, they require the most specific and targeted drug delivery systems for maximum therapeutic benefits. Metal nanoparticles are the preferred drug delivery system, possessing greater blood-brain barrier permeability, biocompatibility, and enhanced bioavailability. But some metal nanoparticles exhibit neurotoxic activity owing to their shape, size, surface charge, or surface modification. This review article has discussed the pathophysiology of AD. The neuroprotective mechanism of gold, silver, selenium, ruthenium, cerium oxide, zinc oxide, and iron oxide nanoparticles are discussed. Again, the neurotoxic mechanisms of gold, iron oxide, titanium dioxide, and cobalt oxide are also included. The neuroprotective and neurotoxic effects of nanoparticles targeted for treating AD are discussed elaborately. The review also focusses on the biocompatibility of metal nanoparticles for targeting the brain in treating AD. The clinical trials and the requirement to develop new drug delivery systems are critically analyzed. This review can show a path for the researchers involved in the brain-targeted drug delivery for AD.
INTRODUCTION
Alzheimer’s disease (AD) is a neurodegenerative disease that causes progressive and irreversible damage to thought, language, and memory. This mental illness occurs not only in people of older age but also in people with chronic diseases like diabetes mellitus [1]. The basic characteristics of this disease are cognitive inability, memory loss, and behavior instability. Generally, the patient does not even remember names, has misperceptions, develops poor communication and more difficulties in speaking, walking, or doing any physical activities, loss of body functions, and lack of self-care [2].
According to the statistics of the World Alzheimer Report, 44 million people had dementia worldwide by 2015, which caused an estimated loss of US$600 billion annually. The incidence was estimated to increase three-fold worldwide by 2050 [3]. It was also reported that the problem had been exacerbated with the baby boomer generation as the size of the older population started to elevate. It was found to increase to 88 million by 2050 if no cure or prevention is planned for AD [4]. AD impacts the patient’s family’s economic and social burden in developing and underdeveloped regions [5].
AD mainly begins with cognitive impairment and then gradually hampers sensory and motor neurons resulting in complete loss of memory and cognition [6]. The pathogenesis of AD involves oxidative stress, neurodegeneration and neuroinflammation, dysregulation of calcium, dysfunction of mitochondria, formation of neurofibrillary tangles, oligomerization of amyloid-β (Aβ), synaptic toxicity, and dyshomeostasis of metal ions like iron, copper, and zinc [7]. AD arises mainly due to the accumulation of hard plaques of Aβ generated from amyloid-β protein precursor (AβPP), neurofibrillary tangles, neuronal dysfunction, generation of reactive oxygen species (ROS), decreased brain energy metabolism, redox brain stress, and neuroinflammation [8]. Reports also indicate that AD patients’ blood-brain barrier (BBB) remains weak, abnormal, and leaky [9]. Currently, various nanotechnology-based techniques have shown favorable results for the diagnosis and treatment of AD. Diagnosis of AD extended to an advanced stage by using ultrasensitive nanoparticles based on biosensors and immune sensors [10].
Nanotechnology acquired huge attention these days [11]. Nanoparticles have been commonly utilized recently, and this technology has been in the limelight since the 1970 s. Due to their enhanced activity, different types of nanoparticles, such as polymeric, metal, and solid lipid nanoparticles, are in recent literature. The unique optical, electrical, and magnetic properties of the metal and metal oxide nanoparticles have made them the most suitable nanotools for application in various fields of biomedical science in therapy, imaging, and as theranostic agents [12]. Metal nanoparticles are gaining importance in the field of theranostics due to the advantages of both therapeutic efficacy and diagnosis of diseases like cancer and neurodegenerative disorders like Parkinson’s disease and AD. Different neurological disorders, including Parkinson’s disease, Huntington’s disease, AD, and dementia, are widely under research for the delivery of brain-targeted drugs. Nanotechnology has been used to provide successful and therapeutic treatment of neurodegenerative disorders [13]. Different nanotools, such as liposomes, niosomes, micelles, polymeric and metal nanoparticles, and solid lipid nanoparticles, have been designed and developed to deliver brain-targeted drugs with increased safety, efficacy, stability, and bioavailability [14, 15].
Among all the nanotools, metal nanoparticles manifest distinctive physicochemical and biological properties compared to their respective particles at higher scales. These unique properties of metal nanoparticles are because of comparatively more surface area to the volume, increased mechanical strength, and enhanced stability or reactivity in the chemical reaction [16]. These colloidal elements integrated with the active pharmaceutical drug are considered a single entity that can exhibit a controlled-release or site-specific drug delivery system [17]. The metal nanoparticles have several advantages that can be useful for treating AD. The BBB is the major problem behind drug targeting for every neurodegenerative disease. The lower permeability of different drugs through BBB limits their treatment by the marketed drugs. The metal nanoparticles can easily cross BBB and possess greater bioavailability, stability, biocompatibility, solubility, and target specificity [18]. It includes a large amount of Gibb’s free energy, a higher surface area, and is multivalent, increasing its adsorption profiles. It exhibited improved biological interaction and coupling with protein or biomolecules [13].
Oxidative stress is one of the target mechanisms in the treatment of AD. The high permeability and biocompatibility of metal nanoparticles like gold, selenium, and ruthenium exhibit an antioxidant activity that facilitates the cognition deficit in the early stages of AD [19, 20]. But some transition metal oxide nanoparticles like iron oxide, copper oxide, cobalt oxide, and titanium dioxide generate ROS in vivo. The reduced metal ions from the nanoparticles participate in the Fenton reaction generating hydrogen peroxide and highly reactive ROS [21]. The metal oxide nanoparticles affect the neuronal viability and interfere with the protein synthesis in the brain by ribosomal RNA, which causes the neurotoxicity and occurrence of AD [22].
The review article aims to elaborate on the neuroprotective and neurotoxic mechanisms of different magnetic and noble metal nanoparticles in AD. Some metal nanoparticles exhibit neuroprotective and neurotoxic activity depending on their size and shape. Hence, this review article will focus on brain-targeted drug delivery systems with metal nanoparticles.
PATHOPHYSIOLOGY OF ALZHEIMER’S DISEASE
AD is a multifactorial neurodegenerative disease that causes several pathophysiological dysfunctions. It has been millions of centuries since AD exists, but its exact etiology is unknown. Physiological abnormalities or changes such as extracellular Aβ production, hyperphosphorylation of tau protein, mitochondrial dysfunction, oxidative stress, cholinergic imbalance, and risk factors like environmental factors, aging, and gene mutations, develop AD-like pathophysiology (Fig. 1) [23].
Fig. 1
Diagrammatic representation of pathogenesis of Alzheimer’s disease (AD). The main pathophysiology for AD is not known. However, deposition of the amyloid-β (Aβ) plaques, formation of neurofibrillary tangles (NFTs) of hyperphosphorylated tau, and presence of oxidative stress are the main causes of AD pathogenesis. Oxidative stress mainly elevates lipid peroxidation, which significantly decreases the antioxidant defense system and causes mitochondrial dysfunction, leading to synaptic plasticity reduction and neurodegeneration. Deposition of amyloid plaques produces toxic and nontoxic Aβ, which increases the levels of cytokines leading to AD formation. Moreover, hyperphosphorylated tau proteins also cause Aβ aggregation resulting in neuronal damage and the development of AD pathogenesis.
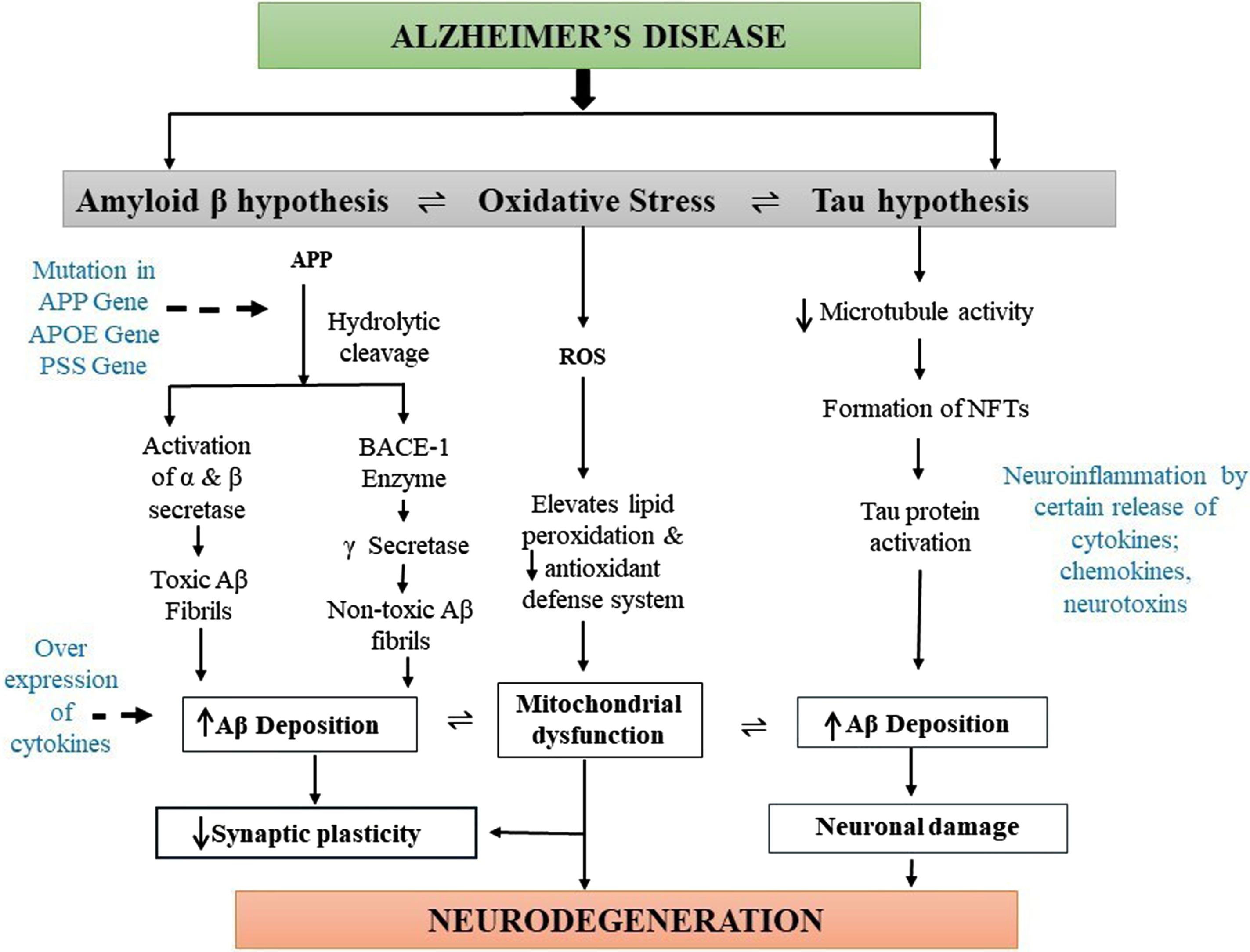
However, these triggering factors of abnormalities are also not well explained in how it causes AD etiology. The first hypothesis which causes AD is the extracellular Aβ which is the most prevailing one and was defined 30 years ago in 1991 [24]. The production and accumulation of Aβ in the brain intrude on the functioning of neurons, which further causes cell death resulting in neurodegeneration. AβPP contains 21 chromosomes, and its pathogenic mutation produces AβPP aberrant metabolism resulting in the formation of Aβ aggregates or deposits [25]. Furthermore, hydrolytic cleavage of AβPP occurs mainly by two enzymes, α- and γ-secretases, which produces toxic soluble Aβ fibrils, and AβPP cleavage intervened by BACE 1 followed by γ-secretase forms insoluble and nontoxic Aβ fibrils, which get deposited in the brain via the immune system. Reportedly, solubilized Aβ oligomers are more cytotoxic than the other isoform, and their long-term potentiation causes loss of synaptic plasticity and memory in various animal models [26]. So, the main causative agent for the occurrence of AD includes soluble Aβ oligomers; however, its main mechanism and their physical and biological characteristics remain unclear.
The tau protein is present in the neurons which belong to microtubule-associated protein and are abundantly present in the nervous system both centrally and peripherally. Tau proteins mainly assist in stabilizing microtubules in the cell cytoskeleton and remain concentrated in the axon part of the neuronal cells. Tau possesses several phosphorylation sites, which favors tau protein phosphorylation. The tau hyperphosphorylation occurs at the S235, S265, and T231 positions of the protein and leads to the formation of the cluster into paired helical filaments leading to the neurofibrillary tangles [24, 27, 28]. These neurofibrillary tangles then accumulate inside neurons due to hyperphosphorylation and disintegrate the microtubule network of the nerve cells. This inhibits the biochemical communication of the nerve cells leading to disruption in the cytoskeleton [29]. It was also observed that hyperphosphorylation of tau proteins enhances Aβ formation, which further promotes neuronal damage [30].
Moreover, a deficiency in the cholinergic neurotransmission in the levels of acetylcholinesterase enzymes and choline acetyltransferase in the cortex and other parts of the brain develops AD-like pathology [31]. Therefore, to enhance the cholinergic function and acetylcholine availability, cholinesterase inhibitor drugs such as tacrine, donepezil, rivastigmine, and galantamine are used for the treatment of AD to inhibit the acetylcholinesterase enzyme, which is responsible for the degradation of acetylcholine activity in the brain synapses [32].
An increase in the activity of glutamate receptors, mediated by the NMDA subtype, results in several neurodegenerative diseases. Excitatory glutaminergic neurotransmission mediated by NMDA receptors is essential for cell viability and synaptic plasticity. Still, mild depolarization of the postsynaptic membrane increases magnesium levels, stimulating NMDA receptors. Then the stimulation causes a sustained influx of calcium (Ca2 +) ions into the postsynaptic neurons of the cortex and subcortical region of the brain, which is generally referred to as “excitotoxicity” and this abnormal signaling of Ca2 + further deteriorates synaptic plasticity and finally, neuronal death which clinically coincides with progressive cognitive decline resulting in AD [33, 34].
Oxidative stress plays an important role in neurodegeneration that worsens and aims at disease progression. Oxidative stress is the imbalance between cellular antioxidants and pro-oxidants which interferes with normal mitochondrial activity [35]. Various factors, such as aging, injury, shock, disease conditions, and environmental factors, are responsible for the formation of uncontrolled and excessive ROS, interrupting normal cellular homeostasis. Mitochondrial dysfunction includes one of the major driving factors for the formation of ROS as mitochondria produce different intermediates during their TCA cycle, such as pyruvates and malate, which thereby improves cell longevity and maintains the antioxidant redox signaling system, and the destruction of these metabolites leads to the generation of ROS [36, 37]. The ATP produced by the mitochondria generates membrane potential along the axon and helps in neurotransmission. The glucose metabolism in the AD brain causes imbalances in the Ca2 + buffering, oxidative phosphorylation, ROS production, and a decrease in the number of mitochondria at the synapse and mitochondrial DNA mutation, which occurs much earlier than the onset of the pathophysiology of AD [38–40]. Increased oxidative stress and ROS level also develop AD-like pathology by enhancing other pathological conditions of AD such as Aβ aggregation, tau hyperphosphorylation, and finally neuronal damage [41]. Inflammatory responses of microglia and astrocytes develop neuroinflammation, which worsens AD progression [42]. Hyperactivity of microglial cells in the brain aggregates Aβ, neurofibrillary tangles, and damage neurons about 2.5-fold higher compared to a healthy brain. The over-expression of microglial cells also mediates the release of various inflammatory cytokines such as TNF-α, IL-1β, IL-6, IL-8, and IL-12, which is responsible for causing severe neuroinflammation resulting in AD development [43, 44]. Activation of microglia is brought about by Aβ plaques, which results in a release of inflammatory mediators, which causes neuroinflammation [45].
Genetic inheritance is one of the causes of AD, and the symptoms are seen between 30–50 years of age. The mutation of any of the genes encoding APP, presenilins 1 and 2, leads to an error in the cleavage of AβPP. This phenomenon forms Aβ42 rather than Aβ40, forming the senile plaques resulting in AD [46]. Autosomal dominant genes (SORL1 and ABCA7) rarely involve AD [47]. The allele ɛ4 of Apolipoprotein E found in homozygotes and heterozygotes increases the risk of AD by 15% and 3%, respectively. Genome-wide association studies report the influence of 19 genes (ABCA7, BIN1, CASS4, CD2AP, CELF1, CLU, CR1, EPHA1, FERMT2, HLA-DRB5, INPP5D, MEF2 C, MS4A, NME8, PICALM, PTK2B, SlC24A4, SORL1, and ZCWPW1) as the risk factors of AD [47].
Another factor responsible for AD is the deficiency of vitamin B5. The decrease in vitamin B5 causes acute impairment in the middle temporal gyrus and entorhinal cortex, whereas the hippocampus is severely affected by a lack of vitamin B5. Vitamin B5 is the precursor of acetyl-coenzyme A which is the precursor of acetylcholine and one of the active constituents of fatty-acyl groups, which controls the insulator function of the neural myelin sheath. Hence deficiency of vitamin B5 affects the concentration of acetylcholine and the abnormal functioning of the myelin sheath leading to neurodegeneration and dementia [48].
The transactive response DNA binding protein (TDP-43) is one of the causes of degeneration of the frontotemporal lobe. Many reports suggested the influence of TDP-43 residues in the brains of AD patients [49]. The concentration of TDP-43 or phosphorylated TDP-43 is high in AD patients [50]. TDP-43 attaches to RNAs of the axonal genes, which controls the aggregation of TDP-43 at the terminal of the axon [51]. The overexpression of TDP-43 activates GSK-3β and impairs cellular homeostasis and protein-protein interaction [52]. The cleavage of the C-terminal of TDP-43 by calpain forms the N-terminal fragment, which aggregates and results in the accumulation of TDP-43[53]. Aβ and the intracellular domain of APP (AICD) with TDP-43 upregulate the expression of p53 mRNA and stimulate the apoptosis induced by AICD. TDP-43 and Aβ share similar epitomes; hence TDP-43 and Aβ both produce amyloid oligomers [54]. The deposition of TDP-43 is increased when associated with hyperphosphorylated tau proteins [55].
The inhibition of synaptic plasticity induced by Aβ oligomers is facilitated by an unknown mechanism’s expression of Fyn kinases [56]. The loss of synapse and onset of cognitive impairment is augmented by overexpression of Fyn. Histology studies of brain sections from AD patients show enhanced Fyn staining in neural cells in the presence of hyperphosphorylated tau protein [57].
Reactive astrocytes are also responsible for the pathogenesis of AD. Astrocytes carry out the clearance of aggregates of oligomers of Aβ. Hence to reduce the toxic effect of Aβ, the drugs activate the like transcription of nuclear factor (erythroid-derived 2)-like 2 (Nrf-2) in astrocytes for therapeutic activity in AD. But, simultaneously, activating such receptors may change the metabolism of neurons and produce excess toxic metabolites and ROS inhibitory factors. These factors convert the astrocytes as neurotoxic; hence the activation of antioxidant mechanisms must be properly regulated [58].
ROLE OF METAL NANOPARTICLES IN ALZHEIMER’S DISEASE
Metal nanoparticles exhibit distinct mechanisms for application in several neurodegenerative diseases. After administration, metal nanoparticles are readily absorbed into the body and different organs, including the brain [5]. However, these metal nanoparticles improve the absorption, bioavailability, and biocompatibility rate due to their high surface area, size ranging from 1-200 nm, and unique physicochemical characteristics compared to bulk metal [59]. These nanoparticles can be supplemented through different routes of administration such as oral, intratracheal, intravenous, intraperitoneal, and intranasal administration [60].
Metal nanoparticles are mainly categorized into two classes: magnetic nanoparticles like iron (Fe), Cobalt (Co), Copper (Cu), Nickel (Ni), Titanium (Ti), and Zinc (Zn) nanoparticles; and noble metal nanoparticles such as gold (Au), Silver (Ag), Platinum (Pt), and Ruthenium (Ru) [15]. However, these metals can also induce neurotoxicity and can aggravate AD-like pathology by various mechanisms like inducing oxidative stress, neuroinflammation, and cell death; however, some metals like Au, Se, and Zn possesses beneficial effect which can treat AD effectively [61]. The detailed effect of each metal nanoparticle exhibiting the preventive or toxic effect for AD has been discussed as follows:
Neuroprotective mechanism of metal nanoparticles for Alzheimer’s disease
The neuroprotection by regulating different metabolic processes by metal nanoparticles involves inhibition of ROS and reactive nitrogen species (RNS) production by regulating the release of mitochondrial cytochrome C. Metal nanoparticles suppress microgliosis or activation of microglia and macrophages in the brain cells [62]. Hyperphosphorylation of tau proteins and deposition of Aβ aggravates the symptoms of AD. Hence reduction in the development of Aβ plaques, decomposition of Aβ fibrils into nontoxic fibrils, suppression of intracellular Aβ40 fibrillation, and Zn2 +-Aβ mediated generation of ROS, inhibition of tau hyperphosphorylation is the primary mechanism-based approaches of metal nanoparticles for neuroprotective action (Fig. 2) Neuroinflammation leads to the development of plasticity and neuronal death, enhancing the risk of AD [63]. The metal nanoparticles reduce the level of proinflammatory cytokines like TNF-α, IL-6, and IL-1β and downregulate NF-κB and IκB enzymes [64]. The metal nanoparticles can control the death of neurons by reducing caspase enzyme levels and inhibiting apoptosis. The decrease in levels of acetylcholinesterase enzyme also alleviates the symptoms of AD [65]. Metal nanoparticles elevate the levels of brain-derived neurotrophic factor, hippocampal protein, and phosphorylated-cAMP response element binding protein which helps in neuroprotection [62, 65]. The reports of the neuroprotective activity of metal nanoparticles are assembled in Table 1.
Fig. 2
Neuroprotective and neurotoxic mechanisms of metal nanoparticles affecting Alzheimer’s disease (AD). The mechanisms of action of different metal nanoparticles against AD are discussed. Some of the metal nanoparticles such as Au, ZnO, Se, Ag, CeO2, and Ru possess neuroprotective action by modulating oxidative stress activity, acetylcholinesterase levels, and inflammation. In contrast, Cu, NO, SnO2, TiO2, and Fe2O3 are responsible for the occurrence of AD by increasing the amyloid-β plaque formation, neurofibrillary tangles of hyperphosphorylated tau, necrosis and apoptosis, and degeneration in the neuronal activity and the levels of reactive oxygen species.
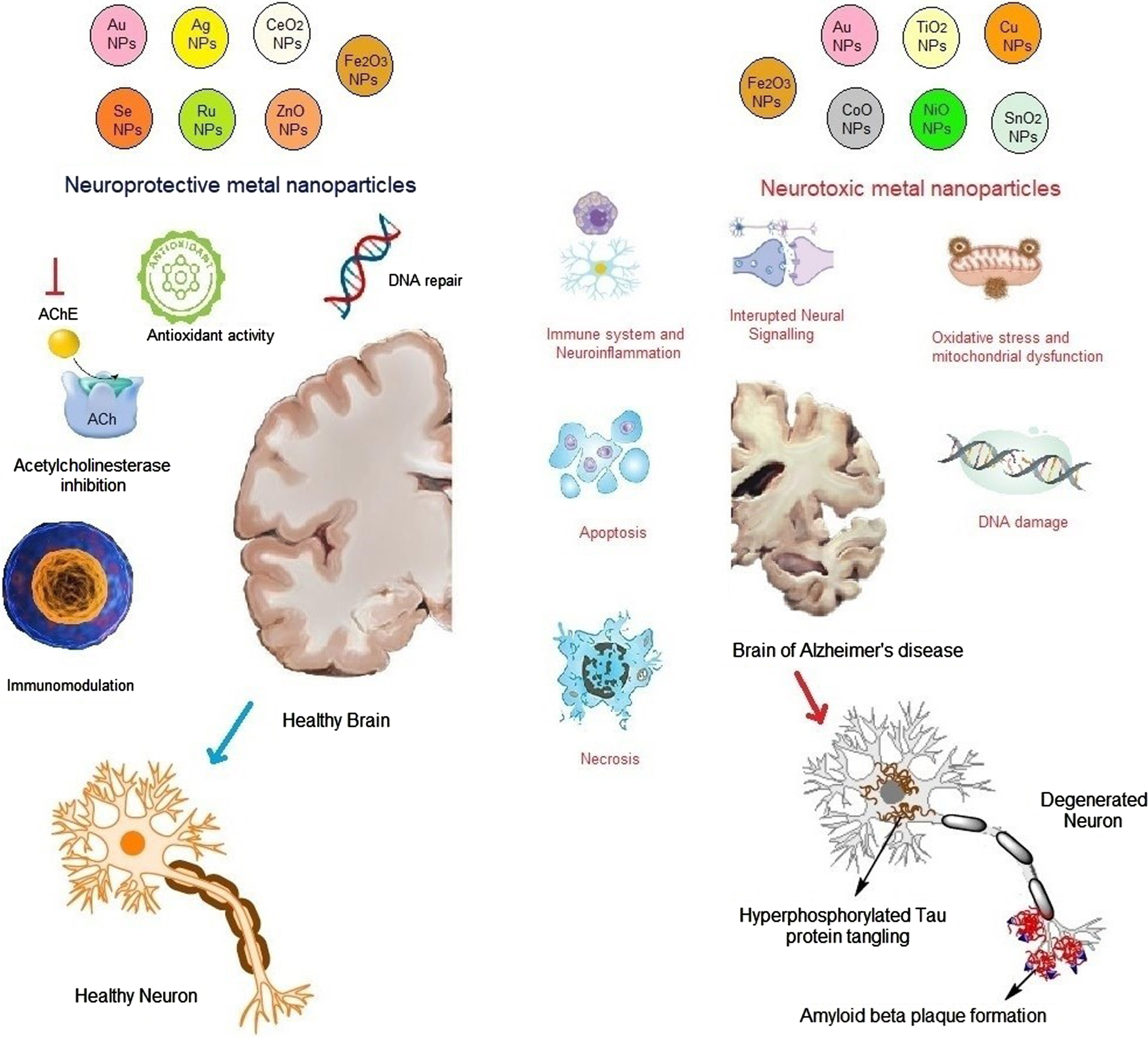
Table 1
Metal nanoparticles with neuroprotective activity
Sl no. | Metal nanoparticles | Sources of nanoparticles | Characterization of the nanoparticle | Route of administration | Dose | Mechanism of action | References |
1. | AgNPs | Nepenthes khasiana | Spherical shape, moderately polydisperse, size range of 10-15 nm | Intraperitoneal | 2.5 mg/kg | It prevented the cognitive deficit in learning and recognition | [144] |
2. | Lampranthuscoccineus, Malephoralutea | Mean particle size ranges between 12.86-28.19 nm | Oral | 200 mg/kg | Inhibits AChE enzyme activity and oxidative stress by increasing the levels of GSH and decreasing MDA levels | [145] | |
3. | Sideritis species (S. argyrea, S. brevidens, S. lycia) | Spherical and monodispersed in shape with an average particle size of 22-26 nm | Oral | 5.46 and 18.31 mg/kg | Decreases activity of butyrylcholinesterase and tyrosinase enzymes | [146] | |
4. | AuNPs | Oral | 2.5 mg/kg | Decreases tau hyperphosphorylation and IL-1β factor. Improved spatial memory, brain and nerve neurotropic factors in the cortex and hippocampus. Restored antioxidant enzymes and decreased mitochondrial activity and ATP synthase activity | [147] | ||
5. | Terminalia arjuna | Spherical, triangular, cubic crystalline-shaped AuNPs with sizes ranging from 20 and 50 nm. Surface charge – 45 mv | In vitro study | Antioxidant, AChE, and anti-amyloidogenic effect | [148] | ||
6. | Mimosine (a non-protein amino acid found in plants) | The average diameter of the NP is 8-12 nm and spherical with size ranging from 6 nm | In vitro study | Suppresses Aβ aggregation and increases neuronal viability by decreasing tau phosphorylation, and production of free radicals | [149] | ||
7. | Chiral AuNPs (L- and D-glutathione stabilized AuNPs) | Intravenous | 25 mg/kg-1 | Inhibits Aβ in in vitro, in vivo, and cellular toxicity models. Improves biodistribution and spatial memory function | [150] | ||
8. | SeNPs (curcumin and SeNPs loaded PLGA nanosphere) | Curcuma longa | Spherical in shape with size ranging from 70.5±6 nm. | In vitro study | Decreases Aβ plaque production in the brain | [151] | |
9. | SeNPs conjugated with two targeting peptides LPFFD and TGN in a ratio 1 : 1 (L1T1 SeNPs) | LPFFD and TGN | The round shape of moderate uniform particle size measuring 100 nm | In vitro study | Reduces extracellular Aβ fibrillation, Aβ40 fibers generating ROS, cytotoxicity, apoptosis rate | [152] | |
10. | SeNPs | Resveratrol (After nanoformulation of Res@SeNPs, it was decorated with BBB peptide TGN peptide (TGN-Res@SeNPs) | Uniform particle size ranging from 14±0.12 nm | Oral | Inhibits AD pathogenesis by decreasing Aβ aggregates in the hippocampus and increasing functional activity of gut microbiota | [153] | |
11. | Resveratrol | Oral | Ameliorates impaired oxidative markers and mitochondrial dysfunction. Increases Aβ clearance neurite outgrowth, expression of Sirtuin-1. Downregulates signal transducer, an activator of transcription, neuroinflammation, and IL-1β | [154] | |||
12. | Monodisperse spherical structure with particle diameter less than 50 nm | Oral | 0.4 mg/kg | Improves spatial memory function, decreases deposition of Aβ plaques, enhances BDNF level and cell survival in the hippocampus | [155] | ||
13. | Epigallocatechingalate with Tet-1 (Tet-1-EGCG@Se) | The average diameter of the particle size was found to be 25 and 29 nm | In vitro study | Reduced Aβ aggregation and Aβ induced cytotoxicity | [156] | ||
14. | RuNPs | Nerve growth factor | The average particle diameter of the NP was found to be about 140 nm measuring with 122 nm particle size | Intracranially | 20μg/ml | Inhibits tau phosphorylation and aggregation, reduces oxidative stress, arrests the rate of apoptosis alleviates microgliosis and enhances memory and learning impairment | [87] |
15. | In vitro study | Promotes neuron regeneration and Aβ clearance, inhibits Aβ plaque formation and Aβ-induced cytotoxicity | [89] | ||||
16. | ZnONPs | The average diameter of the NP is around 20-200 nm | Intraperitoneal | Downregulates Aβ plaque formation and levels of proinflammatory cytokines like IL-6 and IL-18 | [85] | ||
17. | Sabalblack burniana | It possesses different shapes predominantly hexagonal and spherical narrow particles measuring from 2.23-49.56 nm | In vitro study | Inhibits AChE levels significantly | [86] | ||
18. | Fe2O3NPs | PEGylated superparamagnetic Fe2O3 NP | Intraperitoneal and Intra hippocampal | 0.01-0.1μg/kg-1 and 1-10μg/kg-1 | Downregulates Aβ aggregation and fibrillation with improvement in spatial memory functions increases all brain-related factors like BDNF and p-CERB | [95] | |
19. | Fibrin γ377 - 395 peptide conjugated with Fe2O3 NP | The average mean diameter of the NP is 21±3.5 nm | Intracranial | Fibrin γ377 - 395 peptide conjugated with Fe2O3 NP | The average mean diameter of the NP is 21±3.5 nm | [92] | |
20. | CeO2NPs | Spherically shaped and ranged in size from 20 to 25 nm | Oral | Spherically shaped and ranged in size from 20 to 25 nm | [157] |
AChE, acetylcholinesterase; AD, Alzheimer’s disease; Aβ, amyloid-β; BBB, blood-brain barrier; BDNF, brain-derived neurotrophic factor; GSH, glutathione; IL, interleukin; MDA, malondialdehyde; NP, nanoparticle; ROS, reactive oxygen species.
Mechanism of gold nanoparticles in Alzheimer’s disease
The gold nanoparticle is considered the most eminent metal nanoparticle with several applications in diagnosing tumor markers and microbial pathogens, and for many imaging techniques, gene therapies, cancer therapies, and drug delivery systems [66]. Drug delivery across the BBB is a major problem in neurodegenerative diseases. Gold nanoparticles are important in drug delivery systems and induce neuronal activities [67]. It was reported that gold nanoparticles possess antioxidant and anti-inflammatory properties, which are used to treat various neurodegenerative diseases [67]. It also suppresses microglial and macrophage activation in the brain cells [62], decreases tumor necrosis factor-α (TNF-α) in the hippocampal region, reduces the ROS in the rat brain by modulating mitochondrial cytochrome C release, and decreases apoptosis by reducing caspase enzymes levels [67]. Gold nanoparticles reduce NF-κB and IκB enzyme activity in several in vitro studies [68]. Gold nanoparticles exhibit effective results against oxidative stress by modulating the hydrogen peroxide and superoxide anion levels, thereby increasing antioxidant defense systems [69].
Mechanism of silver nanoparticles in AD
Silver nanoparticles can inhibit the production of extraneous ROS without any substrate. It also helps to maintain the mitochondrial activation for adenosine triphosphate (ATP) formation and hydrogen peroxide in return for adenosine diphosphate (ADP) and analogous succinate. Complex I in the mitochondria promotes the production of ROS [70]. Complex-I in the mitochondrial electron chain transport reduces the ubiquinone to ubiquinol (CoQ⟶CoQH2) and oxidizes to form NADH to NAD+ [71]. It was also reported in some theories that this metal nanoparticle performed antioxidant activity by preventing RNS, ROS, and chelator-free radicals [72]. It leads to a decrease in the formation of RNS and ROS and prevents the release of proinflammatory cytokines.
Mechanism of cerium dioxide nanoparticles in AD
It is reported that cerium dioxide nanoparticles have protective effects against oxidative stress in AD. The reports reported the potential antioxidant role of cerium dioxide nanoparticles in AD [73]. Cerium dioxide nanoparticles decrease the level of development of Aβ plaques and oxidative stress in cortical neurons via the regulation of mitochondrial function [74]. CeNP@MnMoS4 core-shell nanoparticles decreased the Aβ aggregation induced by Cu2 + and reduced ROS production [75]. So, cerium dioxide nanoparticles can be the potential lead for preventing AD pathophysiology.
Mechanism of selenium nanoparticles in AD
Selenium is one of the essential trace elements with vast physiological and pharmacological effects required by the body. Selenium nanoparticles have various effectual characteristics that display biomedical applications and preventive effects on several diseases, including treating AD. [76] Therapeutic applications of selenium nanoparticles include antidiabetic and anticancer activities. Selenium nanoparticles display efficient antioxidant properties as they improve antioxidant defense mechanisms and bioenergetics and reduce oxidative stress. Selenium nanoparticles have an important role in the regulation of the redox system. There is a correlation between increased cognitive decline with decreased selenium levels. The selenium nanoparticles perform an important mechanism for inhibiting ROS production [77]. Selenium nanoparticles increase neuronal longevity by regulating the oxidative defense system, inflammatory reactions, cellular metabolic state, and functional properties of hippocampal neurons [78]. It is also reported that selenium nanoparticles have greater bioavailability and biological activity with decreased cytotoxicity [79]. It was also found that selenium nanoparticles significantly decompose Aβ fibrils into nontoxic fibrils, reduce tau hyperphosphorylation, and alleviate neuroinflammation, resulting in the downregulation of AD progression [80].
Mechanism of zinc oxide nanoparticles in AD
Zinc oxide is a micronutrient that interacts with several enzymes in different metabolic processes in the body. Zinc is also involved with the folding of proteins and the expression of genes [81]. The extracellular aggregation of Aβ and intracellular tangle formation of neurofibrils leads to the pathogenesis of AD. Zinc is found in presynaptic vesicles and is released along with glutamate upon neuronal excitation [82]. Different enzymes involved in the degradation of Aβ are zinc metalloproteases, like neprilysin, insulin-degrading enzymes, matrix metalloproteinases, and endothelin-converting enzymes. Zinc also regulates the NMDA and AMPA receptors. Depletion in zinc levels causes increased amyloid plaque formation, neurofibrillary tangling, and neuronal damage, causing aggravation of AD [83, 84].
Moreover, it was reported that zinc oxide nanoparticles could deliver zinc into the brain across BBB. The zinc oxide nanoparticles significantly reduce amyloid plaque formation, decrease inflammatory markers such as IL-6 and IL-18, alleviate hyperlocomotion, and improve spatial memory, learning, and synaptic density [85]. It also possesses an inhibitory effect against the acetylcholinesterase enzyme, and all these results signify that zinc oxide nanoparticles exhibit potential therapeutic activity against AD [86].
Effect of ruthenium nanoparticles in AD
Among all inorganic transition metal nanomaterials, ruthenium nanoparticles have achieved an excellent profile due to their biocompatibility, large surface energy, and good packaging efficiency [87]. Ruthenium nanoparticles also exhibited a good photothermal effect under near-infrared irradiation, increasing their efficiency to possess better permeability across BBB and temperature-responsive release of drugs [88]. It was also reported that ruthenium nanoparticles possess an inhibitory effect against hyperphosphorylated tau protein, tau aggregation, oxidative stress, microgliosis, and neuronal apoptosis and improve impaired learning and memory function [88]. Ruthenium nanoparticles are also responsible for inhibiting Aβ fibrils, Aβ-induced cytotoxicity, and enhancing neuronal outgrowth, which can be used significantly in treating and preventing AD [89]. It also suppresses Zn2 +-Aβ mediated generation of ROS, intracellular Aβ40 fibrillation, and their corresponding neurotoxicity in PC12 cells [90].
Effect of iron oxide nanoparticles in AD
It was detected that iron deposits were found in the amyloid plaques in magnetic resonance imaging reports, and larger plaques possess a huge amount of iron content which requires greater than a 7-Tesla magnetic field and various hours as acquisition time [91]. Some studies demonstrated that the administration of iron oxide nanoparticles significantly inhibited the microglial activity in the Tg4510 tau mutant mice model in vivo. When administered with superparamagnetic iron oxide nanoparticles, combined nerve growth factor, and quercetin promoted the outgrowth of neurites and enhanced the complexity of neuronal branching trees in PC12 cells, which may have therapeutic potential for AD [93]. The superparamagnetic iron oxide nanoparticles conjugating with Aβ oligomer antibody and class A scavenger receptor activator also inhibits Aβ oligomers induced cytotoxicity and increases microglial phagocytosis of Aβ. It improves cognitive function significantly and alleviates AD neuropathology in the mice model [94]. It was also observed that PEGylated superparamagnetic iron oxide nanoparticles ameliorated memory and learning deficit by inhibiting Aβ aggregation and elevating the levels of hippocampal protein, brain-derived neurotrophic factor, and phosphorylated-cAMP response element binding protein [95]. All these studies prove that iron oxide nanoparticles can be used to treat AD.
Neurotoxic mechanisms of metal nanoparticles for Alzheimer’s disease
Though metal nanoparticles are reported as neuroprotective and anti-inflammatory for neurons, several reports of neurotoxicity by metal nanoparticles are reported. The metal nanoparticles accumulate in brain cells and cause neurotoxicity through different mechanisms illustrated in Fig. 2. They trigger the generation of ROS and induce protein oxidation, and lipid peroxidation increases the malondialdehyde content in the cortex and hippocampal regions of the temporal lobe, leading to neurodegeneration [96]. ROS elevates the expression of hypoxia-induced factor alpha 1, causes damage to mitochondria, diminishes ATP content, brings about morphological damage, neuronal cell damage, and induces autophagy leading to neuronal cell death [97]. The metal nanoparticles stimulate the apoptosis-related caspase pathways leading to neuronal cell death. Damage in DNA by triggering DNA fragmentation also leads to cell death by metal nanoparticles. The increase in acetylcholinesterase levels leads to neurodegeneration. Neuroinflammation is also another primary factor involved in AD. Certain metal nanoparticles like cobalt oxide nanoparticles promote the activity of heme oxygenase-1 and p-45-related factor-2 protein levels, which cause neuroinflammation in the glial cells, damaging adjacent neurons. Cobalt oxide nanoparticles increase the expression of microglial marker Iba-1 and NADPH oxidase in the hippocampus leading to neuronal damage [98]. The increase in expression of inflammatory cytokines like TNF-α, IL-1β, and NLRP3 favors the neuroinflammation following neurodegeneration. An increase in Aβ (Aβ40) production in the hippocampal region and an increase in Aβ fibrillization due to increased Aβ42 aggregation aggravates AD. Upregulation of phosphorylated and unphosphorylated tau protein levels in the cortex and hippocampus also worsens the symptoms of AD. Some metal nanoparticles, like cobalt oxide nanoparticles, decrease the weight of the brain and Nissl bodies leading to neural damage [99]. A detailed section of neurotoxicity induced by different metal nanoparticles is discussed in the following section.
Mechanisms of gold nanoparticles in AD
The neurotoxicity of gold nanoparticles is size and shape-dependent in the brain. Smaller-size gold nanoparticles in the 1-5 nm range are neurotoxic as the permeability through the BBB is increased by altering endothelial components of tight junctions and accumulating nanoparticles. The mechanism of neurotoxicity of gold nanoparticles involves disturbance of antioxidants and depletion of antioxidant enzymes in the body leading to oxidative stress, disruption of lipid bilayer causing membrane disruption, causing necrosis and apoptosis, microbial dysfunction, and surface charge of gold nanoparticles leading to DNA damage [100]. The shape of gold nanoparticles has a greater role in neurotoxicity of gold nanoparticles. The star-shaped nanorods and spherical gold nanoparticles studied by Steckiewicz et al. reported that the star-shaped gold nanoparticles are more potentially cytotoxic than spherical or rod-shaped gold nanoparticles [101]. Surface modification of gold nanoparticles with polymers is reported to exert neurotoxic activity [102]. The neurotoxic effect of gold nanoparticles is dependent on surface roughness. Liu et al. studied the toxicity of gold nanoparticles having a rough surface in the hippocampus of a mouse model. He synthesized three types of gold nanoparticles viz, nano-flowers, nanospheres, and nano-triakisoctahedron, and evaluated the effect of gold nanoparticles on the action potential and voltage-dependent sodium channel of hippocampal CA1 neurons in mice. The nano-flowers were found to be more neurotoxic as compared to nanospheres and nano-triakisoctahedron [102].
Mechanisms of iron oxide nanoparticles in AD
Some studies reported that the administration of iron oxide nanoparticles deteriorates memory and cognition, induces oxidative stress, decreases neuronal viability, and activates the apoptosis-related caspase pathways, which can thereby develop AD-like pathology [103]. Fahmy et al. reported the green synthesis of magnetic iron oxide nanoparticles and studied the neurotoxicity of the iron oxide nanoparticles in different brain regions. The hippocampus and striatum were mostly affected by neurodegeneration due to oxidative stress-induced damage [104]. Fernández-Bertólez evaluated the neurotoxic effect of iron oxide nanoparticles in SH-SY5Y neural cell lines. The cytotoxicity of oleic acid-coated iron oxide nanoparticles was reported due to membrane disruption, cell cycle impairment, and induction of cell death [105]. Wu et al. reported the neurotoxic effect of iron oxide nanoparticles in the striatum and hippocampus of rat brains after nose-to-brain delivery. The neurotoxicity was contributed by induction of oxidative stress, cell cycle disruption, decreased neuronal viability, and activation of JNK-pathway and p53-mediated pathway controlling cell cycle and apoptosis [106].
Mechanisms of titanium dioxide nanoparticles in AD
Titanium dioxide nanoparticles are extensively utilized and accumulated in different organs in the body like the kidney, liver, and spleen with the associated toxicity [107]. The possible brain toxicity concerned with titanium dioxide nanoparticles is that they can pass through the BBB and can accumulate in the total brain [108]. It induces inflammation, oxidative stress, and apoptosis, possibly leading to neurodegenerative diseases. It also activates different signaling pathways leading to the activation of microglia, loss of mitochondrial membrane potential, generation of ROS, neuroinflammation, and cell death [109]. Due to high metabolic demand and deficient antioxidant systems, titanium dioxide nanoparticles induced toxicity in the brain by oxidative stress. Titanium dioxide nanoparticles are highly reactive and have a small particle size. Exposure to titanium dioxide nanoparticles enhances the production of ROS [110]. Oxidative stress causes cellular imbalance where the production of ROS surpasses the antioxidants, leading to inducing protein oxidation, lipid peroxidation, DNA damage, and RNA-causing cell death [111].
Mechanism of copper nanoparticles in AD
Copper and copper oxide nanoparticles are advantageous for their desired properties as they have large surface areas and excellent electrical, catalytic, and very good optical and microbial properties. But notably, copper nanoparticles are toxic and cause neurodegeneration by triggering DNA fragmentation and cell death [112]. It was observed that when human neuroglioma brain cells are subjected to copper nanoparticles, it produces significant cell damage in a dose-dependent manner [113]. It also inhibits voltage-gated Na+ channels in the hippocampal CA1 neuronal cells resulting in the prolongation of action potential and decreasing overall amplitude, further alleviating the normal functioning of neurons and cells [114]. In an in vivo study, it was observed that copper nanoparticles significantly affect spatial learning and memory function and induce oxidative stress by enhancing the ROS level and malondialdehyde content in the rat hippocampus. It was also found that cooper nanoparticles increase the acetylcholinesterase levels in the brain, spleen, liver, and blood and upgrade the Aβ production in the hippocampal region, which is the chief contributing factor of AD [115].
Mechanism of cobalt oxide nanoparticles on AD
Cobalt oxide nanoparticles were determined to elevate malondialdehyde content by increasing lipid peroxidation levels in both the hippocampal and cortex regions of the temporal lobe. It also decreases cell viability and cell proliferation and increases the rate of caspase 9 and apoptosis, leading to cell death. Exposure to cobalt oxide nanoparticles promotes heme oxygenase-1 and p-45-related factor-2 protein levels [98]. Additionally, it adversely affects BBB during its translocation into central nervous system parenchyma and causes neuroinflammation in the glial cells, damaging adjacent neurons [98, 116, 117]. Moreover, cobalt oxide nanoparticles induce oxidative stress by generating secondary ROS, elevating the hypoxia-induced factor alpha 1 expression. It also leads to mitochondrial damage, reduces ATP content, morphological damage, and neuronal cell damage, and induces autophagy [118]. Cobalt oxide nanoparticles reduce brain weight and Nissl bodies leading to neural damage. It also upregulates phosphorylated and unphosphorylated tau protein levels in the cortex and hippocampus. Besides, it increases inflammatory cytokines like NLRP3 and IL-1β expression, microglial marker Iba-1 expression, and NADPH oxidase in the mice hippocampus. All these findings depict the neurotoxicity effect of cobalt nanoparticles which can cause AD progression [119].
Mechanism of nickel oxide nanoparticles on AD
The neurotoxic effect of nickel nanoparticles has a wide concern as the brain is considered a targeted site of nickel oxide nanoparticles. Nickel oxide nanoparticles are reported to possess a higher solubility profile than fine particles and release more Ni2 + per weight than fine particles; hence, their toxicity is greater than fine particles. These nickel oxide nanoparticles were found to be absorbed in the gastrointestinal tract and distributed in the various organs, including the liver and lungs, via the circulatory system and induce oxidative stress and inflammatory responses leading to toxic effects [120, 121]. Some studies reported that nickel oxide nanoparticles are associated with neurotoxicity, including neurodegenerative diseases and neuroinflammation, by reaching the brain [122]. Inhalation of nickel oxide nanoparticles can also cause a rapid increase of Aβ40. [123].
Mechanism of tin oxide nanoparticles
Jaragh-Alhadad and Falahati studied the mechanism of neurotoxicity of tin oxide nanoparticles in AD. Tin oxide nanoparticles induced the formation of protofibrils or oligomers of Aβ proteins and fastened the fibrillation of Aβ42 peptides. The tin oxide nanoparticles also reduced cell viability due to the activation of caspase-3 [124].
BIOCOMPATIBILITY OF METAL NANOPARTICLES FOR THE TREATMENT OF AD
Any drug delivery system targeting biological systems must be properly studied and characterized. The biological environment in which the delivery system is targeted must ensure its compatibility [125]. The nanoparticles administered as smart drug delivery systems, including liposomes or vesicular systems, nanoparticles (polymeric, solid-lipid, or metal), and dendrimers, should be evaluated for biocompatibility for the delivery of a drug safely with minimum cytotoxicity. The multi-billion-dollar pharmaceutical industries are conducting biocompatibility studies of nano-delivery systems from laboratories to establish their efficacy and safety in treating different diseases [126]. The metal nanoparticles interact with biological systems by their surface properties than their mass [127–131]. Biophysico-chemical interactions of the metal nanoparticles occur at their surface in contact with the biological membranes and cells [132]. The biocompatibility of metal nanoparticles depends on different intrinsic properties od particles, types of formulations, biological targets, dose, route of administration, and the methodology adopted to determine the toxicity [133]. Surface modification of the metal nanoparticles may enhance their biological efficacy. Still, the nanoparticles without any surface modification are mostly taken up by phagocytes, leading to undesired interactions of the metal nanoparticles with the immune system. The interaction of the metal nanoparticles with immune systems decreases their bioavailability and increases their toxicity [134].
Scientifically, the biocompatibility of a delivery system ensures that the interaction of the nanoparticles with the biological system does not induce undesirable toxins or exhibit thrombogenic, immunogenic, or carcinogenic effects. When metal nanoparticles are introduced into the body, the immune system considers them as foreign bodies eliciting undesirable immune responses [135]. Immunostimulation occurs by the molecular structures, the pattern of folding motifs, purity, and stability of the packages of pharmaceuticals [136]. Similarly, immunosuppression is the downregulation or inhibition of the immune system activation. Generally, immunosuppression is disagreeable, but it is found to be useful in the treatment of autoimmune diseases. Immunosuppression depends on different factors like dose, route, and time of administration, site of activity, and the mechanism of action [137].
The administration of metal nanoparticles leads to distribution into different organs, but the distribution and accumulation of metal ions cause neurotoxicity [138]. The major mechanism of neurotoxicity is also associated with oxidative stress. Hence, some reports reported that using antioxidants along with metal nanoparticles reduced neurotoxicity by reducing ROS [20]. Selenium, gold, ruthenium, and zinc oxide nanoparticles are reported to be safer and more effective in treating AD [90, 139]. Green synthesized metal nanoparticles are reportedly less neurotoxic and have high antioxidant effects in AD treatment [140, 141].
CLINICAL TRIALS OF ALZHEIMER’S DISEASE USING METAL NANOPARTICLES
Though many advances in research have been made in diagnosing and treating AD, no successful results have been obtained. The first four acetylcholinesterase inhibitors approved were Tacrine (1995), donepezil (1996), rivastigmine (1997), and galantamine (2001), and the fifth one was memantine (2003), an NMDA receptor antagonist. Tacrine was withdrawn from the market in 2013, whereas the combination of donepezil and memantine was approved in 2014 by the FDA. These drugs are moderately effective, and since then, many drugs have undergone clinical trials, but all have failed [142]. The list includes Gantenerumab (2010, phase I), Semagacestat (2011, phase III), ABT-354 (2013, phase I), Avagacestat (2013, phase II), Bapineurumab (2013, phase III), Solanezumab and Verubecestat (2017, phase III), Intepirdine (2018, phase III), Atabecestat (2018, phase I), Lanabecestat (2018, phase II), Aducanumab (2019, phase III), Crenezumab (2019, phase III), and CNP520 (2020, phase II/III) [142]. Later towards the end of 2019, China’s National Medical Products Administration approved an oligosaccharide named oligomannate (GV-971) derived from seaweed-derived in mild-to-moderate AD patients through a single-phase III trial of Chinese patients, and the sponsor now initiated a global phase III trial [143].
The safety and efficacy of metal nanoparticles are still under research up to pre-clinical studies; though some reports showed remarkable efficacy, they are still not approved for clinical studies. The mechanism of action of anti-AD drugs is very limited, and the continuous failure of new medicines and withdrawal of previously approved drugs may be a limitation for the clinical study of metal nanoparticles in AD.
CHALLENGES AND FUTURE PERSPECTIVES
The characterization and quality assessment regarding the safety and efficacy of metal nanoparticles is one of the major challenges in the current scenario. Lack of standardized methodologies for the above limits the application of nanomedicines in different neurodegenerative diseases or malignancy ailments. Hence, a critical role of regulatory agencies is required to develop universal regulatory guidelines for the characterization and evaluation of metal nanoparticles for therapeutic application. Though some nanoparticles are reported to be nontoxic and biocompatible, still the packaging materials may be immunogenic. So proper in-vitro-in-vivo-correlation studies must be conducted to develop nanomedicines. From the metal-involved pathogenesis, the designing of metal-based therapeutics or theranostics requires some potential direction such as 1) the discovery of new targets, 2) the development of stimuli-responsive targeted theranostics, and 3) integrating metal-complexes, metal-chelators, or metal-nanoparticles in pairs or all together. Tau protein and hyperphosphorylated tau proteins are considered the most toxic substances in the pathogenesis of AD; hence metal ions from the metal nanoparticles may influence the phosphorylation of tau and reduce ROS production. Stimuli-responsive metal nanoparticles exhibit target-specific delivery and release of the drug. Hence, pH, temperature, and light-sensitive drug delivery systems should be developed to get the maximum therapeutic efficacy and minimum side-effects of the metal nanoparticles. Combination of metal nanoparticles with metal chelators or metal complexes may elicit a multifunctional therapeutic effect synergistically.
CONCLUSION
The present review illustrated the neuroprotective and neurotoxic effects of different metal nanoparticles. The activity of magnetic and noble metal particles in the central nervous system or the brain determines its protective or toxic effect. The metal nanoparticles affect different cellular and molecular processes to elicit the neuroprotective or neurotoxic effect. Two primary mechanisms involved in neurodegeneration are the imbalance in the oxidative enzymes leading to oxidative stress and neuroinflammation. Again, the physical properties of metal nanoparticles determine their fate, whether they will exert neuroprotective or neurotoxic activity. Hence, more research studies are required to establish the relationship between the physical properties affecting neuroprotection or neurotoxicity.
ACKNOWLEDGMENTS
The authors are thankful to Siksha ‘O’ Anusandhan Deemed to be University for providing the facilities for the review work.
FUNDING
The authors have no funding to report.
CONFLICT OF INTEREST
The authors have no conflict of interest to report.
REFERENCES
[1] | Brookmeyer R , Gray S , Kawas C ((1998) ) Projections of Alzheimer’s disease in the United States and the public health impact of delaying disease onset. Am J Public Health 88: , 1337–1342. |
[2] | Ayaz M , Junaid M , Ullah F , Subhan F , Sadiq A , Ali G , Ovais M , Shahid M , Ahmad A , Wadood A , El-Shazly M , Ahmad N , Ahmad S ((2017) ) Anti-Alzheimer’s studies on β-sitosterol isolated from polygonum hydropiper L. . Front Pharmacol 8: , 697. |
[3] | Weiner MW , Veitch DP , Aisen PS , Beckett LA , Cairns NJ , Green RC , Harvey D , Jack CR , Jagust W , Liu E , Morris JC , Petersen RC , Saykin AJ , Schmidt ME , Shaw L , Siuciak JA , Soares H , Toga AW , Trojanowski JQ ; Alzheimer’s Disease Neuroimaging Initiative ((2013) ) The Alzheimer’s disease neuroimaging initiative: A review of papers published since its inception. Alzheimers Dement 9: , e111–e194. |
[4] | Fonseca-Santos B , Gremião MP , Chorilli M ((2015) ) Nanotechnology-based drug delivery systems for the treatment of Alzheimer’s disease. Int J Nanomedicine 10: , 4981–5003. |
[5] | Ayaz M , Ovais M , Ahmad I , Sadiq A , Khalil AT , Ullah F ((2020) ) Biosynthesized metal nanoparticles as potential Alzheimer’s disease therapeutics. In Metal Nanoparticles for Drug Delivery and Diagnostic Applications, Shah MR, Imran M, Ullah S, eds. Elsevier, pp. 31–42 . |
[6] | Cummings JL , Vinters HV , Cole GM , Khachaturian ZS ((1998) ) Alzheimer’s disease: Etiologies, pathophysiology, cognitive reserve, and treatment opportunities. Neurology 51: , S2–S17. |
[7] | Castellani RJ , Rolston RK , Smith MA ((2010) ) Alzheimer disease. Dis Mon 56: , 484–546. |
[8] | Ballatore C , Lee VM , Trojanowski JQ ((2007) ) Tau-mediated neurodegeneration in Alzheimer’s disease and related disorders. Nat Rev Neurosci 8: , 663–672. |
[9] | Ryu JK , McLarnon JG ((2009) ) A leaky blood– brain barrier, fibrinogen infiltration and microglial reactivity in inflamed Alzheimer’s disease brain. J Cell Mol Med 13: , 2911–2925. |
[10] | Siddiqi KS , Husen A , Sohrab SS , Yassin MO ((2018) ) Recent status of nanomaterial fabrication and their potential applications in neurological disease management. Nanoscale Res Lett 13: , 231. |
[11] | Bhowmick TK , Suresh AK , Kane SG , Joshi AC , Bellare JR ((2009) ) Indian traditional medicine jasada bhasma and other zinc-containing nanoparticles alleviate reactive oxygen species– mediated cell damage in saccharomyces cerevisiae. Int J Green Nanotechnol Biomed 1: , B69–B89. |
[12] | Behera A , Padhi S ((2020) ) Passive and active targeting strategies for the delivery of the camptothecin anticancer drug: A review. Environ Chem Lett 18: , 1557–1567. |
[13] | Gupta J , Fatima MT , Islam Z , Khan RH , Uversky VN , Salahuddin P ((2019) ) Nanoparticle formulations in the diagnosis and therapy of Alzheimer’s disease. Int J Biol Macromol 130: , 515–526. |
[14] | Pradhan SP , Padhi S , Dash M , Heena , Mittu B , Behera A ((2022) ) Carotenoids. In Nutraceuticals and Health Care, Kaur J, Nayik GA, eds. Academic Press pp. 135–157. |
[15] | Behera A , Mittu B , Padhi S , Patra N , Singh J ((2020) ) Bimetallic nanoparticles: Green synthesis, applications, and future perspectives. In Multifunctional Hybrid Nanomaterials for Sustainable Agri-Food and Ecosystems, Abd-Elsalam KA, ed. Elsevier, pp. 639–682. |
[16] | Ealia SAM , Saravanakumar M ((2017) ) Paper presented at the IOP conference series: Materials science and engineering. A review on the classification, characterisation, synthesis of nanoparticles and their application. |
[17] | Singh R , Lillard JW Jr ((2009) ) Nanoparticle-based targeted drug delivery. Exp Mol Pathol 86: , 215–223. |
[18] | Blanco E , Shen H , Ferrari M ((2015) ) Principles of nanoparticle design for overcoming biological barriers to drug delivery. Nat Biotechnol 33: , 941–951. |
[19] | Pradhan SP , Sahoo S , Behera A , Sahoo R , Sahu PK ((2022) ) Memory amelioration by hesperidin conjugated gold nanoparticles in diabetes induced cognitive impaired rats. J Drug Deliv Sci Technol 69: , 103145. |
[20] | Pradhan SP , Tejaswani P , Sa N , Behera A , Sahoo RK , Sahu PK ((2023) ) Mechanistic study of gold nanoparticles of vildagliptin and vitamin E in diabetic cognitive impairment. J Drug Deliv Sci Technol 84: , 104508. |
[21] | Sayre LM , Perry G , Harris PL , Liu Y , Schubert KA , Smith MA ((2000) ) In situ oxidative catalysis by neurofibrillary tangles and senile plaques in Alzheimer’s disease: A central role for bound transition metals. J Neurochem 74: , 270–279. |
[22] | Bonda DJ , Lee HG , Blair JA , Zhu X , Perry G , Smith MA ((2011) ) Role of metal dyshomeostasis in Alzheimer’s disease. Metallomics 3: , 267–270. |
[23] | Torres AK , Jara C , Park-Kang HS , Polanco CM , Tapia D , Alarcón F , de la Peña A , Llanquinao J , Vargas-Mardones G , Indo JA , Inestrosa NC , Tapia-Rojas C ((2021) ) Synaptic mitochondria: An earlytarget of amyloid-β and tau in Alzheimer’s disease. JAlzheimers Dis 84: , 1391–1414. |
[24] | Liu PP , Xie Y , Meng XY , Kang JS ((2019) ) History and progress of hypotheses and clinical trials for Alzheimer’s disease. Signal Transduct Target Ther 4: , 29. |
[25] | Du X , Wang X , Geng M ((2018) ) Alzheimer’s disease hypothesis and related therapies. Transl Neurodegener 7: , 2. |
[26] | Dubey SK , Lakshmi K , Krishna KV , Agrawal M , Singhvi G , Saha RN , Saraf S , Saraf S , Shukla R , Alexander A ((2020) ) Insulin mediated novel therapies for the treatment of Alzheimer’s disease. Life Sci 249: , 117540. |
[27] | Wischik CM , Harrington CR , Storey JM ((2014) ) Tau-aggregation inhibitor therapy for Alzheimer’s disease. Biochem Pharmacol 88: , 529–539. |
[28] | Jouanne M , Rault S , Voisin-Chiret AS ((2017) ) Tau protein aggregation in Alzheimer’s disease: An attractive target for the development of novel therapeutic agents. Eur J Med Chem 139: , 153–167. |
[29] | Congdon EE , Sigurdsson EM ((2018) ) Tau-targeting therapies for Alzheimer disease. Nat Rev Neurol 14: , 399–415. |
[30] | Rajmohan R , Reddy PH ((2017) ) Amyloid-beta and phosphorylated tau accumulations cause abnormalities at synapses of Alzheimer’s disease neurons. J Alzheimers Dis 57: , 975–999. |
[31] | Chen XQ , Mobley WC ((2019) ) Exploring the pathogenesis of Alzheimer disease in basal forebrain cholinergic neurons: Converging insights from alternative hypotheses. Front Neurosci 13: , 446. |
[32] | Ferreira-Vieira TH , Guimaraes IM , Silva FR , Ribeiro FM ((2016) ) Alzheimer’s disease: Targeting the cholinergic system. Curr Neuropharmacol 14: , 101–115. |
[33] | Esposito Z , Belli L , Toniolo S , Sancesario G , Bianconi C , Martorana A ((2013) ) Amyloid β, glutamate, excitotoxicity in Alzheimer’s disease: Are we on the right track? CNS Neurosci Ther 19: , 549–555. |
[34] | Carvajal FJ , Mattison HA , Cerpa W ((2016) ) Role of NMDA receptor-mediated glutamatergic signaling in chronic and acute neuropathologies. Neural Plast 2016: , 2701526. |
[35] | Singh A , Kukreti R , Saso L , Kukreti S ((2019) ) Oxidative stress: A key modulator in neurodegenerative diseases. Molecules 24: , 1583. |
[36] | Cui H , Kong Y , Zhang H ((2012) ) Oxidative stress, mitochondrial dysfunction, and aging. J Signal Transduct 2012: , 646354. |
[37] | Zorov DB , Juhaszova M , Sollott SJ ((2014) ) Mitochondrial reactive oxygen species (ROS) and ROS-induced ROS release. Physiol Rev 94: , 909–950. |
[38] | Butterfield DA , Halliwell B ((2019) ) Oxidative stress, dysfunctional glucose metabolism and Alzheimer disease. Nat Rev Neurosci 20: , 148–160. |
[39] | Area-Gomez E , de Groof A , Bonilla E , Montesinos J , Tanji K , Boldogh I , Pon L , Schon EA ((2018) ) A key role for MAM in mediating mitochondrial dysfunction in Alzheimer disease. Cell Death Dis 9: , 335. |
[40] | Wang J , Markesbery WR , Lovell MA ((2006) ) Increased oxidative damage in nuclear and mitochondrial DNA in mild cognitive impairment. J Neurochem 96: , 825–832. |
[41] | Guo C , Sun L , Chen X , Zhang D ((2013) ) Oxidative stress, mitochondrial damage and neurodegenerative diseases. Neural Regen Res 8: , 2003–2014. |
[42] | Kwon HS , Koh SH ((2020) ) Neuroinflammation in neurodegenerative disorders: The roles of microglia and astrocytes. Transl Neurodegener 9: , 42. |
[43] | Mandrekar-Colucci S , Landreth GE ((2010) ) Microglia and inflammation in Alzheimer’s disease. CNS Neurol Disord Drug Targets 9: , 156–167. |
[44] | Kinney JW , Bemiller SM , Murtishaw AS , Leisgang AM , Salazar AM , Lamb BT ((2018) ) Inflammation as a central mechanism in Alzheimer’s disease. Alzheimers Dement 4: , 575–590. |
[45] | Bernaus A , Blanco S , Sevilla A ((2020) ) Glia crosstalk in neuroinflammatory diseases. Front Cell Neurosci 14: , 209. |
[46] | Zhang W , Jiao B , Xiao T , Liu X , Liao X , Xiao X , Guo L , Yuan Z , Yan X , Tang B , Shen L ((2020) ) Association of rare variants in neurodegenerative genes with familial Alzheimer’s disease. Ann Clin Transl Neurol 7: , 1985–1995. |
[47] | Han MR , Schellenberg GD , Wang LS ; Alzheimer’s Disease Neuroimaging Initiative ((2010) ) Genome-wide association reveals genetic effects on human Aβ42 and τ protein levels in cerebrospinal fluids: A case control study. BMC Neurol 10: , 90. |
[48] | Xu J , Patassini S , Begley P , Church S , Waldvogel HJ , Faull RL , Unwin RD , Cooper GJ ((2020) ) Cerebral deficiency of vitamin B5 (d-pantothenic acid; pantothenate) as a potentially-reversible cause of neurodegeneration and dementia in sporadic Alzheimer’s disease. Biochem Biophys Res Commun 527: , 676–681. |
[49] | Chang XL , Tan MS , Tan L , Yu JT ((2016) ) The role of TDP-43 in Alzheimer’s disease. Mol Neurobiol 53: , 3349–3359. |
[50] | Tremblay C , St-Amour I , Schneider J , Bennett DA , Calon F ((2011) ) Accumulation of transactive response DNA binding protein 43 in mild cognitive impairment and Alzheimer disease. J Neuropathol Exp Neurol 70: , 788–798. |
[51] | Medina DX , Orr ME , Oddo S ((2014) ) Accumulation of C-terminal fragments of transactive response DNA-binding protein 43 leads to synaptic loss and cognitive deficits in human TDP-43 transgenic mice. Neurobiol Aging 35: , 79–87. |
[52] | Chung YH , Lin CW , Huang HY , Chen SL , Huang HJ , Sun YC , Lee GC , Lee-Chen GJ , Chang YC , Hsieh-Li HM ((2020) ) Targeting inflammation, PHA-767491 shows a broad spectrum in protein aggregation diseases. J Mol Neurosci 70: , 1140–1152. |
[53] | Cascella R , Fani G , Bigi A , Chiti F , Cecchi C ((2019) ) Partial failure of proteostasis systems counteracting TDP-43 aggregates in neurodegenerative diseases. Int J Mol Sci 20: , 3685. |
[54] | Fang YS , Tsai KJ , Chang YJ , Kao P , Woods R , Kuo PH , Wu CC , Liao JY , Chou SC , Lin V , Jin LW , Yuan HS , Cheng IH , Tu PH , Chen YR ((2014) ) Full-length TDP-43 forms toxic amyloid oligomers that are present in frontotemporal lobar dementia-TDP patients. Nat Commun 5: , 4824. |
[55] | Calderón-Garcidueñas L , González-Maciel A , Reynoso-Robles R , Hammond J , Kulesza R , Lachmann I , Torres-Jardón R , Mukherjee PS , Maher BA ((2020) ) Quadrupleabnormal protein aggregates in brainstem pathology and exogenousmetal-rich magnetic nanoparticles (and engineered Ti-rich nanorods). The substantia nigrae is a very early target in young urbanites andthe gastrointestinal tract a key brainstem portal. Environ Res 191: , 110139. |
[56] | Lambert MP , Barlow AK , Chromy BA , Edwards C , Freed R , Liosatos M , Morgan TE , Rozovsky I , Trommer B , Viola KL , Wals P , Zhang C , Finch CE , Krafft GA , Klein WL ((1998) ) Diffusible, nonfibrillar ligands derived from Aβ1–42 are potent central nervous system neurotoxins. Proc Natl Acad Sci U S A 95: , 6448–6453. |
[57] | Shirazi SK , Wood JG ((1993) ) The protein tyrosine kinase, Fyn, in Alzheimer’s disease pathology. Neuroreport 4: , 435–437. |
[58] | Chun H , Lee CJ ((2018) ) Reactive astrocytes in Alzheimer’s disease: A double-edged sword. Neurosci Res 126: , 44–52. |
[59] | Padhi S , Behera A ((2021) ) Advanced drug delivery systems in the treatment of ovarian cancer. In Advanced Drug Delivery Systems in the Management of Cancer, Dua K, Mehta M, de Jesus Andreoli Pinto T, Pont LG, Williams KA, Rathbone MJ, eds. Academic Press, pp. 127–139. |
[60] | Nazıroğlu M , Muhamad S , Pecze L ((2017) ) Nanoparticles aspotential clinical therapeutic agents in Alzheimer’s disease: Focuson selenium nanoparticles. Expert Rev Clin Pharmacol 10: , 773–782. |
[61] | Wang X , Wang X , Guo Z ((2018) ) Metal-involved theranostics: An emerging strategy for fighting Alzheimer’s disease. Coord Chem Rev 362: , 72–84. |
[62] | Larsen A , Kolind K , Pedersen DS , Doering P , Pedersen MØ , Danscher G , Penkowa M , Stoltenberg M ((2008) ) Gold ions bio-released from metallic gold particles reduce inflammation and apoptosis and increase the regenerative responses in focal brain injury. Histochem Cell Biol 130: , 681–692. |
[63] | Heneka MT , Carson MJ , El Khoury J , Landreth GE , Brosseron F , Feinstein DL , Jacobs AH , Wyss-Coray T , Vitorica J , Ransohoff RM , Herrup K , Frautschy SA , Finsen B , Brown GC , Verkhratsky A , Yamanaka K , Koistinaho J , Latz E , Halle A , Petzold GC , Town T , Morgan D , Shinohara ML , Perry VH , Holmes C , Bazan NG , Brooks DJ , Hunot S , Joseph B , Deigendesch N , Garaschuk O , Boddeke E , Dinarello CA , Breitner JC , Cole GM , Golenbock DT , Kummer MP ((2015) ) Neuroinflammation in Alzheimer’s disease. Lancet Neurol 14: , 388–405. |
[64] | Deng Z , Jin J , Wang Z , Wang Y , Gao Q , Zhao J ((2017) ) The metal nanoparticle-induced inflammatory response is regulated by SIRT1 through NF-κB deacetylation in aseptic loosening. Int J Nanomedicine 12: , 3617–3636. |
[65] | Walczak-Nowicka ŁJ , Herbet M ((2021) ) Acetylcholinesterase inhibitors in the treatment of neurode-generative diseases and therole of acetylcholinesterase in their pathogenesis. Int J MolSci 22: , 9290. |
[66] | Patra N , Kar D , Pal A , Behera A ((2018) ) Antibacterial, anticancer, anti-diabetic and catalytic activity of bio-conjugated metal nanoparticles. Adv Nat Sci Nanosci Nanotechnol 9: , 035001. |
[67] | Sivanesan S , Rajeshkumar S ((2019) ) Gold nanoparticles in diagnosis and treatment of Alzheimer’s disease. Nanobiotechnology in Neurodegenerative Diseases, Rai M, Yada A, eds. Springer Cham, pp. 289–306. |
[68] | Han S , Kim K , Kim H , Kwon J , Lee YH , Lee CK , Song Y , Lee SJ , Ha N , Kim K ((2008) ) Auranofin inhibits overproduction of pro-inflammatory cytokines, cyclooxygenase expression and PGE 2 production in macrophages. Arch Pharm Res 31: , 67–74. |
[69] | Barathmanikanth S , Kalishwaralal K , Sriram M , Pandian SR , Youn HS , Eom S , Gurunathan S ((2010) ) Anti-oxidant effect of gold nanoparticles restrains hyperglycemic conditions in diabetic mice. J Nanobiotechnology 8: , 16. |
[70] | Saini P , Saha SK , Roy P , Chowdhury P , Sinha Babu SP ((2016) ) Evidence of reactive oxygen species (ROS) mediated apoptosis in Setaria cervi induced by green silver nanoparticles from Acacia auriculiformis at a very low dose. Exp Parasitol 160: , 39–48. |
[71] | Yin T , Yang L , Liu Y , Zhou X , Sun J , Liu J ((2015) ) Sialic acid (SA)-modified selenium nanoparticles coated with a high blood– brain barrier permeability peptide-B6 peptide for potential use in Alzheimer’s disease. Acta Biomater 25: , 172–183. |
[72] | Salnikov V , Lukyanenko Y , Frederick C , Lederer W , Lukyanenko V ((2007) ) Probing the outer mitochondrial membrane in cardiac mitochondria with nanoparticles. Biophys J 92: , 1058–1071. |
[73] | Busquets MA , Sabaté R , Estelrich J ((2014) ) Potential applicationsof magnetic particles to detect and treat Alzheimer’s disease. Nanoscale Res Lett 9: , 538. |
[74] | Dowding JM , Song W , Bossy K , Karakoti A , Kumar A , Kim A , Bossy B , Seal S , Ellisman MH , Perkins G , Self WT , Bossy-Wetzel E ((2014) ) Cerium oxide nanoparticles protect against aβ-induced mitochondrial fragmentation and neuronal cell death. Cell Death Differ 21: , 1622–1632. |
[75] | Guan Y , Gao N , Ren J , Qu X ((2016) ) Rationally designed CeNP@ MnMoS4core-shell nanoparticles for modulating multiple facets ofAlzheimer’s disease. Chemistry 22: , 14523–14526. |
[76] | Chaudhary S , Umar A , Mehta S ((2014) ) Surface functionalized selenium nanoparticles for biomedical applications. J Biomed Nanotechnol 10: , 3004–3042. |
[77] | Rajeshkumar S , Ganesh L , Santhoshkumar J ((2019) ) Selenium nanoparticles as therapeutic agents in neurodegenerative diseases. In Nanobiotechnology in Neurodegenerative Diseases, Rai M, Yada A, eds. Springer Cham, pp. 209–224. |
[78] | Amani H , Habibey R , Shokri F , Hajmiresmail SJ , Akhavan O , Mashaghi A , Pazoki-Toroudi H ((2019) ) Selenium nanoparticles for targeted stroke therapy through modulation of inflammatory and metabolic signaling. Sci Rep 9: , 6044. |
[79] | Khurana A , Tekula S , Saifi MA , Venkatesh P , Godugu C ((2019) ) Therapeutic applications of selenium nanoparticles. Biomed Pharmacother 111: , 802–812. |
[80] | Gao F , Zhao J , Liu P , Ji D , Zhang L , Zhang M , Li Y , Xiao Y ((2020) ) Preparation and in vitro evaluation of multi-target-directed selenium-chondroitin sulfate nanoparticles in protecting against the Alzheimer’s disease. Int J Biol Macromol 142: , 265–276. |
[81] | Roohani N , Hurrell R , Kelishadi R , Schulin R ((2013) ) Zinc and its importance for human health: An integrative review. J Res Med Sci 18: , 144–157. |
[82] | Blakemore LJ , Trombley PQ ((2017) ) Zinc as a neuromodulator in the central nervous system with a focus on the olfactory bulb. Front Cell Neurosci 11: , 297. |
[83] | Watt NT , Whitehouse IJ , Hooper NM ((2011) ) The role of zinc in Alzheimer’s disease. Int J Alzheimers Dis 2011: , 971921. |
[84] | Stelmashook E , Isaev N , Genrikhs E , Amelkina G , Khaspekov L , Skrebitsky V , Illarioshkin S ((2014) ) Role of zinc and copper ions in the pathogenetic mechanisms of Alzheimer’s and Parkinson’s diseases. Biochemistry (Moscow) 79: , 391–396. |
[85] | Vilella A , Belletti D , Sauer AK , Hagmeyer S , Sarowar T , Masoni M , Stasiak N , Mulvihill JJE , Ruozi B , Forni F , Vandelli MA , Tosi G , Zoli M , Grabrucker AM ((2018) ) Reduced plaque size and inflammation in the APP23 mouse model for Alzheimer’s disease after chronic application of polymeric nanoparticles for CNS targeted zinc delivery. J Trace Elem Med Biol 49: , 210–221. |
[86] | El-Hawwary SS , Abd Almaksoud HM , Saber FR , Elimam H , Sayed AM , El Raey MA , Abdelmohsen UR ((2021) ) Green-synthesized zinc oxide nanoparticles, anti-Alzheimer potential and the metabolic profiling of Sabal blackburniana grown in Egypt supported by molecular modelling. RSC Adv 11: , 18009–18025. |
[87] | Zhao Y , Luo Y , Yang X , Yang Y , Song Q ((2017) ) Tunable preparation of ruthenium nanoparticles with superior size-dependent catalytic hydrogenation properties. J Hazard Mater 332: , 124–131. |
[88] | Zhou H , Gong Y , Liu Y , Huang A , Zhu X , Liu J , Yuan G , Zhang L , Wei JA , Liu J ((2020) ) Intelligently thermoresponsive flower-like hollow nano-ruthenium system for sustained release of nerve growth factor to inhibit hyperphosphorylation of tau and neuronal damage for the treatment of Alzheimer’s disease. Biomaterials 237: , 119822. |
[89] | Yuan X , Jia Z , Li J , Liu Y , Huang Y , Gong Y , Guo X , Chen X , Cen J , Liu J ((2021) ) A diselenide bond-containing ROS-responsive ruthenium nanoplatform delivers nerve growth factor for Alzheimer’s disease management by repairing and promoting neuron regeneration. J Mater Chem B 9: , 7835–7847. |
[90] | Yang L , Chen Q , Liu Y , Zhang J , Sun D , Zhou Y , Liu J ((2014) ) Se/Ru nanoparticles as inhibitors of metal-induced Aβ aggregation in Alzheimer’s disease. J Mater Chem B 2: , 1977–1987. |
[91] | Ansciaux E , Burtea C , Laurent S , Crombez D , Nonclercq D , Vander Elst L , Muller RN ((2015) ) In vitro and characterization of several functionalized ultrasmall particles of iron oxide, vectorized against amyloid plaques and potentially able to cross the blood– brain barrier: Toward earlier diagnosis of Alzheimer’s disease by molecular imaging. Contrast Media Mol Imaging 10: , 211–224. |
[92] | Glat M , Skaat H , Menkes-Caspi N , Margel S , Stern EA ((2013) ) Age-dependent effects of microglial inhibition on Alzheimer’s disease neuropathology using bioactive-conjugated iron oxide nanoparticles. J Nanobiotechnology 11: , 32. |
[93] | Katebi S , Esmaeili A , Ghaedi K , Zarrabi A ((2019) ) Super paramagnetic iron oxide nanoparticles combined with NGF and quercetin promote neuronal branching morphogenesis of PC12 cells. Int J Nanomedicine 14: , 2157–2169. |
[94] | Liu XG , Zhang L , Lu S , Liu DQ , Huang YR , Zhu J , Zhou WW , Yu XL , Liu RT ((2020) ) Superparamagnetic iron oxide nanoparticles conjugated with Aβ oligomer-specific SCFV antibody and class a scavenger receptor activator show therapeutic potentials for Alzheimer’s disease. J Nanobiotechnology 18: , 160. |
[95] | Sanati M , Aminyavari S , Khodagholi F , Hajipour MJ , Sadeghi P , Noruzi M , Moshtagh A , Behmadi H , Sharifzadeh M ((2021) ) PEGylated superparamagnetic iron oxide nanoparticles (SPIONs) ameliorate learning and memory deficit in a rat model of Alzheimer’s disease: Potential participation of STIMs. Neurotoxicology 85: , 145–159. |
[96] | Medina C , Santos-Martinez M , Radomski A , Corrigan O , Radomski M ((2007) ) Nanoparticles: Pharmacological and toxicological significance. Br J Pharmacol 150: , 552–558. |
[97] | Guo K , Pan Q , Wang L , Fang S ((2002) ) Nano-scale copper-coated graphite as anode material for lithium-ion batteries. J Appl Electrochem 32: , 679–685. |
[98] | Zheng F , Luo Z , Zheng C , Li J , Zeng J , Yang H , Chen J , Jin Y , Aschner M , Wu S , Zhang Q , Li H ((2019) ) Comparison of the neurotoxicity associated with cobalt nanoparticles and cobalt chloride in Wistar rats. Toxicol Appl Pharmacol 369: , 90–99. |
[99] | Li J , Wang J , Wang YL , Luo Z , Zheng C , Yu G , Wu S , Zheng F , Li H ((2021) ) NOX2 activation contributes to cobalt nanoparticles-induced inflammatory responses and tau phosphorylation in mice and microglia. Ecotoxicol Environ Saf 225: , 112725. |
[100] | Song B , Zhang Y , Liu J , Feng X , Zhou T , Shao L ((2016) ) Is neurotoxicity of metallic nanoparticles the cascades of oxidative stress? Nanoscale Res Lett 11: , 291. |
[101] | Steckiewicz KP , Barcinska E , Malankowska A , Zauszkiewicz– Pawlak A , Nowaczyk G , Zaleska-Medynska A , Inkielewicz-Stepniak I ((2019) ) Impact of gold nanoparticles shape on their cytotoxicity against human osteoblast and osteosarcoma in in vitro model. Evaluation of the safety of use and anti-cancer potential. J Mater Sci Mater Med 30: , 22. |
[102] | Liu Z , Li W , Wang F , Sun C , Wang L , Wang J , Sun F ((2012) ) Enhancement of lipopolysaccharide-induced nitric oxide and interleukin-6 production by PEGylated gold nanoparticles in RAW264.7 cells. Nanoscale 4: , 7135–7142. |
[103] | Carro CE , Pilozzi AR , Huang X ((2019) ) Nanoneurotoxicity and potential nanotheranostics for Alzheimer’s disease. EC Pharmacol Toxicol 7: , 1–7. |
[104] | Fahmy HM , Aly EM , Mohamed FF , Noor NA , Elsayed AA ((2020) ) Neurotoxicity of green-synthesized magnetic iron oxide nanoparticles in different brain areas of Wistar rats. Neurotoxicology 77: , 80–93. |
[105] | Fernández-Bertólez N , Costa C , Brandão F , Kiliç G , Teixeira JP , Pásaro E , Laffon B , Valdiglesias V ((2018) ) Neurotoxicity assessment of oleic acid-coated iron oxide nanoparticles in SH-SY5Y cells. Toxicology 406: , 81–91. |
[106] | Wu J , Ding T , Sun J ((2013) ) Neurotoxic potential of iron oxide nanoparticles in the rat brain striatum and hippocampus. Neurotoxicology 34: , 243–253. |
[107] | Schrand AM , Rahman MF , Hussain SM , Schlager JJ , Smith DA , Syed AF ((2010) ) Metal-based nanoparticles and their toxicity assessment. Wiley Interdiscip Rev Nanomed Nanobiotechnol 2: , 544–568. |
[108] | Song B , Liu J , Feng X , Wei L , Shao L ((2015) ) A review on potential neurotoxicity of titanium dioxide nanoparticles. Nanoscale Res Lett 10: , 1042. |
[109] | Czajka M , Sawicki K , Sikorska K , Popek S , Kruszewski M , Kapka-Skrzypczak L ((2015) ) Toxicity of titanium dioxide nanoparticles in central nervous system. Toxicol In Vitro 29: , 1042–1052. |
[110] | Gheshlaghi ZN , Riazi GH , Ahmadian S , Ghafari M , Mahinpour R ((2008) ) Toxicity and interaction of titanium dioxide nanoparticles with microtubule protein. Acta Biochim Biophys Sin (Shanghai) 40: , 777–782. |
[111] | Poulsen HE , Specht E , Broedbaek K , Henriksen T , Ellervik C , Mandrup-Poulsen T , Tonnesen M , Nielsen PE , Andersen HU , Weimann A ((2012) ) RNA modifications by oxidation: A novel disease mechanism? Free Radic Biol Med 52: , 1353–1361. |
[112] | Perreault F , Pedroso Melegari S , Henning da Costa C , de Oliveira Franco Rossetto AL , Popovic R , Gerson Matias W ((2012) ) Genotoxic effects of copper oxide nanoparticles in neuro 2a cell cultures. Sci Total Environ 441: , 117–124. |
[113] | Li F , Zhou X , Zhu J , Ma J , Huang X , Wong ST ((2007) ) High content image analysis for human H4 neuroglioma cells exposed to CuO nanoparticles. BMC Biotechnol 7: , 66. |
[114] | Liu Z , Liu S , Ren G , Zhang T , Yang Z ((2011) ) Nano-CuO inhibited voltage-gated sodium current of hippocampal CA1 neurons via reactive oxygen species but independent from G-proteins pathway. J Appl Toxicol 31: , 439–445. |
[115] | An L , Liu S , Yang Z , Zhang T ((2012) ) Cognitive impairment in rats induced by nano-CuO and its possible mechanisms. Toxicol Lett 213: , 220–227. |
[116] | Karovic O , Tonazzini I , Rebola N , Edström E , Lövdahl C , Fredholm BB , Daré E ((2007) ) Toxic effects of cobalt in primary cultures of mouse astrocytes: Similarities with hypoxia and role of HIF-1alpha. Biochem Pharmacol 73: , 694–708. |
[117] | Cendrowska-Pinkosz M , Krauze M , Juśkiewicz J , Ognik K ((2021) ) The effect of the use of copper carbonate and copper nanoparticles inthe diet of rats on the level of β-amyloid and acetylcholinesterase in selected organs. J Trace Elem Med Biol 67: , 126777. |
[118] | Zheng F , Luo Z , Lin X , Wang W , Aschner M , Cai P , Wang YL , Shao W , Yu G , Guo Z , Wu S , Li H ((2021) ) Intercellular transfer of mitochondria via tunneling nanotubes protects against cobalt nanoparticle-induced neurotoxicity and mitochondrial damage. Nanotoxicology 24: , 1358–1379. |
[119] | Deng S , Yan X , Xiong P , Li G , Ku T , Liu N , Liao C , Jiang G ((2021) ) Nanoscale cobalt-based metal-organic framework impairs learning and memory ability without noticeable general toxicity: First evidence. Sci Total Environ 771: , 145063. |
[120] | Siddiqui MA , Ahamed M , Ahmad J , Khan MM , Musarrat J , Al-Khedhairy AA , Alrokayan SA ((2012) ) Nickel oxide nanoparticles induce cytotoxicity, oxidative stress and apoptosis in cultured human cells that is abrogated by the dietary antioxidant curcumin. Food Chem Toxicol 50: , 641–647. |
[121] | Ahamed M , Ali D , Alhadlaq HA , Akhtar MJ ((2013) ) Nickel oxide nanoparticles exert cytotoxicity via oxidative stress and induce apoptotic response in human liver cells (HepG2). Chemosphere 93: , 2514–2522. |
[122] | Abudayyak M , Guzel E , Özhan G ((2017) ) Nickel oxide nanoparticles are highly toxic to SH-SY5Y neuronal cells. Neurochem Int 108: , 7–14. |
[123] | Rivas-Arancibia S , Guevara-Guzmán R , López-Vidal Y , Rodríguez-Martínez E , Zanardo-Gomes M , Angoa-Pérez M , Raisman-Vozari R ((2010) ) Oxidative stress caused by ozone exposure induces loss of brain repair in the hippocampus of adult rats. Toxicol Sci 113: , 187–197. |
[124] | Jaragh-Alhadad LA , Falahati M ((2022) ) Tin oxide nanoparticles trigger the formation of amyloid β oligomers/protofibrils and underlying neurotoxicity as a marker of Alzheimer’s diseases. Int J Biol Macromol 204: , 154–160. |
[125] | Williams DF ((2008) ) On the mechanisms of biocompatibility. Biomaterials 29: , 2941–2953. |
[126] | Kohane DS , Langer R ((2010) ) Biocompatibility and drug delivery systems. Chem Sci 1: , 441–446. |
[127] | Brown DM , Wilson MR , MacNee W , Stone V , Donaldson K ((2001) ) Size-dependent proinflammatory effects of ultrafine polystyrene particles: A role for surface area and oxidative stress in the enhanced activity of ultrafines. Toxicol Appl Pharmacol 175: , 191–199. |
[128] | Donaldson K , Brown D , Clouter A , Duffin R , MacNee W , Renwick L , Tran L , Stone V ((2002) ) The pulmonary toxicology of ultrafine particles. J Aerosol Med 15: , 213–220. |
[129] | Donaldson K , Li X , MacNee W ((1998) ) Ultrafine (nanometre) particle mediated lung injury. J Aerosol Sci 29: , 553–560. |
[130] | Oberdörster G , Ferin J , Gelein R , Soderholm SC , Finkelstein J ((1992) ) Role of the alveolar macrophage in lung injury: Studies with ultrafine particles. Environ Health Perspect 97: , 193–199. |
[131] | Tran C , Buchanan D , Cullen R , Searl A , Jones A , Donaldson K ((2000) ) Inhalation of poorly soluble particles. II. Influence of particle surface area on inflammation and clearance. Inhal Toxicol 12: , 1113–1126. |
[132] | Nel AE , Mädler L , Velegol D , Xia T , Hoek EM , Somasundaran P , Klaessig F , Castranova V , Thompson M ((2009) ) Understanding biophysicochemical interactions at the nano– bio interface. Nat Mater 8: , 543–557. |
[133] | Kus-Liśkiewicz M , Fickers P , Ben Tahar I ((2021) ) Bio compatibility and cytotoxicity of gold nanoparticles: Recent advances in methodologies and regulations. Int J Mol Sci 22: , 10952. |
[134] | Naahidi S , Jafari M , Edalat F , Raymond K , Khademhosseini A , Chen P ((2013) ) Biocompatibility of engineered nanoparticles for drug delivery. J Control Release 166: , 182–194. |
[135] | Descotes J ((2004) ) Importance of immunotoxicity in safety assessment: A medical toxicologist’s perspective. Toxicol Lett 149: , 103–108. |
[136] | Chamberlain P , Mire-Sluis A ((2003) ) An overview of scientific and regulatory issues for the immunogenicity of biological products. Dev Biol (Basel) 112: , 3–11. |
[137] | Říhová B ((2007) ) Biocompatibility and immunocompatibility of water-soluble polymers based on HPMA. Compos B Eng 38: , 386–397. |
[138] | Feng X , Chen A , Zhang Y , Wang J , Shao L , Wei L ((2015) ) Central nervous system toxicity of metallic nanoparticles. Int J Nanomedicine 10: , 4321–4340. |
[139] | Suganthy N , Sri Ramkumar V , Pugazhendhi A , Benelli G , Archunan G ((2018) ) Biogenic synthesis of gold nanoparticles from terminalia arjuna bark extract: Assessment of safety aspects and neuroprotective potential via antioxidant, anticholinesterase, and antiamyloidogenic effects. Environ Sci Pollut Res Int 25: , 10418–10433. |
[140] | Ovais M , Zia N , Ahmad I , Khalil AT , Raza A , Ayaz M , Sadiq A , Ullah F , Shinwari ZK ((2018) ) Phyto-therapeutic and nanomedicinal approaches to cure Alzheimer’s disease: Present status and future opportunities. Front Aging Neurosci 10: , 284. |
[141] | Hassan D , Khalil AT , Saleem J , Diallo A , Khamlich S , Shinwari ZK , Maaza M ((2018) ) Biosynthesis of pure hematite phase magnetic iron oxide nanoparticles using floral extracts of Callistemon viminalis (bottlebrush): Their physical properties and novel biological applications. Artif Cells Nanomed Biotechnol 46: , 693–707. |
[142] | Srivastava S , Ahmad R , Khare SK ((2021) ) Alzheimer’s disease and its treatment by different approaches: A review. Eur J Med Chem 216: , 113320. |
[143] | Green valley announces NMPA approval of oligomannate for mild to moderate Alzheimer’s disease. Green Valley announces NMPA approval of oligomannate for mild to moderate Alzheimer’s disease- press release-Green Valley, https://www.greenvalleypharma.com/En/Index/pageView/catid/48/id/28.html. |
[144] | Zhang X , Li Y , Hu Y ((2020) ) Green synthesis of silver nanoparticles and their preventive effect in deficits in recognition and spatial memory in sporadic Alzheimer’s rat model. Colloids Surf A Physicochem Eng Asp 605: , 125288. |
[145] | Youssif KA , Haggag EG , Elshamy AM , Rabeh MA , Gabr NM , Seleem A , Salem MA , Hussein AS , Krischke M , Mueller MJ , Abdelmohsen UR ((2019) ) Anti-Alzheimer potential, metabolomic profiling and molecular docking of green synthesized silver nanoparticles of Lampranthus coccineus and Malephora lutea aqueous extracts. PLoS One 14: , e0223781. |
[146] | Ceylan R , Demirbas A , Ocsoy I , Aktumsek A ((2021) ) Green synthesis of silver nanoparticles using aqueous extracts of three sideritis species from Turkey and evaluations bioactivity potentials. Sustain Chem Pharm 21: , 100426. |
[147] | Dos Santos Tramontin N , da Silva S , Arruda R , Ugioni KS , Canteiro PB , de Bem Silveira G , Mendes C , Silveira PCL , Muller A ((2020) ) Gold nanoparticles treatment reverses brain damage in Alzheimer’s disease model. Mol Neurobiol 57: , 926–936. |
[148] | Suganthy N , Sri Ramkumar V , Pugazhendhi A , Benelli G , Archunan G ((2018) ) Biogenic synthesis of gold nanoparticles from terminalia arjuna bark extract: Assessment of safety aspects and neuroprotective potential via antioxidant, anticholinesterase, and antiamyloidogenic effects. Environ Sci Pollut Res 25: , 10418–10433. |
[149] | Anand BG , Wu Q , Karthivashan G , Shejale KP , Amidian S , Wille H , Kar S ((2021) ) Mimosine functionalized gold nanoparticles (mimo-AuNPs) suppress β-amyloid aggregation and neuronal toxicity. Bioact Mater, 6: , 4491–4505. |
[150] | Hou K , Zhao J , Wang H , Li B , Li K , Shi X , Wan K , Ai J , Lv J , Wang D , Huang Q , Wang H , Cao Q , Liu S , Tang Z ((2020) ) Chiral gold nanoparticles enantioselectively rescue memory deficits in a mouse model of Alzheimer’s disease. Nat Commun 11: , 4790. |
[151] | Huo X , Zhang Y , Jin X , Li Y , Zhang L ((2019) ) A novel synthesis of selenium nanoparticles encapsulated PLGA nanospheres with curcumin molecules for the inhibition of amyloid β aggregation in Alzheimer’s disease. J Photochem Photobiol B 190: , 98–102. |
[152] | Yang L , Sun J , Xie W , Liu Y , Liu J ((2017) ) Dual-functional selenium nanoparticles bind to and inhibit amyloid β fiber formation in Alzheimer’s disease. J Mater Chem B 5: , 5954–5967. |
[153] | Li C , Wang N , Zheng G , Yang L ((2021) ) Oral administration of resveratrol-selenium-peptide nanocomposites alleviates Alzheimer’s disease-like pathogenesis by inhibiting aβ aggregation and regulating gut microbiota. ACS Appl Mater Interfaces 13: , 46406–46420. |
[154] | Abozaid OA , Sallam MW , El-Sonbaty S , Aziza S , Emad B , Ahmed ES ((2022) ) Resveratrol-selenium nanoparticles alleviate neuroinflammation and neurotoxicity in a rat model of Alzheimer’s disease by regulating SIRT1/miRNA-134/GSK3β expression. Biol Trace Elem Res 200: , 5104–5114. |
[155] | Gholamigeravand B , Shahidi S , Afshar S , Gholipour P , Samzadeh-Kermani A , Amiri K , Majidi M , Abbasalipourkabir R , Arabestani MR , Soleimani Asl S ((2021) ) Synergistic effects of adipose-derived mesenchymal stem cells and selenium nanoparticles on streptozotocin-induced memory impairment in the rat. Life Sci 272: , 119246. |
[156] | Zhang J , Zhou X , Yu Q , Yang L , Sun D , Zhou Y , Liu J ((2014) ) Epigallocatechin-3-gallate (EGCG)-stabilized selenium nanoparticles coated with TET-1 peptide to reduce amyloid-β aggregation and cytotoxicity. ACS Appl Mater Interfaces 6: , 8475–8487. |
[157] | Sundararajan V , Venkatasubbu GD , Sheik Mohideen S ((2021) ) Investigation of therapeutic potential of cerium oxide nanoparticles in Alzheimer’s disease using transgenic drosophila. 3 Biotech 11: , 159. |