Resting-State Functional Connectivity Change in Frontoparietal and Default Mode Networks After Acute Exercise in Youth
Abstract
BACKGROUND:
A single bout of aerobic exercise can provide acute benefits to cognition and emotion in children. Yet, little is known about how acute exercise may impact children’s underlying brain networks’ resting-state functional connectivity (rsFC).
OBJECTIVE:
Using a data-driven multivariate pattern analysis, we investigated the effects of a single dose of exercise on acute rsFC changes in 9-to-13-year-olds.
METHODS:
On separate days in a crossover design, participants (N = 21) completed 20-mins of acute treadmill walking at 65–75% heart rate maximum (exercise condition) and seated reading (control condition), with pre- and post-fMRI scans. Multivariate pattern analysis was used to investigate rsFC change between conditions.
RESULTS:
Three clusters in the left lateral prefrontal cortex (lPFC) of the frontoparietal network (FPN) had significantly different rsFC after the exercise condition compared to the control condition. Post-hoc analyses revealed that from before to after acute exercise, activity of these FPN clusters became more correlated with bilateral lPFC and the left basal ganglia. Additionally, the left lPFC became more anti-correlated with the precuneus of the default mode network (DMN). An opposite pattern was observed from before to after seated reading.
CONCLUSIONS:
The findings suggest that a single dose of exercise increases connectivity within the FPN, FPN integration with subcortical regions involved in movement and cognition, and segregation of FPN and DMN. Such patterns, often associated with healthier cognitive and emotional control, may underlie the transient mental benefits observed following acute exercise in youth.
INTRODUCTION
Existing research has established that physical activity promotes healthy overall development. Alarmingly, this comes against a backdrop where inadequate physical activity accounts for $117 billion in annual U.S. health care costs. [1, 2]. Notably, reviews and meta-analyses have identified a positive relationship between physical activity and cognitive health in childhood and adolescence [3–11]. Acute exercise, defined as a single instance of structured physical activity, also described as a “dose” of exercise [12], is believed to create a temporary state of enhanced mood [13, 14] and cognitive performance [15, 16]. Benefits are primarily studied for cardiorespiratory activities at moderate-to-vigorous intensities (65% +heart rate maximum) and are thought to have the strongest effects 10–20 minutes after exercise, beginning to wane thereafter [16]. Prior research has provided valuable insights for public health recommendations [11], illustrating that understanding the cognitive effects of acute exercise can offer novel information supporting youth development. However, an understanding of the relationship between acute exercise and neurobiological changes remains incomplete [17].
Noninvasive neuroimaging presents tools to dissect this neurobiological relationship in human development in vivo. Among neuroimaging features, event-related brain potentials (ERP), evident in task-based electroencephalography (EEG) recordings, are the most well-documented in connection with acute bouts of exercise in children and adolescents [18]. For example, Hillman et al. [19] studied acute exercise, specifically aerobic treadmill walking, on cognitive control in 9–10-year-olds. They observed improved response accuracy and an amplified P300-ERP component, suggesting enhanced attentional control processing [20]. This effect of acute exercise on neuroelectric brain function has been well replicated in multiple studies [4, 12, 21] and across populations [22–24], and are generally of a larger effect size than observed benefits to cognitive performance [25, 26]. Such research establishes that acute exercise induces a temporary state that affords greater allocation of neural resources supporting attentional control [27].
While task-based measures are important for understanding specific cognitive processes (e.g., cognitive control), resting-state measures may better describe more general characteristics of an exercise-induced state. Consensus exists regarding a transient rise in EEG alpha activity (7.5–12.5 Hz) following an exercise bout, a phenomenon initially observed by Beaussart et al. [28], which has been interpreted to reflect post-exercise improvements in information processing [29]. In addition to alpha, resting-state EEG changes have been observed in theta (3.5–7.5 Hz), beta (12.5–35 Hz) and gamma (>35 Hz) ranges [25], but there is no strong consensus regarding the functional relevance of differing frequency ranges in the exercise literature [30]. In essence, while EEG studies confirm that acute exercise enhances cognitive performance and associated neural processes, EEG resting-state descriptions, to date, have not offered a clear picture of brain-wide changes induced by exercise.
Functional magnetic resonance imaging (fMRI) complements EEG by providing regional specificity in studying the resting-state brain. Functional connectivity, defined as temporal dependencies of the blood oxygen-level dependent (BOLD) signal, reflects coherence between disparate brain regions and networks of regions that support cognition [31]. Classic work by Fox and Rachle [32] showed that the BOLD signal reflects local changes in blood flow relating neuronal aerobic glycolysis, indirectly indexing information processing [33]. Resting-state functional connectivity (rsFC) therefore reflects neural information processing via intrinsic network interconnectedness, where within-network rsFC combined with low out-network rsFC ensures optimal functioning by maintaining specialization of processes and reducing potential interference [34]. Of note, the frontoparietal network (FPN), including posterior parietal (PPC) and lateral prefrontal cortices (lPFC), is critical for cognitive control processes [35] like those shown to be improved by acute exercise [16]. Prior fMRI research establishes that with greater rsFC within the FPN, individuals tend to perform better on tasks that require goal-directed control of thoughts and actions [36].
Network rsFC changes in association with other functional networks may also be important for understanding cognitive performance. For example, another functional network of regions termed the default mode network (DMN) includes posterior cingulate, precuneus, and medial prefrontal cortices (mPFC). The DMN is most active during a resting-state and is broadly associated with introspective processes such as daydreaming, self-referential thought, and recalling past experiences [37]. FPN-DMN between-network associations change throughout childhood, moving from a relative lack of correlation (or even a positive correlation) in childhood to a robust anti-correlation between these networks in adulthood [38, 39]. Increased FPN-DMN anti-correlations have been linked with heightened cognitive performance, particularly in tasks demanding complex working memory [40–42]. Disruptions to FPN-DMN anti-correlations have also been linked to various mental health conditions in development [43], such as in a sample of 7-year-olds, where lacking age-appropriate FPN-DMN anti-correlation was predictive of attention deficit symptoms four years later [44].
Critically, FPN-DMN rsFC anti-correlations can be beneficially modulated through behavioral interventions. For example, Bauer et al. [45] studied mindfulness training in 10-11-year-olds. They found FPN-DMN anticorrelations were maintained for those who practiced mindfulness over an 8-week intervention but were reduced for those assigned to a coding-training control group. Further, FPN-DMN rsFC change in that sample was related to an attentional control task, such that increased anti-correlation was related to increased cognitive performance. These findings supported the hypothesis that mindfulness training can promote healthy network rsFC and cognition in youth. Prior reviews on neural plasticity have noted similarities between meditation and physical activity [46]. Like exercise, the neurobiological mechanisms of mediation are less widely studied than cognitive effects, where a reoccurring practice of meditation is known to benefit to cognitive control [47]. Moreover, even a single session of meditation has been shown to acutely benefit task performance [48]. It is therefore possible that meditation-like FPN-DMN anti-correlations could be observed following acute exercise in youth.
Evidence for the influence of acute exercise on rsFC is currently primarily derived from adult samples [49–53]. For example, studying healthy younger and older adults, Weng et al. [53] found that compared to a passive cycling control condition, moderate-intensity cycling increased rsFC within the affective-reward network, suggestive of acute exercise effects on mood, and decreased rsFC between regions of the FPN and a network correlated with hippocampus activity. Additional research by Schmitt et al. [51] in athletic adult males found that compared to pre-high-intensity cycling, post-high-intensity cycling showed increased rsFC within the affective-reward network, supported by significant corresponding exercise-induced positive affect. They further observed decreased rsFC within the somatomotor and dorsal attention networks, primarily affecting different regions of the post-central gyrus, consistent with effects of muscular fatigue. Finally, compared to pre-low-intensity cycling, post-low-intensity cycling showed increased rsFC within the FPN [51]. The significance of acute exercise-induced rsFC change is further underscored by Voss et al. [52], who demonstrated that immediate rsFC responses to an exercise bout, especially within the DMN, predicted long-term rsFC adaptations in response to a sustained exercise intervention among healthy older adults. Previous research hypothesizes that rsFC changes are mediated by norepinephrine circuits originating in the locus coeruleus [53], consistent with the catecholamine hypothesis for cognitive performance benefits of acute exercise [54]. In sum, acute exercise affects cortical networks implicated in mood, cognitive performance, and motor control by increasing within-network rsFC in service of network coherence underlying temporary alterations in cognitive state. However, regions of rsFC change are widespread throughout the brain and peak loci are inconsistent across acute exercise studies, potentially due to substantial variation regarding seed regions used for network analyses.
Our current research builds on previous studies by assessing the impact of acute exercise on rsFC in youth with multivariate pattern analysis, a data-driven approach that allows simultaneous analysis across the whole brain rsFC connectome [55, 56]. This innovative technique, not yet commonly used in the exercise neuroimaging literature, offers a nuanced understanding of rsFC changes associated with an acute exercise intervention. Specifically, multivariate pattern analysis estimates the entire connectome at the resolution of individual voxels without reliance on seed regions, constructing a model that best explains the heterogeneity in rsFC related to experimental properties [55]. This data-driven approach allows whole-brain analysis by reducing dataset dimensionality, focusing only on the most robust sources of voxel-wise rsFC variance. Given prior exercise rsFC research, we hypothesized that an acute cardiorespiratory exercise intervention at a moderate-to-vigorous intensity would increase rsFC within networks important for mood and cognitive performance (e.g., affective-reward network, DMN, FPN), as well as modulation of within-network rsFC among motor control regions (e.g., somatomotor network). Further, we hypothesized that acute exercise would decrease between-network rsFC, specifically reflected in increased FPN-DMN anti-correlations, reminiscent of mindfulness training and a hallmark of typical healthy development. Thus, we broadly hypothesized that a single dose of acute exercise would modulate cortical circuits implicated in mood and cognitive performance.
MATERIALS AND METHODS
Participants
This study recruited 24 healthy youth following their participation in a prior study ([57]; ClinicalTrials.gov NCT03592238). After voluntary withdrawal of one participant and fMRI data exclusion from two participants due to excessive motion, the final sample consisted of 21 youth, 9 to 13 years-old. (see Table 1; additional supporting information may be found in the Supplemental Material).
Table 1
Participant characteristics
N | 21 |
Age, years (mean (sd)) | 11.4 (1.08) |
Sex, female (n (%)) | 9 (43%) |
Aerobic fitness, relative VO2max (mean (sd)) | 44.3 (5.69) |
Socioeconomic status, mother’s years in school (mean (sd)) | 16.7 (3.25) |
Race/ethnicity (n (%)) | |
White or Caucasian | 8 (38.1%) |
Hispanic or Latino | 5 (23.1%) |
Black or African | 4 (19%) |
Asian | 2 (9.5%) |
Mixed or other | 2 (9.5%) |
In prior recruitment [57], participant exclusion criteria included non-consent from guardian or non-assent from participant, presence of contraindicators for physical exercise as assessed by the Physical Activity Readiness Questionnaire [58], an IQ < 85 on the Kaufman Brief Intelligence Test-2 [59], reported diagnosis of physical or cognitive disability, use of psychiatric medication, vision not correctable to the minimum 20/20 standard, and not fluent in English. For this study, participants with known claustrophobia or dental work potentially causing MRI artifacts were also excluded during the recruitment process. For this study, all participants provided addition written assent, and their legal guardians provided written informed consent in accordance with the Institutional Review Board of Northeastern University.
Experimental procedure
In prior research with these participants [57], cardiorespiratory fitness was assessed as maximum volume of oxygen (VO2max) utilized per minute per kilogram of body mass, collected with a modified Balke protocol consistent with the American College of Sports Medicine guidelines [60]. Specifically, participants warmed up by walking at a comfortable pace for 2.5 minutes, before being brought to a constant running speed. The speed was determined by an experimenter based on participant running preference and technique. After 3 minutes, intensity increased by graded incline of 2.5%, which reoccurred every 2 minutes until volitional fatigue.
In this study, we employed a single-blinded, within-participant crossover pretest-posttest comparison [12]. Participants visited the lab on two occasions, at least one week apart (Mean = 19±14.8 days), after prior data collection [57]. During both visits, participants completed resting-state fMRI scans before and after (pre and post) an intervention of two possible conditions (acute exercise and seated reading control). The interventions lasted 20 minutes each, were counterbalanced across laboratory visits, and blinded from participants until after pre-intervention fMRI. To minimize residual physiological effects of acute exercise, participants waited at least 10 minutes after interventions to return to within 10% of resting heart rate, which was included in both conditions. The only physiological and cognitive change measures that we collected during the interventions were heart rate percentage (HR%), used by the experimenter to ensure target intensity during exercise, feeling scale (FS) [61], and rating of perceived exertion (RPE) [62]. The FS and RPE respectively measure affective pleasantness and perception of expended effort, and are among the most common measures in the acute exercise literature [63]. Participants rated FS and RPE every 2 minutes while the experimenter recorded HR% every minute throughout both interventions (see qualitative analysis in supplement).
Acute exercise condition
The acute exercise condition included physical activity at a sustained heart rate of 65–75% of participants’ own maximal heart rate achieved during cardiorespiratory fitness testing. Specifically, participants engaged in 20 minutes of aerobic walking on a motor-driven treadmill, including a 1-minute warmup. Most of the time (i.e., 19 minutes) was spent exercising with HR% at target intensity. An experimenter monitored participants and after warm-up, adjusted the treadmill speed and incline to achieve target walking intensity, recording heart rate every minute. This exercise stimulus is consistent with the American College of Sports Medicine [60] guidelines for improving aerobic capacity. The acute exercise condition occurred first for a random selection of 10 of 21 participants. Participants began post-intervention resting-state fMRI 14.1±3.0 minutes after acute exercise; HR% was within 10% of pre-intervention for all participants.
Seated reading control condition
The seated reading control condition consisted of sedentary cognitive engagement at a sustained resting heart rate. Specifically, participants engaged in 20 minutes of reading in a seated position, including a 1-minute period to select reading material from a collection provided by the experimenter. Most of the time (i.e., 19 minutes) was spent reading age-appropriate non-fiction while seated. An experimenter monitored participants throughout the intervention, recording HR% every minute. This control stimulus is consistent with the plurality of control conditions used in exercise research [12]. The control condition occurred first for a random selection of 11 of 21 participants in the final sample. Participants began post-intervention resting-state fMRI approximately 13.9±2.9 minutes after seated reading.
MRI acquisition
MRI data were collected before and after each intervention condition on a 3T Siemens MAGNETOM Prisma whole-body MRI scanner with a 64-channel head coil (Siemens Healthcare, Erlangen, Germany). Each imaging session, we acquired two spin-echo images with opposite anterior/posterior (AP and PA) phase encoding direction to generate a field map and one 8-minute resting-state scan with posterior-to-anterior phase encoding direction using a T2*-weighted EPI sequence (TR = 800 ms, TE = 37 ms, flip angle = 52°, matrix size = 104×104, 2 mm isotropic, multiband acceleration factor = 8). During the resting-state scan, participants were instructed to relax and fixate on a white crosshair on a black screen. We also acquired a 0.8 isotropic T1-weighted MPRAGE with Volumetric Navigators [64] for prospective motion correction (TR = 2500 ms, TI = 1000 ms, flip angle = 8°, matrix size = 30×320; see additional acquisition details in supplement).
MRI preprocessing and denoising
Data processing and analysis were performed using the CONN-toolbox v.21a [65] relying on SPM12 (Wellcome Department of Imaging Neuroscience, UCL, London, UK) in MATLAB 2020b (The MathWorks Inc, Natick, MA, USA). First, field inhomogeneity distortions were estimated from field maps data using FSL’s Topup (FMRIB Software Library’s Tool for Unified Platform) and later applied to the resting-state data to perform susceptibility distortion correction [66, 67]. Functional resting-state data were realigned, unwrapped, corrected for distortions [68], and coregistered and resampled to the first scan. Outlier volumes were identified using Artifact Detection Tools [69] and defined as volumes with framewise displacement above 0.5 mm or global BOLD signal change above 3 standard deviations [70]. Functional and structural data were indirectly normalized to MNI152 space [71]. Structural data were segmented into grey matter, white matter (WM), and cerebrospinal fluid (CSF) tissue classes [72]. Lastly, functional data were smoothed with an 8 mm Gaussian kernel.
Functional data were denoised using the CONN default fMRI denoising pipeline [73]. This pipeline comprises three main sequential steps. First, principal components of WM and CSF masks were extracted using a component analysis-based correction (aCompCor) method [74, 75]. Then linear regression was used to remove confounding effects specific to WM (top 5 components) and CSF (top 5 components), six realignment parameters and their first order derivatives (12 components), participant-specific outlier scans (149 components), and condition (pre- and post- exercise and control) including convolved first order derivative (8 components). Finally, band-pass filtering (0.008Hz-0.09 Hz) removed frequencies outside the expected range for neural-derived components in the BOLD signal. A minimum of 5 minutes of scanning time was achieved for all participants in each resting-state data collection session and did not significantly differ across sessions. Quality assurance was conducted in accordance with recent recommendations [76]. Before preprocessing, raw data were examined for acquisition artifacts. After preprocessing, data were inspected for adequate normalization, realignment, and registration. After denoising, data were inspected for denoising success and participants were considered for exclusion. At this stage, two participants were removed from further analysis due to excessive mean motion, constituting less than 10% of the total sample (see supplement). For context, fMRI research in this age group often excludes at least 10% of total participants due to in-scanner motion and other participant-caused artifacts [77].
Statistical analyses
Resting-state data were analyzed via multivariate pattern analysis to investigate within-participant differences following acute exercise, relative to the seated reading control condition, on rsFC patterns across the whole brain. We analyzed 64 within-voxel dimensions and the first factor extracted from 21 participants across four scanning sessions, corresponding to a conservative 20 : 1 participant-factor ratio which is commonly used in multivariate pattern analysis of rsFC [55, 77–81]. Additionally, analysis of the first factor is independently interpretable because this is the principal pattern best characterizing the observed rsFC variance across participants and conditions [55]. We can therefore interpret our results as the most robust within-participant effect that exists in the dataset as a function of intervention condition.
We applied a General Linear Model (GLM) analysis to the data derived from our multivariate pattern analysis. Our GLM was constructed around four conditions (2×2), which combined two intervention conditions (acute exercise or control) and two points in time (pre- and post-intervention). In accordance with prior literature [77], a height-level statistical threshold of p<0.001, false discovery rate (FDR)-corrected cluster threshold of p<0.05, and cluster size k≥50 were used to adetermine significant clusters [65]. This second-level analysis modeled the first multivariate factor, a statistical construct representing whole-brain variance in rsFC across all participants and conditions, as predicted by intervention condition. We therefore used a priori confound selection [82], including six covariates of no interest in the GLM: age, sex, socioeconomic status (mother’s education), aerobic fitness (VO2-max), the intervention condition on the first lab visit (i.e., counterbalancing), and mean framewise displacement. Prior research with a known effect size found a similar whole-brain multivariate procedure to boast the greatest power of all tested alternative [83]. We evaluated using a t(14) statistic ((exercise-post > exercise-pre)> (control-post > control-pre)), which allowed us to examine within-participant changes in whole-brain rsFC associated with intervention condition. To determine functional network membership, statistically significant clusters were related to whole-brain mean rsFC and to an influential functional parcellation [84]. Additionally, sensitivity analyses evaluated both the number of multivariate factors and a priori covariate selections (see supplement).
Clusters identified in the multivariate pattern analysis GLM were then used as seeds in a post-hoc seed-to-voxel analyses with the same GLM parameters (exercise-post > exercise-pre), (control-post > control-pre). This was done because multivariate pattern analysis GLM can describe the location of brain regions that differ in rsFC but cannot inform as to where these connection differences occur. Because post-hoc significance testing provides only an arbitrary threshold wherein significance should not be independently interpreted, uncorrected cluster threshold of p<0.05 was used with a height-level statistical threshold of p<0.001 and k≥50 to aid interpretation of multivariate pattern analysis in post-hoc analyses [55, 85]. This describes a pattern of regions that exhibited within-participant rsFC change associated with intervention condition.
Pearson-product moment correlations were used to compare (post > pre) changes in rsFC between each cluster to changes in FS and RPE in the respective intention condition (acute exercise, seated reading; FDR-corrected, q = 0.05). Intervention effects on these measures were additionally compared between intervention conditions with paired t-tests to describe the mean differences and Levene’s tests to describe interindividual differences in measure variance.
RESULTS
Multivariate pattern analysis
Statistically significant clusters identified by multivariate pattern analysis of whole brain rsFC for the ((exercise-post > exercise-pre)> (control-post > control-pre)) contrast are displayed in Fig. 1 and tabulated in Table 2.
Fig. 1
Multivariate analysis of resting-state functional connectivity results. Three clusters in the left FPN were associated with pre-to-post connectivity changes between the acute exercise and seated reading control conditions: inferior frontal gyrus pars triangularis (cluster 1), middle frontal gyrus (cluster 2), inferior frontal gyrus pars opercularis (cluster 3). Opaque gold clusters represent significant differences in rsFC change (voxel p<0.001; FDR-corrected cluster threshold p<0.05; k≥50). Shaded red (positive) and blue (negative) clusters show the broader pattern of rsFC change (post > pre; exercise > control) with an uncorrected significance threshold (voxel/cluster p < 0.05).
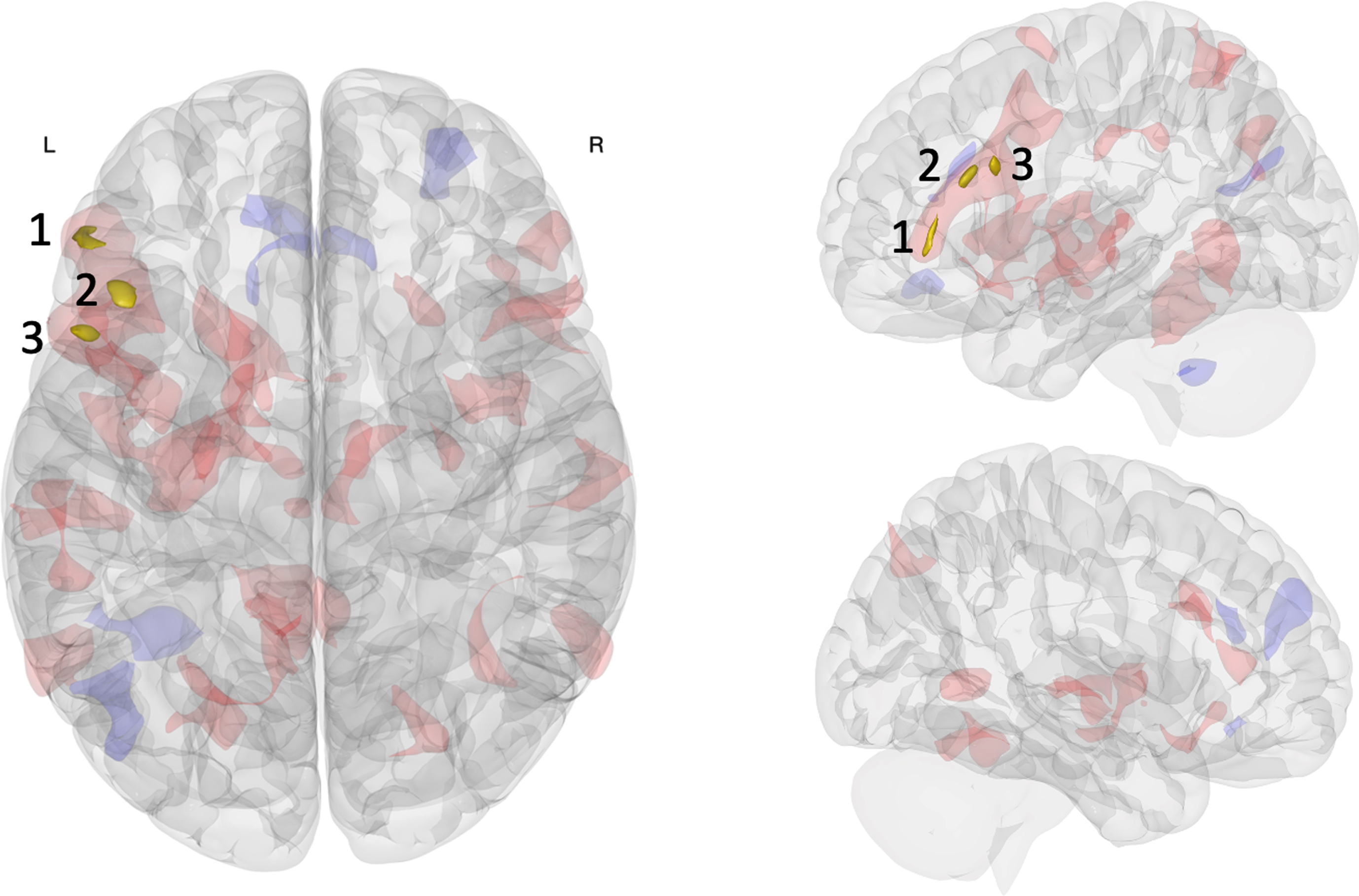
Table 2
Resting-state functional connectivity results
Cluster | Peak coordinate (MNI) | Anatomical region | Network | Cluster size | T Value |
Cluster 1 | -50 + 36 -02 | Left inferior frontal gyrus pars triangularis | Frontoparietal network+Default mode network | 73 | 5.57 |
Cluster 1a | +50 + 36 -04 | Right frontal pole extending into inferior frontal gyrus | Frontoparietal network+Default mode network | 87 | – |
Cluster 1b | -56 + 22 + 14 | Left inferior frontal gyrus pars triangularis extending into pars opercularis | Frontoparietal network+Default mode network | 67 | – |
Cluster 2 | -42 + 24 + 22 | Left middle frontal gyrus extending into inferior frontal gyrus | Frontoparietal network | 68 | 6.56 |
Cluster 2a | -14 + 06 + 06 | Left caudate extending into putamen | – | 85 | – |
Cluster 3 | -50 + 14 + 26 | Left inferior frontal gyrus pars opercularis extending into middle frontal gyrus | Frontoparietal network | 52 | 5.77 |
Cluster 3a | -08 -64 + 20 | Left precuneus | Default mode network | 112 | – |
Cluster 3b | +54 + 28 + 00 | Right inferior frontal gyrus pars triangularis extending into frontal pole | Frontoparietal network+Default mode network | 87 | – |
The within-participant crossover pretest-posttest comparison revealed three clusters differentially affected by the intervention conditions located in the left lPFC along the inferior frontal sulcus: inferior frontal gyrus pars triangularis (cluster 1), middle frontal gyrus (cluster 2), and inferior frontal gyrus pars opercularis (cluster 3). These regions were highly intercorrelated during both pre-intervention resting state scans and predominately included voxels belonging to the FPN [84], additionally including the DMN at the most anterior aspect of cluster 1. Broadly, this pattern of results indicated that the largest rsFC change associated with intervention condition was centered on the ventral left lPFC along the inferior frontal sulcus, primarily within the FPN extending to the anterior FPN-DMN network border. This is especially noteworthy because our multivariate pattern analysis focused on change in the first rsFC factor, which is the principal pattern best characterizing the observed rsFC variance across participants and conditions [55]. When we consider the whole brain, we found the left lPFC of the FPN was most affected by acute exercise relative to seated reading.
Post hoc seed-to-voxel analyses to lateral prefrontal cortex, basal ganglia, and precuneus
Seed-to-voxel results using the multivariate pattern analysis derived clusters are displayed in Fig. 2 and tabulated in Table 2.
Fig. 2
Post-hoc seed to voxel results for clusters within the FPN reveal specific rsFC changes. A) Cluster 1 significantly changed rsFC with the right and left lPFC in the FPN (clusters 1a and 1b, respectively); Cluster 2 with a heteromodal area in left basal ganglia (cluster 2a); Cluster 3 with the precuneus in the DMN (cluster 3a) and the right lPFC in the FPN (cluster 3b). Red (positive) and blue (negative) regions represent rsFC changes associated with acute exercise relative to the seated reading control condition (post > pre; exercise > control). B) Unthresholded results are shown in coronal slices for difference of rsFC change between conditions, represented as Fischer Z-transformed regressor coefficients. B) Bar graphs express mean rsFC at each time point in each intervention (x-axis) represented regressor coefficients (y-axis), with bar color (red positive, blue negative) indicating the direction of pre-to-post intervention rsFC change. Error bars depict 95% confidence intervals for regressor coefficients. Significant main effects were observed for the seated reading control condition between clusters 1 and 1b, and for the acute exercise condition between clusters 2 and 2a and between clusters 3 and 3a.
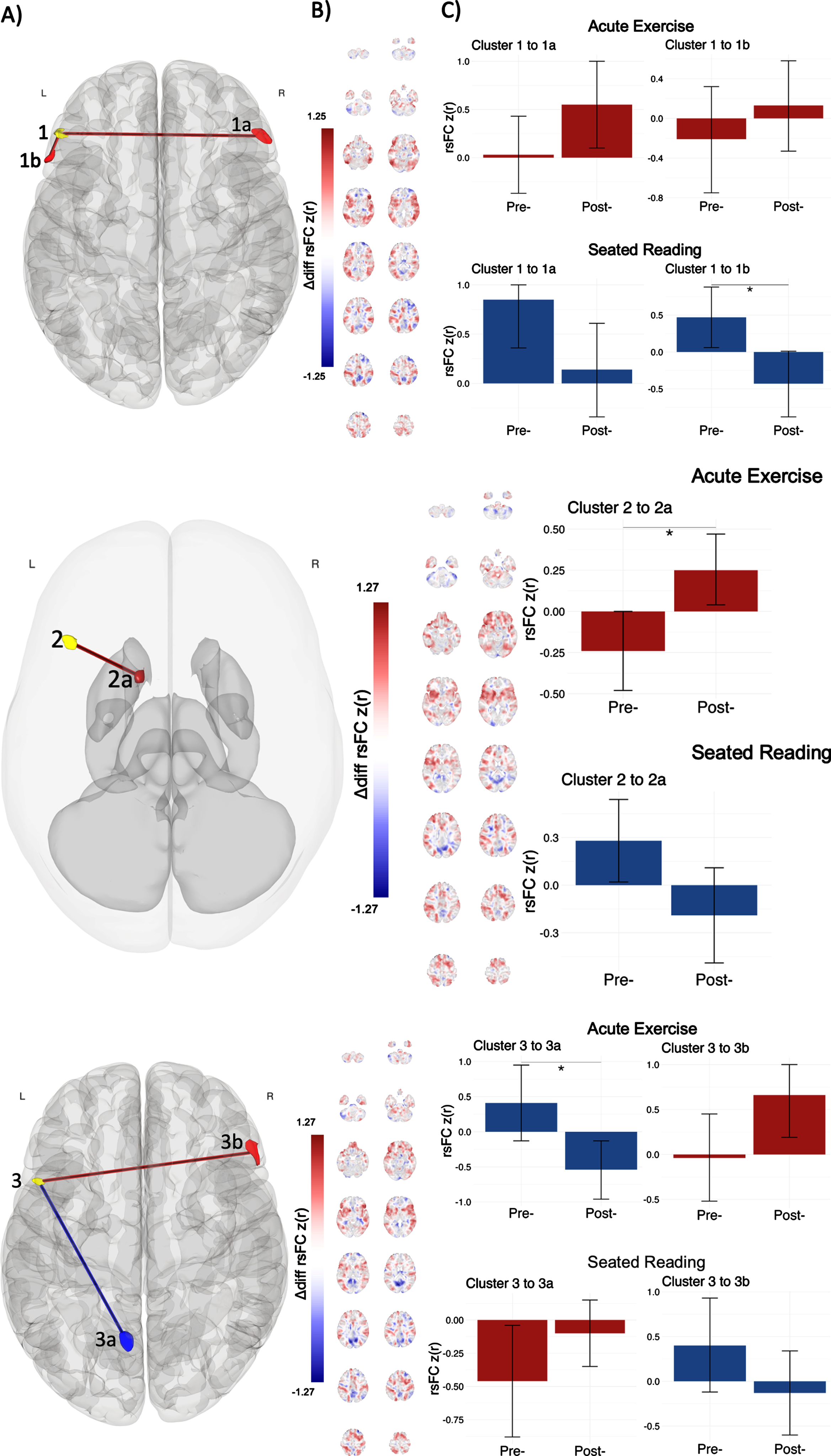
We observed a pattern of increased rsFC associated with the exercise bout in all FPN clusters identified by multivariate pattern analysis. For cluster 1, acute exercise was associated with positive pre-to-post rsFC change to the right lPFC (cluster 1a; pre: r = 0.03, SE = 0.20; post: r = 0.55, SE = 0.23) and to the left lPFC (cluster 1b; pre: r = –0.21, SE = 0.27; post: r = 0.13, SE = 0.23), while seated reading was associated with negative pre-to-post rsFC change to the right lPFC (cluster 1a; pre: r = 0.85, SE = 0.25; post: r = 0.14, SE = 0.24) and to the left lPFC (cluster 1b; pre: r = 0.47, SE = 0.21; post: r = –0.43, SE = 0.23). The rsFC change was especially pronounced for seated reading between clusters 1 and 1b. A similar anterior FPN pattern was observed for cluster 3; acute exercise was associated with positive pre-to-post rsFC change to the right lPFC (cluster 3b; pre: r = –0.04, SE = 0.25; post: r = 0.66, SE = 0.24), while seated reading was associated with negative pre-to-post rsFC change to the right lPFC (cluster 3b; pre: r = 0.40, SE = 0.27; post: r = –0.13, SE = 0.24). Anatomically, the clusters identified with post-hoc seed to voxel analyses sat over the left and right inferior frontal gyrus pars triangularis, extending into the right frontal pole, and left inferior frontal gyrus pars opercularis. Functionally, these clusters displayed positive mean rsFC with seed clusters (
For cluster 2, acute exercise was associated with positive pre-to-post rsFC change in the subcortex (cluster 2a; pre: r = –0.24, SE = 0.12; post: r = 0.25, SE = 0.11), while seated reading was associated with negative pre-to-post rsFC change within the same region (cluster 2a; pre: r = 0.28, SE = 0.13; post: r = –0.19, SE = 0.15); the rsFC change was especially pronounced for acute exercise. Anatomically, this cluster sat over the left caudate of the basal ganglia, extending into the putamen. Functionally, this cluster displayed positive mean rsFC with seed clusters (
We observed a pattern of decreased rsFC associated with exercise in one FPN cluster identified by multivariate pattern analysis. For cluster 3, acute exercise was associated with negative pre-to-post rsFC change within the left precuneus (cluster 3a; pre: r = 0.41, SE = 0.28; post: r = –0.54, SE = 0.21), while seated reading was associated with positive pre-to-post rsFC change within the left precuneus (cluster 3a; pre: r = –0.46, SE = 0.21; post: r = –0.10, SE = 0.13); the rsFC change was especially pronounced for acute exercise. Anatomically, this cluster was entirely consumed by the left precuneus. Functionally, this cluster displayed negative mean rsFC with seed clusters (
Post hoc analyses on rating of perceived exertion and feeling scale changes
We observed no significant correlation between rsFC change and changes in RPE or FS during the acute exercise bout that survived FDR-correction (see supplement).
Paired-sample t-test of RPE indicated that the acute exercise condition was perceived as more exerting than seated reading (acute exercise: M = 4.0, SE = 2.6; seated reading: M = 2.0, SE = 1.9; t(20) = 2.0, p < 0.001). In contrast, FS did not significantly differ between conditions (acute exercise: M = 3.5, SE = 1.6; seated reading: M = 3.9, SE = 1.1; t(20)=0.3, p = 0.08), suggesting similar overall self-reported feeling. Therefore, we found no evidence indicating that exercise induced a state of positive affect in our study. Additionally, Levene’s test showed that both RPE and FS varied more during the acute exercise condition than during seated reading (RPE: F(1, 880)=19.5, p < 0.001; FS: F(1, 880) = 10.4, p < 0.05), indicating greater interindividual variability during acute exercise than during seated reading. This suggests a relatively uniform response between participants due to seated reading relative to acute exercise.
DISCUSSION
The goal of this study was to investigate how a single bout of acute aerobic exercise affects whole-brain rsFC among 9–13-year-old youth. To this end, we employed a single-blinded, within-participant, crossover, pre-test/post-test design, incorporating acute exercise and control conditions that have been commonly used in similar research [12]. Multivariate pattern analysis revealed that the left lPFC exhibited the greatest heterogeneity in rsFC variance across conditions. Post-hoc analyses showed that rsFC in the lPFC changed along three axes: within-FPN, between FPN-subcortex, and between FPN-DMN. Specifically, following exercise, the left lPFC increased rsFC correlation within itself as well as the homologous right lPFC within the FPN; increased rsFC correlation with the left basal ganglia (primarily in the caudate); and increased anti-correlation with the left precuneus of the DMN. Further, the seated reading control condition was associated with an opposite pattern of rsFC change in all identified regions. While significant clusters are small, they represent only the tips of an in underlying pattern identified by our multivariate pattern analysis of rsFC [55]. Overall, this study provides novel evidence of the underlying brain networks impacted by acute exercise research.
Acute exercise and increased within-FPN rsFC
Our data-driven multivariate pattern analysis of rsFC across the whole brain identified that the left lPFC was significantly affected by acute exercise relative to seated reading. Thus, we present novel insight for increased within-FPN rsFC change after acute exercise, consistent with prior acute exercise investigations [49, 51, 53]. Specifically, following acute exercise in 9-13-year-olds, we observed increased rsFC between the left and bilateral lPFC. Bilaterally, the lPFC, located on the side of the frontal lobes from the central sulcus to the anterior pole, encompasses the inferior frontal, the middle frontal, and the superior frontal gyri. The lPFC are best recognized as key regions of the FPN, known for their role in cognitive control [87]. Exercise-induced changes in regions of the FPN are often observed in neurobiological investigations of exercise effects on cognition [3, 6], interpreted to underlie enhanced cognitive performance following acute exercise [16]. For example, in a chronic exercise intervention for 8-9-year-olds, Chaddock- Heyman et al. (2013) found decreased task-based activation in the anterior FPN following a 9-month physical activity intervention, relative to a wait-list control group. This pattern of results indicated more efficient neural processing in the FPN following the physical activity intervention, as this activation pattern was more akin to a group of young adults used as a comparison group in the same study and was accompanied by greater task performance at post-test (Chaddock-Heyman et al., 2013). Accordingly, our findings are consistent with prior research finding exercise-induced changes in the FPN. Therefore, our within-FPN rsFC findings suggest that a single bout of exercise provides greater coherence within a network that underlies cognitive control.
Acute exercise and increased FPN-subcortical rsFC
In addition to increased within-network rsFC change, we present novel evidence for increased FPN integration with the basal ganglia after acute exercise. Specifically, following acute exercise, we observed increased rsFC between the FPN and the functionally heteromodal basal ganglia, primarily in the left caudate. Together, the caudate nucleus and putamen make up the dorsal striatum and play a pivotal role in cognitive and motor functions (Choi et al., 2010) such as coordinating habit and goal-directed actions [88]. Notably, the FPN feeds into the basal ganglia along the dorsal striatum to begin the cortico-basal ganglia-thalamo-cortical loop in service of excitation/inhibition of voluntary movement [89]. Interestingly, [50] observed that rsFC between the thalamus and cortical regions of the somatomotor network (i.e., pre/postcentral gyri, secondary somatosensory area) increased after acute exercise, relative to a passive control condition. While their study involved 20-35-year-olds, we support such thalamo-cortical rsFC change findings with cortico-basal ganglia rsFC change. Therefore, our FPN-subcortical rsFC findings suggest that a single bout of exercise provides greater coherence between a network that underlies cognitive control and a subcortical region involved in integration of thought and motor control. Collectively, our increased rsFC findings may reflect candidate mechanisms that underlie how single doses of exercise provide temporary improvements in cognitive performance.
Acute exercise and increased FPN-DMN rsFC anti-correlations
We present novel evidence for increased FPN-DMN anti-correlations after acute exercise. Specifically, following acute exercise, we observed increased anti-correlation in rsFC between the FPN and the left precuneus, a key region of the DMN. While no prior research has observed FPN-DMN anti-correlations after acute exercise, our findings compliment previous investigations. For example, the robust rise in EEG alpha activity following acute exercise, which is thought to reflect post-exercise improvements in information processing [29], has been suggested by Schneider et al. [90, 91] to arise from both the PFC and precuneus. Additionally, in an 8-month exercise intervention, Krafft et al. (2014) found that overweight 8–11-year-olds in the exercise group exhibited lower rsFC between the FPN and the posterior cingulate extending into the bilateral precuneus, relative to a wait-list control group. It is noteworthy that the posterior cingulate region identified by [92] overlapped with their FPN seed, which was created through independent component analysis before the exercise intervention, suggesting that this region began positively coupled to the FPN before rsFC decreased due to chronic exercise. Converging evidence therefore implicates the precuneus as a brain region with functional relevance to exercise interventions. In contrast, Bauer et al. (2020) focused on the mPFC, not the precuneus, when investigating mindfulness training as a behavioral intervention to modulate cognitive performance and FPN-DMN anti-correlation. This raises the speculative prospect that exercise and mindfulness training target separable aspects (anterior vs. posterior) of FPN-DMN anti-correlation in youth, which could indicate a synergistic effect with combined use.
The precuneus is located in the superior parietal lobule and has a broad functional role, implicated in episodic memory retrieval, visuospatial processing, and self-processing [93]. The precuneus and adjacent posterior cingulate (posterior aspects of the DMN) have even been suggested to be an integration hub for FPN and DMN in the service of affective and emotional control [94]. For example, failure to suppress the posterior cingulate (as well as the left dorsal lPFC) during an emotional control task has been correlated with depression severity in clinically depressed patients [95]. This is reminiscent of how lacking FPN-DMN anti-correlation has been associated with developing mental health symptoms [44]. Importantly, the beneficial effect of chronic exercise on mental health is well known [5, 96], such that regular exercise is thought to mitigate the risk of developing anxiety [97] and depression [98] across age groups, which may be observed in the transient effects of acute exercise on mood [13, 14]. Therefore, our finding that acute exercise increased FPN-DMN anti-correlation in the young brain bridges a conceptual gap between acute exercise effects on neurobiological processes and chronic effects of exercise on mental health. Given that disruptions to FPN-DMN anti-correlation are associated with mental health disorders [43], it is possible that these immediate changes to brain networks induced by acute exercise are precursory steps in a process that, with repetition of chronic exercise, leads to the long-term mental health benefits observed in prior studies [99].
Seated reading control and inverse pattern of rsFC change
Our full model identified three regions within the FPN, in the left lPFC along the inferior frontal sulcus, that exhibited significant heterogeneity in rsFC variance across conditions. While we expected no rsFC change to be specifically associated with the seated reading control condition, post-hoc analyses showed that the control was associated with an opposite pattern of rsFC change as acute exercise. This was especially pronounced in the more anterior cluster that overlapped the FPN and DMN. While there is sparce literature surrounding single sessions of a reading intervention on rsFC change, broader review leads to the intriguing possibility that the seated reading intervention, as a type of sedentary cognitive engagement which is a common control condition in the acute exercise literature [12], was of critical importance to our findings.
Anatomically, this clusters sat over the left inferior frontal gyrus pars triangularis (BA 45), a subdivision of Broca’s area that is known to be important for language processing [100]. For example, task-based fMRI has consistently shown that the left inferior frontal gyrus is active during the processing of written language [101, 102], potentially acting as a locus for cognitive control over semantic information [103]. Therefore, it is possible that our seated reading control condition fatigued associated regions, leading to decreased rsFC after the intervention [104]; a finding requiring further investigation. It is not clear if this pattern of rsFC change is specific to seated reading or is generalizable to other control conditions. In rsFC analysis spanning 9–25-year-olds, Vogel et al. [105] showed that the regions active during reading are broadly distributed across “task-positive” resting-state networks. It is therefore plausible that other forms of sedentary cognitive engagement elicit similar rsFC change in respective cognitive regions. Our post-hoc analyses of cognitive measures reported during both conditions (RPE and FS) characterized the response to seated reading as less variable than to acute exercise, with a significantly lower (although not absent) average perceived exertion, and a nonsignificant difference in overall pleasant feelings. Unfortunately, no significant relationships between changes in these measures and rsFC change in either condition survived correction for multiple comparisons (see supplement). Thus, we were unable to further evaluate the differential rsFC changes that we observed after acute exercise and seated reading. An investigation of acute intervention control conditions could add to this study by better contextualizing the observed inverse pattern of rsFC change between condition.
Limitations
Our primary innovation was the use of multivariate pattern analysis to investigate whole-brain rsFC change associated with acute exercise; however, this method does come with limitations. While multivariate pattern analysis reduces input features that may include spurious correlations [56], all uses of a data-driven approach increase the potential for modeling chance. Indeed, we observed baseline rsFC differences despite randomization and blinding participants to their intervention condition prior to when it began. However, condition order was not related to rsFC change in sensitivity analyses that further displayed a consistent pattern of rsFC change across a priori covariate selection. Sensitivity analyses also revealed our model was robust to the number multivariate factors selected, showing a significant pattern of rsFC change in the left lPFC consistent across analyses. There is the possibility that the relatively high socioeconomic status of our sample was important to our results, as prior research has shown a diverse pattern of FPN-DMN anti-correlations associated with cognitive performance in different socioeconomic groups [42].
An additional limitation of our research was a relative lack of pre-post cognitive and physiological measures. In the present study, we longitudinally measured only overall pleasant feeling, perceived exertion, and heart rate. Future studies should include a greater variety of measures taken before, during, and after acute exercise to better understand specific patterns of rsFC change. While statistical power in our analysis benefited from dimension reduction through multivariate pattern analysis and a within-participant design, our results can only be interpreted with respect to our modest sample size. Future research should replicate our data-driven approach with classical inferential statistics in an independent sample, ideally enriched with a large sample size and deep phenotyping to better understand potential moderating effects. Finally, sensitivity analyses indicated that in-scanner head motion as the most robust confound in second-level analyses, despite our stringent motion correction. However, no MRI denoising strategy is perfect [106]. Future research should carefully control head motion in this population, especially after extended periods of body movements, such as exercise. Our results therefore stand as a critical first step towards characterizing the neural network changes associated with acute exercise in youth.
CONCLUSION
In summary, we found acute exercise in youth led to connectivity change in resting-state functional networks across the whole brain, with increased rsFC within the FPN, between the FPN and basal ganglia, and heightened anti-correlation between the FPN and DMN. The seated reading control condition demonstrated contrasting rsFC changes, suggesting a distinct effect of the common control condition on neural network architecture. Our findings underscore the value of acute exercise as a modulator of rsFC patterns in the developing brain, suggesting an avenue through which physical activity may contribute to cognitive and mental health outcomes. While our research represents an early exploration into the topic, we offer a promising steppingstone for future investigations. As future research expands upon our findings, acute exercise could be utilized alone or with other behavioral interventions as an effective and accessible tool to optimize brain function, supporting cognitive and academic performance, neural development, and potentially buffering against threats to mental health.
ACKNOWLEDGMENTS
The authors have no acknowledgments to report.
The raw and processed data that support the findings of this study are available from the corresponding author upon reasonable request.
CONFLICT OF INTEREST
The authors declare no conflict of interest.
FUNDING
The authors have no funding to report.
SUPPLEMENTARY MATERIAL
[1] The supplementary material is available in the electronic version of this article: https://dx.doi.org/10.3233/BPL-240003.
REFERENCES
[1] | Piercy KL , Troiano RP , Ballard RM , et al. The Physical Activity Guidelines for Americans. JAMA. (2018) ;320: :2020–2028. |
[2] | Powell KE , King AC , Buchner DM , et al. The Scientific Foundation for the Physical Activity Guidelines for Americans, 2nd Edition. J Phys Act Health. (2018) :1–11. |
[3] | Hillman CH , Logan NE , Shigeta TT . A review of acute physical activity effects on brain and cognition in children. Translational Journal of the American College of Sports Medicine. (2019) ;4: :132–136. |
[4] | Raine LB , Kao S-C , Pindus D , et al. A Large-Scale Reanalysis of Childhood Fitness and Inhibitory Control. Journal of Cognitive Enhancement. (2018) ;2: :170–192. |
[5] | Smith PJ , Merwin RM . The Role of Exercise in Management of Mental Health Disorders: An Integrative Review. Annu Rev Med. (2021) ;72: :45–62. |
[6] | Hillman CH , Erickson KI , Kramer AF . Be smart, exercise your heart: exercise effects on brain and cognition. Nature Reviews Neuroscience. (2008) ;9: :58–65. |
[7] | Donnelly JE , Hillman CH , Castelli D , et al. Physical Activity, Fitness, Cognitive Function, and Academic Achievement in Children: A Systematic Review. Med Sci Sports Exerc. (2016) ;48: :1197–1222. |
[8] | Leahy AA , Mavilidi MF , Smith JJ , et al. Review of High-Intensity Interval Training for Cognitive and Mental Health in Youth. Med Sci Sports Exerc. (2020) ;52: :2224–2234. |
[9] | Rodriguez-Ayllon M , Cadenas-Sanchez C , Estevez-Lopez F , et al. Role of Physical Activity and Sedentary Behavior in the Mental Health of Preschoolers, Children and Adolescents: A Systematic Review and Meta-Analysis. Sports Med. (2019) ;49: :1383–1410. |
[10] | Verburgh L , Konigs M , Scherder EJ , Oosterlaan J . Physical exercise and executive functions in preadolescent children, adolescents and young adults: a meta-analysis. Br J Sports Med. (2014) ;48: :973–979. |
[11] | Erickson KI , Hillman C , Stillman CM , et al. Physical Activity, Cognition, and Brain Outcomes: A Review of the 2018 Physical Activity Guidelines. Med Sci Sports Exerc. (2019) ;51: :1242–1251. |
[12] | Pontifex MB , McGowan AL , Chandler MC , et al. A primer on investigating the after effects of acute bouts of physical activity on cognition. Psychology of Sport and Exercise. (2019) ;40: :1–22. |
[13] | Basso JC , Suzuki WA . The Effects of Acute Exercise on Mood, Cognition, Neurophysiology, and Neurochemical Pathways: A Review. Brain Plast. (2017) ;2: :127–152. |
[14] | Niven A , Laird Y , Saunders DH , Phillips SM . A systematic review and meta-analysis of affective responses to acute high intensity interval exercise compared with continuous moderate- and high-Intensity exercise. Health Psychol Rev. (2020) :1–34. |
[15] | Ai JY , Chen FT , Hsieh SS , et al. The Effect of Acute High-Intensity Interval Training on Executive Function: A Systematic Review. Int J Environ Res Public Health. (2021) ;18. |
[16] | Chang YK , Labban JD , Gapin JI , Etnier JL . The effects of acute exercise on cognitive performance: a meta-analysis. Brain Res. (2012) ;1453: :87–101. |
[17] | Herold F , Aye N , Lehmann N , Taubert M , Muller NG . The Contribution of Functional Magnetic Resonance Imaging to the Understanding of the Effects of Acute Physical Exercise on Cognition. Brain Sci. (2020) ;10. |
[18] | Hillman CH , Kamijo K , Scudder M . A review of chronic and acute physical activity participation on neuroelectric measures of brain health and cognition during childhood. Prev Med. (2011) ;52 Suppl 1: :S21–28. |
[19] | Hillman CH , Pontifex MB , Raine LB , et al. The effect of acute treadmill walking on cognitive control and academic achievement in preadolescent children. Neuroscience. (2009) ;159: :1044–1054. |
[20] | Polich J . Updating P an integrative theory of P3a and P3b. Clin Neurophysiol. (2007) ;118: :2128–2148. |
[21] | Kamijo K , Pontifex MB , O’Leary KC , et al. The effects of an afterschool physical activity program on working memory in preadolescent children. Dev Sci. (2011) ;14: :1046–1058. |
[22] | Hillman CH , Snook EM , Jerome GJ . Acute cardiovascular exercise and executive control function. Int J Psychophysiol. (2003) ;48: :307–314. |
[23] | Pontifex MB , Hillman CH , Fernhall B , Thompson KM , Valentini TA . The effect of acute aerobic and resistance exercise on working memory. Med Sci Sports Exerc. (2009) ;41: :927–934. |
[24] | Kamijo K , Hayashi Y , Sakai T , et al. Acute effects of aerobic exercise on cognitive function in older adults. J Gerontol B Psychol Sci Soc Sci. (2009) ;64: :356–363. |
[25] | Crabbe JB , Dishman RK . Brain electrocortical activity during and after exercise: A quantitative synthesis. Psychophysiology. (2004) ;41: :563–574. |
[26] | Lambourne K , Tomporowski P . The effect of exercise-induced arousal on cognitive task performance: A meta-regression analysis. Brain Res. (2010) ;1341: :12–24. |
[27] | Polich J . 50+years of P Where are we now? Psychophysiology. (2020) ;57: :e13616. |
[28] | Beaussart M , Niquet G , Gaudier E , Guislain F . The EEG of boxersexamined immediately after combat. Comparative study with the EEGrecorded before combat in 52 cases., Revista de Obstetricia yGinecología de Venezuela. (1959) ;101: :422–427. |
[29] | Klimesch W . Alpha-band Oscillations, Attention, and Controlled Access to Stored Information. Trends Cogn Sci. (2012) ;16: :606–617. |
[30] | Boecker H , Hillman CH , Scheef L , Strüder HK , (Eds.). Functionalneuroimaging in exercise and sport sciences. Springer Science & Business Media; (2012) . |
[31] | Fox MD , Raichle ME . Spontaneous fluctuations in brain activity observed with functional magnetic resonance imaging. Nat Rev Neurosci. (2007) ;8: :700–711. |
[32] | Fox PT , Raichle ME . Focal physiological uncoupling of cerebral blood flow and oxidative metabolism during somatosensory stimulation in human subjects. Proceedings of the National Academy of Sciences. (1986) ;83: :1140–1144. |
[33] | Logothetis NK , Wandell BA . Interpreting the BOLD signal. Annu Rev Physiol. (2004) ;66: :735–769. |
[34] | Boccaletti S , Latora V , Moreno Y , Chavez M , Hwang D . Complex networks: Structure and dynamics. Physics Reports. (2006) ;424: :175–308. |
[35] | Niendam TA , Laird AR , Ray KL , et al. Meta-analytic evidence for a superordinate cognitive control network subserving diverse executive functions. Cogn Affect Behav Neurosci. (2012) ;12: :241–268. |
[36] | Gratton C , Sun H , Petersen SE . Control networks and hubs. Psychophysiology. (2018) ;55. |
[37] | Raichle ME , MacLeod AM , Snyder AZ , et al. A default mode of brain function. Proceedings of the national academy of sciences. (2001) ;98: :676–682. |
[38] | Chai XJ , Ofen N , Gabrieli JD , Whitfield-Gabrieli S . Selective development of anticorrelated networks in the intrinsic functional organization of the human brain. J Cogn Neurosci. (2014) ;26: :501–513. |
[39] | Ernst M , Torrisi S , Balderston N , Grillon C , Hale EA . fMRI functional connectivity applied to adolescent neurodevelopment. Annu Rev Clin Psychol. (2015) ;11: :361–377. |
[40] | Hampson M , Driesen N , Roth JK , Gore JC , Constable RT . Functional connectivity between task-positive and task-negative brain areas and its relation to working memory performance. Magn Reson Imaging. (2010) ;28: :1051–1057. |
[41] | Keller JB , Hedden T , Thompson TW , et al. Resting-state anticorrelations between medial and lateral prefrontal cortex: association with working memory, aging, and individual differences. Cortex. (2015) ;64: :271–280. |
[42] | Ellwood-Lowe ME , Whitfield-Gabrieli S , Bunge SA . Brain network coupling associated with cognitive performance varies as a function of a child’s environment in the ABCD study. Nat Commun. (2021) ;12: :7183. |
[43] | Whitfield-Gabrieli S , Ford JM . Default mode network activity and connectivity in psychopathology. Annu Rev Clin Psychol. (2012) ;8: :49–76. |
[44] | Whitfield-Gabrieli S , Wendelken C , Nieto-Castanon A , et al. Association of Intrinsic Brain Architecture With Changes in Attentional and Mood Symptoms During Development. JAMA Psychiatry. (2020) ;77: :378–386. |
[45] | Bauer CCC , Rozenkrantz L , Caballero C , et al. Mindfulness training preserves sustained attention and resting state anticorrelation between default-mode network and dorsolateral prefrontal cortex: A randomized controlled trial. Hum Brain Mapp. (2020) ;41: :5356–5369. |
[46] | Gallen CL , D’Esposito M . Brain Modularity: A Biomarker of Intervention-related Plasticity. Trends Cogn Sci. (2019) ;23: :293–304. |
[47] | Cahn BR , Polich J . Meditation states and traits: EEG, ERP, and neuroimaging studies. Psychol Bull. (2006) ;132: :180–211. |
[48] | Norris CJ , Creem D , Hendler R , Kober H . Brief Mindfulness Meditation Improves Attention in Novices: Evidence From ERPs and Moderation by Neuroticism. Front Hum Neurosci. (2018) ;12: :315. |
[49] | Chen A-G , Zhu L-N , Xiong X , Li Y . Acute aerobic exercise altersexecutive control network in preadolescent children. Revista depsicología del deporte. (2017) ;26: :132–137. |
[50] | Rajab AS , Crane DE , Middleton LE , et al. A single session of exercise increases connectivity in sensorimotor-related brain networks: a resting-state fMRI study in young healthy adults. Front Hum Neurosci. (2014) ;8: :625. |
[51] | Schmitt A , Upadhyay N , Martin JA , et al. Modulation of Distinct Intrinsic Resting State Brain Networks by Acute Exercise Bouts of Differing Intensity. Brain Plast. (2019) ;5: :39–55. |
[52] | Voss MW , Weng TB , Narayana-Kumanan K , et al. Acute Exercise Effects Predict Training Change in Cognition and Connectivity. Med Sci Sports Exerc. (2020) ;52: :131–140. |
[53] | Weng TB , Pierce GL , Darling WG , et al. The Acute Effects of Aerobic Exercise on the Functional Connectivity of Human Brain Networks. Brain Plast. (2017) ;2: :171–190. |
[54] | Cooper CJ . Anatomical and physiological mechanisms of arousal with specific reference to the effects of exercise. Ergonomics. (1973) ;16: :601–609. |
[55] | Nieto-Castanon A . Brain-wide connectome inferences using functional connectivity MultiVariate Pattern Analyses (fc-MVPA). PLoS Comput Biol. (2022) ;18: :e1010634. |
[56] | McNorgan C , Smith GJ , Edwards ES . Integrating functional connectivity and MVPA through a multiple constraint network analysis. Neuroimage. (2020) ;208: :116412. |
[57] | Raine LB , McDonald K , Shigeta TT , et al. Sympathetic Nervous System and Exercise Affects Cognition in Youth (SNEACY): study protocol for a randomized crossover trial. Trials. (2021) ;22: :154. |
[58] | Thomas S , Reading J , Shephard RJ . Revision of the physical activity readiness questionnaire. PAR-Q. Can J Sport Sci. (1992) ;17: :338–345. |
[59] | Kaufman AS , Kaufman NL . Kaufman Test of Educational Achievement. 2nd ed. Circle Pines: Pearson; (2004) . |
[60] | American College of Sports Medicine. ACSM’s Guidelines for ExerciseTesting and Prescription. 11 ed: Lippincott Williams & Wilkins; (2022) . |
[61] | Hardy CJ , Rejeski WJ . Not what, but how one feels: the measurement of affect during exercise. J Sport Exerc Psychol. (1989) ;11: :304–317. |
[62] | Robertson RJ , Goss FL , Boer NF , et al. Children’s Omni scale ofperceived exertion: Mixed gender and race validation. Medicine &Science in Sports & Exercise. Medicine & Science in Sports &Exercise. (2000) ;32: :452–458. |
[63] | Ekkekakis P , Hartman M , Ladwig M . Affective Responses to Exercise. In: Tenenbaum G, Eklund R eds, Handbook of Sports Psychology: JohnWiley & Sons, Inc; (2020) :231–253. |
[64] | Tisdall MD , Hess AT , Reuter M , et al. Volumetric navigators for prospective motion correction and selective reacquisition in neuroanatomical MRI. Magn Reson Med. (2012) ;68: :389–399. |
[65] | Whitfield-Gabrieli S , Nieto-Castanon A . Conn: a functional connectivity toolbox for correlated and anticorrelated brain networks. Brain Connect. (2012) ;2: :125–141. |
[66] | Andersson JL , Skare S , Ashburner J . How to correct susceptibility distortions in spin-echo echo-planar images: application to diffusion tensor imaging. Neuroimage. (2003) ;20: :870–888. |
[67] | Smith SM , Jenkinson M , Woolrich MW , et al. Advances in functional and structural MR image analysis and implementation as FSL. Neuroimage. (2004) ;23 Suppl 1: :S208–219. |
[68] | Andersson JL , Hutton C , Ashburner J , Turner R , Friston K . Modeling geometric deformations in EPI time series. Neuroimage. (2001) ;13: :903–919. |
[69] | Whitfield-Gabrieli S , Nieto-Castanon A , Ghosh S . Artifact detection tools (ART). Cambridge MA. (2011) ;Release Version 7. |
[70] | Power JD , Mitra A , Laumann TO , et al. Methods to detect, characterize, and remove motion artifact in resting state fMRI. Neuroimage. (2014) ;84: :320–341. |
[71] | Calhoun VD , Wager TD , Krishnan A , et al. The impact of T1 versus EPI spatial normalization templates for fMRI data analyses. Hum Brain Mapp. (2017) ;38: :5331–5342. |
[72] | Ashburner J , Friston KJ . Unified segmentation. Neuroimage. (2005) ;26: :839–851. |
[73] | Nieto-Castanon A . Handbook of functional connectivity MagneticResonance Imaging methods in CONN: Hilbert Press; (2020) . |
[74] | Behzadi Y , Restom K , Liau J , Liu TT . A component based noise correction method (CompCor) for BOLD and perfusion based fMRI. Neuroimage. (2007) ;37: :90–101. |
[75] | Chai XJ , Castanon AN , Ongur D , Whitfield-Gabrieli S . Anticorrelations in resting state networks without global signal regression. Neuroimage. (2012) ;59: :1420–1428. |
[76] | Morfini F , Whitfield-Gabrieli S , Nieto-Castañón A . Functional connectivity MRI quality control procedures in CONN. Frontiers in Neuroscience. (2023) ;17. |
[77] | Westfall DR , Anteraper SA , Chaddock-Heyman L , et al. Resting-State Functional Connectivity and Scholastic Performance in Preadolescent Children: A Data-Driven Multivoxel Pattern Analysis (MVPA). Journal of Clinical Medicine. (2020) ;9. |
[78] | Morris TP , Chaddock-Heyman L , Ai M , et al. Enriching activities during childhood are associated with variations in functional connectivity patterns later in life. Neurobiol Aging. (2021) ;104: :92–101. |
[79] | Whitfield-Gabrieli S , Ghosh SS , Nieto-Castanon A , et al. Brain connectomics predict response to treatment in social anxiety disorder. Mol Psychiatry. (2016) ;21: :680–685. |
[80] | Anteraper SA , Collin G , Guell X , et al. Altered resting-state functional connectivity in young children at familial high risk for psychotic illness: A preliminary study. Schizophr Res. (2020) ;216: :496–503. |
[81] | Logan NE , Westfall DR , Raine LB , et al. The Differential Effects of Adiposity and Fitness on Functional Connectivity in Preadolescent Children. Med Sci Sports Exerc. (2022) ;54: :1702–1713. |
[82] | Wysocki AC , Lawson KM , Rhemtulla M . Statistical Control Requires Causal Justification. Advances in Methods and Practices in Psychological Science. (2022) ;5: :1–19. |
[83] | Noble S , Mejia AF , Zalesky A , Scheinost D . Improving power in functional magnetic resonance imaging by moving beyond cluster-level inference. Proc Natl Acad Sci U S A. (2022) ;119: :e2203020119. |
[84] | Yeo BT , Krienen FM , Sepulcre J , et al. The organization of the human cerebral cortex estimated by intrinsic functional connectivity. J Neurophysiol. (2011) ;106: :1125–1165. |
[85] | Vul E , Harris C , Winkielman P , Pashler H . Puzzlingly high correlations in fMRI studies of emotion, personality, and social cognition. Perspectives on psychological science. (2009) ;4: :274–290. |
[86] | Choi EY , Yeo BT , Buckner RL . The organization of the human striatum estimated by intrinsic functional connectivity. J Neurophysiol. (2012) ;108: :2242–2263. |
[87] | Miller EK . The prefrontal cortex and cognitive control. Nat Rev Neurosci. (2000) ;1: :59–65. |
[88] | Yin HH , Knowlton BJ . The role of the basal ganglia in habit formation. Nat Rev Neurosci. (2006) ;7: :464–476. |
[89] | Parent A , Hazrati LN . Functional anatomy of the basal ganglia. The cortico-basal ganglia-thalamo-cortical loop. Brain research reviews. (1995) ;10: :91–127. |
[90] | Schneider S , Vogt T , Frysch J , Guardiera P , Struder HK . School sport–a neurophysiological approach. Neurosci Lett. (2009) ;467: :131–134. |
[91] | Schneider S , Askew CD , Abel T , Mierau A , Struder HK . Brain and exercise: a first approach using electrotomography. Med Sci Sports Exerc. (2010) ;42: :600–607. |
[92] | Krafft CE , Pierce JE , Schwarz NF , et al. An eight month randomized controlled exercise intervention alters resting state synchrony in overweight children. Neuroscience. (2014) ;256: :445–455. |
[93] | Cavanna AE , Trimble MR . The precuneus: a review of its functional anatomy and behavioural correlates. Brain. (2006) ;129: :564–583. |
[94] | Salehinejad MA , Ghanavati E , Rashid MHA , Nitsche MA . Hot and cold executive functions in the brain: A prefrontal-cingular network. Brain Neurosci Adv. (2021) ;5: :23982128211007769. |
[95] | Grimm S , Beck J , Schuepbach D , et al. Imbalance between left and right dorsolateral prefrontal cortex in major depression is linked to negative emotional judgment: an fMRI study in severe major depressive disorder. Biol Psychiatry. (2008) ;63: :369–376. |
[96] | Mikkelsen K , Stojanovska L , Polenakovic M , Bosevski M , Apostolopoulos V . Exercise and mental health. Maturitas. (2017) ;106: :48–56. |
[97] | McDowell CP , Dishman RK , Gordon BR , Herring MP . Physical Activity and Anxiety: A Systematic Review and Meta-analysis of Prospective Cohort Studies. Am J Prev Med. (2019) ;57: :545–556. |
[98] | Schuch FB , Vancampfort D , Firth J , et al. Physical Activity and Incident Depression: A Meta-Analysis of Prospective Cohort Studies. Am J Psychiatry. (2018) ;175: :631–648. |
[99] | Dunn AL , Jewell JS . The effect of exercise on mental health. Current Sports Medicine Reports. (2010) ;9: :202–207. |
[100] | Sahin NT , Pinker S , Cash SS , Schomer D , Halgren E . Sequential processing of lexical, grammatical, and phonological information within Broca’s area. Science. (2009) ;326: :445–449. |
[101] | Poldrack RA , Wagner AD , Prull MW , et al. Functional specialization for semantic and phonological processing in the left inferior prefrontal cortex. Neuroimage. (1999) ;10: :15–35. |
[102] | Martin A , Schurz M , Kronbichler M , Richlan F . Reading in the brain of children and adults: a meta-analysis of 40 functional magnetic resonance imaging studies. Hum Brain Mapp. (2015) ;36: :1963–1981. |
[103] | Gabrieli JD , Desmond JE , Demb JB , et al. Functional magnetic resonance imaging of semantic memory processes in the frontal lobes. Psychological Science. (1996) ;7: :278–283. |
[104] | Pontifex MB , Parks AC , Henning DA , Kamijo K . Single bouts of exercise selectively sustain attentional processes. Psychophysiology. (2015) ;52: :618–625. |
[105] | Vogel AC , Church JA , Power JD , et al. Functional network architecture of reading-related regions across development. Brain Lang. (2013) ;125: :231–243. |
[106] | Parkes L , Fulcher B , Yucel M , Fornito A . An evaluation of the efficacy, reliability, and sensitivity of motion correction strategies for resting-state functional MRI. Neuroimage. (2018) ;171: :415–436. |