Precision Exercise Medicine: Sex Specific Differences in Immune and CNS Responses to Physical Activity
Abstract
Physical activity is a powerful lifestyle factor capable of improving cognitive function, modifying the risk for dementia associated with neurodegeneration and possibly slowing neurodegenerative disease progression in both men and women. However, men and women show differences in the biological responses to physical activity and in the vulnerabilities to the onset, progression and outcome of neurodegenerative diseases, prompting the question of whether sex-specific regulatory mechanisms might differentially modulate the benefits of exercise on the brain. Mechanistic studies aimed to better understand how physical activity improves brain health and function suggest that the brain responds to physical exercise by overall reducing neuroinflammation and increasing neuroplasticity. Here, we review the emerging literature considering sex-specific differences in the immune system response to exercise as a potential mechanism by which physical activity affects the brain. Although the literature addressing sex differences in this light is limited, the initial findings suggest a potential influence of biological sex in the brain benefits of exercise, and lay out a scientific foundation to support very much needed studies investigating the potential effects of sex-differences on exercise neurobiology. Considering biological sex and sex-differences in the neurobiological hallmarks of exercise will help to enhance our understanding of the mechanisms by which physical activity benefits the brain and also improve the development of treatments and interventions for diseases of the central nervous system.
INTRODUCTION
Biological differences between men and women are normally attributed to genetic, developmental, social and hormonal differences across the lifespan (Fig. 1). Although there exists a considerable overlap between both sexes as well as large distributions within each sex for each of these variables, differences at each of them can lead to unique regulatory mechanisms with significant and sex-specific impacts on overall health, including brain health and function. For example, human males show a 10–12 % larger volume of certain brain regions [1] and genetic sex differences in gene content and dosage [2] are known to lead to differences in immune regulation and brain function [3–5]. Indeed, sex-specific differences extend beyond brain structure and gene expression, as men and women reveal differing vulnerabilities to the onset and outcome of neurodegenerative diseases that vary with the type of neurodegenerative disorder [6]. For example, women are at higher risk of Alzheimer’s disease (AD) and multiple sclerosis (MS), while men are at higher risk of Parkinson’s disease (PD) and amyotrophic lateral sclerosis (ALS) [6, 7]. In contrast, men, when they do get AD, appear to die earlier than women with the same diagnosis [8–10], independent of any co-morbidities. Similarly, men with MS are more likely to evolve to a progressive disease stage, are more prone to cognitive decline and more likely to develop atrophy of subcortical grey matter structures [11–13] compared to women with MS. Likewise, males with PD show significantly greater executive and processing speed impairments [14] despite females showing greater disease pathology [15]. Males also appear to be at a higher risk of getting vascular dementia than females at a younger age, but with aging this relationship reverses and women over the age of 85 have been shown to have a higher occurrence [16]. Despite these differences, there are also some similarities on disease presentation across sexes: both men and women with ALS show similar prognosis and survival rate [17]. This suggests both sex-specific as well as shared disease-associated pathological mechanisms underlying neurodegenerative diseases across sexes. In accordance with these findings, brain transcriptome profiles of women and men show the existence of male biased genes involved in neurological and psychiatric disorders like schizophrenia, bipolar disorder, Alzheimer’s disease and autism [18].
Fig. 1
Depicts contributing factors associated with differences between men and women: normally attributed to genetic, developmental, social, environmental, physiological, behavioral and hormonal differences across the lifespan.
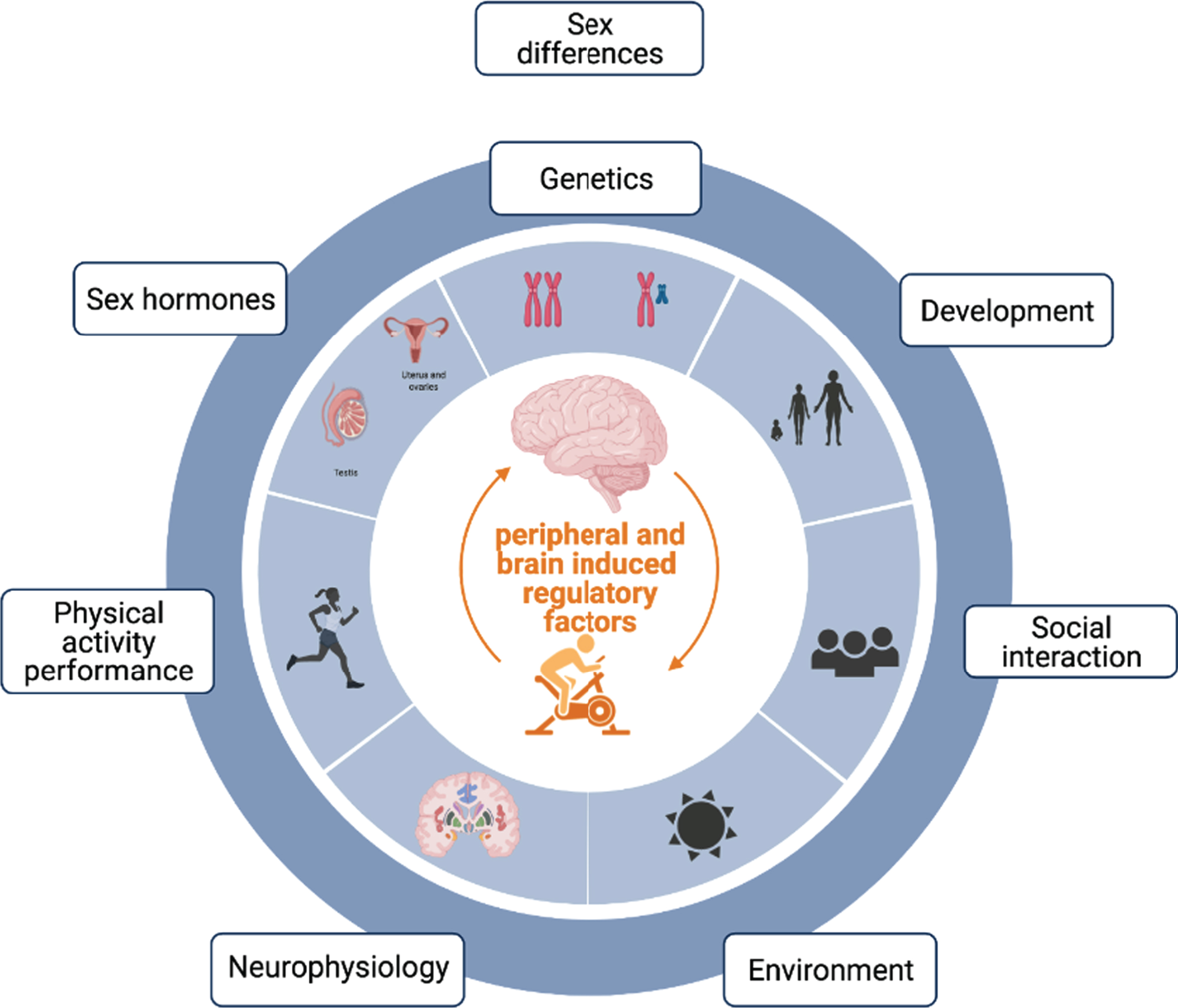
Neuroinflammation, which is a central pathological feature of all of these neurodegenerative diseases [19], has been linked to both age- and disease-associated cognitive impairment and is a good place to begin to look for mechanisms underlying sex differences in the development and progression of neurodegenerative disease. Indeed, systemic inflammation has been linked to neuroinflammation and identified as a risk factor for neurodegenerative disease [20, 21]. Interestingly, this effect might also be sex dependent. Male mice show a greater gene and protein hippocampal expression of neuroinflammatory markers such as interleukin 1α (IL-1α), interleukin-1β (IL-1β), interleukin-4 (IL-4), interleukin-6 (IL-6), tumor necrosis factor α (TNFα), C-C Motif Chemokine Ligand 2 (CCL2), and C-X-C Motif Chemokine Ligand 10 (CXCL10) than female mice when peripherally stimulated with polyinosinic:polycytidylic acid (poly I:C), a synthetic viral mimic [22]. Additionally, only male mice showed increased hippocampal increases of interferon α (IFNα) and interferon γ (IFNγ) in response to poly I:C, suggesting that there are specific sex differences in the peripherally-induced anti-viral response in the hippocampus [22].
Given our recent understanding that peripheral inflammation is likely to interfere with immunological processes of the brain and further promote disease progression, sex differences in mechanisms underlying both central and systemic inflammation need to be considered in the context of neurodegenerative disease.
Exercise is a behavioral intervention with potent benefits on both neuroinflammation and peripheral inflammation, and is currently recognized as a powerful lifestyle factor to promote and preserve cognitive function. In general, physical exercise is considered a significant modifier for the risk for dementia associated with neurodegenerative disease and vascular dementia [23, 24], and may possibly also slow disease progression in both men and women. Mechanistic studies aimed to better understand how physical activity affects the brain and its function have associated the positive effects of exercise with increased neurotrophic factors, neuroplasticity (i.e. Increase in neurogenesis and synaptic connections), vascular support and reduced neuroinflammation within the hippocampus. However, despite the evidence supporting the benefits of exercise training to improve cognitive and brain health in both men and women, our understanding of how females respond to physical activity is severely underpowered [25], with a majority of studies being done in men and male animal models. To begin to develop a better understanding of the differences between females and males, here we focus on the literature examining the sex-specific differences of the immune system response to exercise with potential differing effects on the brain. This review adds to the small but growing body of literature examining the effects of sex-specific differences in neuroplasticity, neurotrophic factors and physiological effects of exercise as possible mediators of sex differences in exercise efficacy on cognition (for reviews see: [26–28])
ARE THERE SEX-SPECIFIC DIFFERENCES IN THE COGNITIVE BENEFITS OF EXERCISE?
Physical activity is a powerful preventative intervention capable of modifying the risk for dementia in women and men [23]. For example, regular exercise has been found to reduce the risk of developing AD by 45% [29], while individuals with sedentary lifestyles are 53% more likely to develop AD than those who engaged in more active lives [30]. Another study found a lower incidence of all-cause dementia and vascular dementia in high performance skiers, with no association of higher physical activity with AD [24]. It is worth noting, however, that previous meta-analyses on the effects of physical exercise on cognitive function have found large heterogeneity between studies [31–35], possibly due to challenges associated with differential study setups, sampling approaches, mixed dementia etiologies (such as AD vs. pure vascular dementia vs. others) causation and ideal follow-up time [36], comorbidities [37], and potentially also due to inter-individual differences, such as the biological sex of the trial participants [38].
However, some available studies suggest that potential sex-differences associated with exercise and physical activity do exist. For example, in populations with mild-cognitive impairment (MCI), the effect size for women undergoing an aerobic exercise intervention on executive function was increased by two-fold in comparison to men [39].
Meta-analysis and retrospective studies suggest sex-differences in the cognitive benefits of exercise
To date, there are significantly fewer reports examining the neurocognitive benefits of exercise on women compared to men, and these often come from retrospective cohort studies, making determination of causality difficult, and increasing the potential for unmeasured confounding variables. Indeed, the results of one meta-analysis [40] of 15 prospective cohort studies following up 33,816 individuals without dementia for 1–12 years reported that physical activity had a significant protective effect against cognitive decline. Among those, only three studies conducted analyses separately for men and women with all suggesting that the effect of physical activity is most protective against cognitive decline in women. A separate retrospective meta-analysis of randomized control trials found that, in particular, females aged more than 55 years showed greater cognitive benefits from aerobic training paradigms compared to age matched male participants [41]. Interestingly, an additional meta-analysis also showed that a similar sex-bias may also exist in exercise responses during healthy aging in rodents. Forced aerobic training enhanced hippocampus-dependent learning and memory to a greater extent in female rodents, whereas voluntary aerobic training enhanced hippocampal non-spatial memory only in males [42].
Conversely, a separate and more recent systematic review and meta-analysis concluded that exercise was less effective in female compared with male individuals, and the dose–response relationship differed between sexes [38]. These seemingly contradictory results highlight the need for dedicated studies using sex as a variable during analysis.
Sex-differences in brain volume changes associated with exercise and physical activity
Human studies show that multimodal training (e.g., combined aerobic and resistance training) may provide a female advantage for the improvements in executive functions [41], often associated with maintenance of volume of specific brain regions. For example, higher levels of daily walking activity were significantly associated with larger hippocampal volumes among older women but not among age-matched men [43]. Further analysis revealed that this sex-bias benefit was particularly significant in the subiculum, a part of the dorsal hippocampus, and a key affected region in AD patients. In contrast, recent evidence suggests that atrophy of the posterior subiculum is also a feature of non-demented, aged individuals with AD pathology and mild memory disfunction [44]. A separate study recently showed that maintaining physical activity levels over 10 years was significantly associated with greater volume of the left dorsolateral prefrontal cortex, another brain region involved in executive function, in females only [45]. Interestingly, in this cohort, walking activity was indeed associated with a larger left hippocampus in older males and a smaller hippocampus in older females, while the maintenance of physical activity over time predicted better global cognitive function for both sexes [45]. Although this discrepancy in physical activity levels and hippocampal volumes may appear contradictory, these differences may be attributed to the measurements of walking behaviors (i.e. Estimations or self-reports) and/or the duration of direct measurements. Indeed, many of the seemingly contradictory findings in exercise medicine are currently believed to be attributed to differences in activity measurements and reports [46]. Furthermore, more insight into the mechanistic and causal effects of physical activity as a modulator of brain volume is necessary. This is why the Molecular Transducers of Physical Activity Consortium (MoTrPAC) has recently begun mapping the dynamic responses to exercise in both human participants and rat models under standardized exercise training interventions [46]. Importantly, despite the lack of understanding of the effects of exercise and physical activity on brain architecture, accumulating evidence from interventional and epidemiological studies suggest that engagement in targeted exercise training as well as longitudinal maintenance of physical activity, is associated with better performance in different domains of cognition in females compared to males [26].
Altogether, the literature in humans support the hypothesis that there exist sex-specific benefits of exercise for brain function, thus making it tempting to speculate that the neurobiological and physiological mechanisms underlying the effects of exercise on the brain and its function can be sex-specific. Even more so, these findings imply that the neurocognitive benefits of exercise and physical activity may be particularly relevant for aging women, especially in light of some epidemiological studies suggesting that older women are more sedentary and engage in less physical activity than age-matched men [47, 48].
HOW CAN SEX-DIFFERENCES AFFECT THE BRAIN AND ITS FUNCTION?
Sex-specific differences in the immune response to physical exercise
Many of the cognitive benefits induced by physical exercise on learning and memory have been linked to the anti-inflammatory effects of exercise [49, 50]. Studies in humans and animal models have shown that physical activity and exercise transiently increase the production and release of circulating factors, collectively named exerkines [51] and reduce the expression of toll-like receptors (TLRs) which are involved in the recognition of and response to microbial infections and their stimulation induces the production of cytokines and chemokines. Some exercise-induced factors, including the production and release of anti-inflammatory cytokines from skeletal muscle [49, 52] down regulate pro-inflammatory immune responses and have been linked to improved cognitive function and brain health. Analysis of human peripheral blood following exercise has also revealed a reduction in the circulating numbers of pro-inflammatory monocytes [53] and an increase in the circulatory numbers of regulatory T cells [54]. In rodents, a similar increase in the circulating numbers of regulatory T cells has also been reported after exercise [55].
Interestingly, among mammals and during non-exercise conditions, females and males already show differences in their immune system composition and function [56, 57], with females generally showing a larger and faster innate and adaptive immune responses to pathogens or injury [56]. Consistent with this finding, female mice have greater density of TLRs than age-matched male mice, and this observation has been shown to be estrogen-dependent [58]. Thus, it is possible that the anti-inflammatory effects of exercise, including its effects on immune cell number and function, are different in females and males. To date, only a few published studies have begun exploring this possibility by looking at differences in immune regulatory factors and immune function between females and males in response to physical activity, which we summarize below.
Sex differences in exerkines as exercise-induced immune regulatory factors and the brain
A growing body of literature supports the existence of crosstalk between the brain and many distant organs and systems in our body. In particular, the brain crosstalks with the immune system, the hormonal system, gut, fat, muscle, heart, pancreas and bone, among others. Multisystemic activation of these systems during physical activity induces the release of factors referred to as “exerkines” [59] that are necessary for the homeostatic adaptation to exercise [59–61] and ultimately can regulate brain function and health. Indeed, recent literature in animal models has shown that cognition, neural plasticity, and brain inflammation can be improved by peripheral circulatory factors increased with exercise, such as irisin [62], cathepsin B [63], insulin-like growth factor-1 (IGF-1) [64], vascular endothelial growth factor (VEGF) [65] and lactate [66, 67], clusterin (CLU) [68], glycerophosphoryl diester phosphodiesterase-like protein (GPDL1) [69] and platelet factor 4 (PF4) [70].
In recent years, skeletal muscle has arisen as one of the largest secretory organs, releasing cytokines and other peptides (named myokines, a type of exerkine [50, 71]) into the circulatory system, where they can reach distant organs and tissues [72] including the brain [68, 69]. One of the most studied muscle-originating exerkine is IL-6 [73–75] which increases up to 100 fold in circulation during physical activity [76, 77]. Interleukin-6 is a particularly interesting exercise-responsive myokine, as it is one of the few that can efficiently cross the blood brain barrier [77, 78] and may thus directly target the CNS. Brain IL-6 has been shown to affect target cells via membrane bound IL-6R or trans-signaling [79] and affect astrocytic and neuronal differentiation of neural stem cells, with conflicting results in mice and in vitro studies [80–82]. Interestingly, sex differences have been reported in both the temporal pattern and magnitude of this response after a single bout of total body resistance exercise, with women participants taking 4 times longer to reach their IL-6 highest peak [83]. Another study in half-marathon runners with pre- and post-exercise measurements showed that transcripts of IL-6, (as well as other well-known inflammatory cytokine markers such as TNFAIP3 Interacting Protein 3 (TNIP-3), interleukin-8 (IL-8), CXCL10, C-X-C Motif Chemokine Ligand 10 (CXCL3), cluster of differentiation 69 (CD69) were differentially regulated between sexes in response to exercise in unstimulated whole blood cultures. Samples from female participants showed stronger increases in TNIP-3, IL-8, CXCL3, CD69, whereas male samples showed stronger differences for IL-6 and CXCL10 [84]. Another human study showed that self-reported physical activity is associated with a decrease in inflammatory chemokines (i.e. Macrophage derived chemokine (MDC), macrophage inflammatory protein-beta (MIP-1b), macrophage inflammatory protein - 4 (MCP-4), eotaxin-3, and improvement of brain structure and function (visual memory and processing speed) in late middle age men but not in women with comparable physical activity levels [85]. Although these results are confounded by the well-known sex-bias of self-reporting, it highlights the need for additional studies under controlled physical activity conditions (or chronic ‘exercisers’) to monitor any effects of long-term physical activity on brain function.
Irisin is another recently-discovered exerkine, also secreted from skeletal muscle and adipose tissue during exercise. Irisin also appears to cross the blood brain barrier, and initiates a neuroprotective genetic program in the hippocampus that culminates with increased expression of brain derived neurotrophic factor (BDNF) [62, 86]. Irisin has been shown to increase more in females than in males immediately after the start of the exercise [87], and this is mediated by tissue, sex hormones, obesity and age [88, 89]. In response to acute aerobic exercise, irisin levels increased significantly more in women than men [87], suggesting that these sex differences remain even under different exercise behavioral interventions. It is currently unknown whether these differences represent true sex-differences or are instead associated with other variables such as baseline exercise levels, genetic heterogeneity or others.
The list of immune regulating factors induced by skeletal muscle or other organs and tissues in response to exercise is extensive, including but not limited to interleukins IL-8, IL-10 and IL-15, tumor necrosis factor alpha (TNF-α), IL-1 receptor antagonist (IL-1ra), oncostatin M (OSM), osteocalcin, fibroblast growth factor-21 (FGF21), chemokine CXC motif ligand-1 (CXCL-1) and C-C motif chemokine ligand 2 (CCL-2), and meteorin-like (Metrnl), secreted protein acidic and cysteine rich (SPARC), myostatin (MSTN), and decorin (DCN), macrophage inflammatory protein α and β (MIP-1α and MIP-1β), apelin and clusterin [60, 68, 72, 90–93]. Several of these factors also have been shown to differ between sexes. For example, activation of peripheral blood mononuclear cells (PBMCs) from human males results in greater IL-10 and TNF production than PBMCs from females [94–96]. These differential responses in circulatory cytokines and chemokines in men and women, prompt us to suggest that the function and temporal responses of exercise induced immunomodulatory factors might be different between women and men. As mentioned before, when examined in pre-clinical animal models, most studies focus on male animals. Indeed, whether there exists a sex-specific proteomic profile in females and males in response to exercise reminds understudied. For example, one study analyzed the salivary proteome of men and women during rest and in response to an acute exercise. They found that 16 (out 87 significantly regulated proteins) were significantly regulated by sex. The most significantly regulated proteins were pregnancy zone protein alpha-2-macroglobulin like (PZP) and the isoform 2 PZP. PZP acts as an immune regulator and it is very similar to alpha-2-macroglobulin (A2M), a protease inhibitor can act as an inhibitor of inflammatory cytokines, and is a negative regulator of the complement pathway. Interestingly, A2M has been linked to Alzheimer Disease [97], suggesting a potential connection between this factor and brain function. A recent study analyzing the plasma proteome of female and male mice with access to a running wheel also found an overall and similar downregulation of the complement pathway in both sexes [68].
The introduction of studies using single-cell and other omics technologies (i.e. RNA-sequencing or mass cytometry) to study the effects of physical activity on gene and protein expression as an untargeted approach, are likely to increase the understanding of the effect of exercise on various organs, tissues and cells from the peripheral system, as well as their function in signaling the brain [98]. These techniques hold great potential to reveal further sex-differences in the response to exercise. For example, in a study by Rubenstein et al., 2020 [99] using the gene signatures obtained by single-cell RNA-sequencing on human skeletal muscle, they described a lower proportion of lymphocyte (e.g. NK cells, T cells, and B cells) gene sets, in the young female group than in any other group (old female, young male, and old male). A recent study from the Genotype-Tissue Expression (GTEx) consortium showed mRNA expression differences between the sexes across all tissues, with skeletal muscle showing the second largest number of differential gene expression after the brain [100]. Additionally, a meta-analysis study looking at the effect of sex on autosomal DNA methylation in human skeletal muscle found 8,420 differentially methylated genes, 94% of which were hypomethylated in males. Gene set enrichment analysis revealed that differentially methylated genes were involved in muscle metabolism and its function [101]. These transcriptomic differences could play an important role in modulating sex-differences in the exercise-induced secretome from muscle, and other tissues, with potential immune and brain regulatory functions.
Sex differences in regulation of the immune response through TLR expression
One of the main mechanisms by which physical activity is known to reduce inflammation, is by downregulating the expression of Toll-like receptors (TLRs) in innate immune myeloid cells. Toll-like receptors are pattern recognition receptors (PPRs) that regulate immune responses by recognizing invading pathogens as well as endogenous damage/danger signals [102–104]. TLRs are transmembrane receptors, with a variable number of leucine-rich repeats in the extracellular domain that mediates the recognition of pathogen- or endogenous damage-associated molecular patterns (PAMPs or DAMPs) and a cytoplasmatic Toll/interleukin-1 receptor (TIR) signaling domain, required for transmission of a signal to downstream pathway components. The TIR domain mediates interactions between TLRs and adaptor proteins involved in regulating TLR signaling. Depending on the particular TLR, receptor engagement can culminate in the induction of nuclear factor-kappa B (NF-κB), mitogen-activated protein kinases (MAPK), and/or interferon-regulatory factor (IRF) signaling pathways, which regulate the expression of a wide array of genes involved in inflammatory responses, such as the expression of pro-inflammatory cytokines, chemokines, and type I and type III interferons [105] and the control the induction of antigen-presenting (MHC class II) and costimulatory molecules (CD80/86) [106, 107].
In humans, physical activity has been shown to induce a downregulation of TLR1, TLR2 and TLR4 in circulating monocytes and macrophages [52, 108, 109], which is associated with decreased inflammatory cytokine production [110]. Interestingly during non-exercise conditions, a sex bias in TLR activation has been observed in cells and tissues of the periphery and the brain across species. For example, primary B lymphocytes, monocytes, and plasmacytoid dendritic cells in women generally show greater expression of toll-like receptor 7 (TLR7) [111, 112], and a greater TLR7 and TLR8 upregulation of immune-related transcriptional factors (e.g., TLR, greater production of interferon-α (IFNα)) following an immune challenge [113, 114]. This sex bias appears specific to these components of the TLR signaling pathway, as there appear to be no significant gender differences in TLR4, TLR3 and TLR9 pathways activity in response to their ligands [113, 115]. In accordance with human data, B lymphocytes from female mice show higher expression of Tlr7 in response to immunization with an inactivated H1N1 vaccine, and had greater antibody responses and protection against an H1N1 challenge than males [116]. Additionally, female mice and rats show a greater mRNA expression of TLR2, TLR3, and TLR4 in peritoneal leukocytes compared with male mice and rats, as well as higher TLR2 and TLR4 expression in macrophages. Ovarian removal, a surgical procedure that quickly ablates cycling estrogen production, significantly reduces leukocyte mRNA expression of TLRs and protein expression of TLR2 and TLR4 on female resident macrophages [117, 118]. These data suggest that sex could be an important factor modulating the effects of physical activity on TLRs expression and activation, although this hypothesis remains untested to this date.
In the brain, the expression of TLRs has been demonstrated in all major glial cell types, including microglia, and neural stem cells [119–123]. TLRs play an important role in brain health, neuroplasticity and cognitive function [124–127]. Our knowledge of human responses in TLR signaling in the brain is limited, but studies using animal models show that exercise can inhibit microglial activation by regulating TLR signaling pathways, particularly in the context of neurological disease. Studies in the field of Parkinson’s disease have shown that soluble α-synuclein indirectly leads to oxidative stress when binding to surface receptors TLR2, TLR4, and CD11b in microglia, resulting in activation of neuroinflammation [128–130]. Interestingly, long-term treadmill running suppressed α-synuclein / TLR2 mediated neuroinflammation and the associated microglia and NADPH oxidase activation [131]. In another study, long-term treadmill running was found to decrease hippocampal neuroinflammation induced by high-fat-diet, including the degree of microglial activation, levels of proinflammatory cytokines (TNF-α and IL-1β) and cyclooxygenase-2, as well as the expression levels of TLR4 and its downstream proteins [132]. In both of these studies, the authors attributed the exercise-induced anti-microglial activation to downregulation of TLR signaling pathways. However, the actual causal relationship between TLRs and microglial activation, as well as the role of sex and exercise in this process remains unconfirmed.
TLR2, TLR4, and TLR8 are also expressed in neural stem cells and play a role in adult hippocampal neurogenesis and learning and memory [120, 133, 134]. Interestingly, the role of TLR4 on adult hippocampal neurogenesis is sex dependent, where female mice deficient of TLR4 showed increased hippocampal neurogenesis [135]. In agreement with this finding, pharmacological inhibition of TLR4 via TLR4 antagonist (TAK-242) enhances memory in young female mice only [133]. However, contradictory evidence shows enhanced spatial memory in young male TLR4 KO mice only and that this sex bias reverses in aged mice [136, 137], suggesting that the role of TLR4 is highly complex and responsive to both sex and age-associated effects. It has been hypothesized that sexually dimorphic response to TLR4 inhibition may relate to sex hormones. Previous work has shown that estrogens have immunomodulatory effects on TLR4 signaling. For instance, estrogens modulate surface expression of TLR4 on macrophages and microglia [58, 138]. Further, recent work by Bonet et al., 2021 [139] showed that estrogens mediated the sex-dependent response to a TLR4 antagonist in a pain model. Specifically, males and females showed the same response to a TLR4 antagonist if females were ovariectomized, however differential effects were seen between the sexes if females were gonad-intact or if 17β-estradiol replacement was given [139].
Thus, accumulating evidence favors a sex-differential expression and activation pathways of TLRs in cells from the periphery and the central nervous system. Yet, very few studies have directly examined whether physical activity alters TLRs expression and function differently in the brain and periphery of female and male brains. Given the powerful anti-inflammatory effects of exercise, additional research into the underlying responses of TLRs to exercise may uncover important new targets to modulate inflammatory responses during brain aging and neurodegenerative diseases.
DISCUSSION
Physical activity is an effective strategy to improve brain health and its function in men and women and in male and female animal models. The U.S. National Institutes of Health (NIH) implemented their Sex as a Biological Variable policy in 2016, with the ultimate goal to improve the value and applicability of biomedical science. Sex- and gender-aware investigations are critical for the advancement of personalized medicine [140], continue to become a key question in all aspects of biological research, and will be particularly relevant in the development of exercise-associated therapeutics.
To date, the field of exercise physiology and exercise medicine has remained male centric. For example, a recent meta-analysis of 50 separate studies performing transcriptomic changes of human skeletal muscle adaptations to exercise and inactivity (Metamex, the most extensive dataset to date) [141], reveals only eight studies with transcriptional profiles from women volunteers. Of these, four profiled healthy controls and four focused on women volunteers with metabolic syndrome. To date, there is no information available on women with diagnosis of MCI, dementia, or frank neurodegenerative disease that participate in these clinical trials. Given the complexity of age-associated immune, metabolic, hormonal and disease-associated changes that affect skeletal muscle physiology and potentially contribute to the onset of age-associated neurodegenerative diseases, this dearth of information on women/female subjects is a critical need for the field.
It is important to note that sex differences are almost always just populational, statistical phenomena –on the average we can see sex differences, but there is a tremendous overlap in the two distributions, so that one could never, for example, determine someone’s sex by just knowing one of these measures. Thus, identifying whether any particular sex difference extends the average overlap between distributions is worth investigating. These fundamental unknowns can only be advanced by actively including women and female animal models in research. This review summarizes various individual findings that suggest that the immune response to exercise might be potentiated in females, but additional studies are needed to create an integrated picture of the intersectional nature of this phenomenon.
In this regard, the on-going Molecular Transducers of Physical Activity Consortium was established to generate a molecular map of exercise using preclinical and clinical studies to examine the systemic effects of endurance and resistance exercise across a range of ages and fitness levels [46]. Although still on-going, the Consortium aims to recruit and enroll sex and age-balanced participants, and they propose to investigate sex differences using multi-omic approaches across multiple tissues in both the acute exercise response and the training response [46]. Generation of this sex, age (and potentially race/ethnicity) driven molecular exercise signatures will yield fascinating insights into the variability of responses to exercise, and potentially help clarify some of the seemingly contradictory reports to date. Integration of responses across tissues in the same individual will also reveal currently unknown nodes of signaling activated by exercise. Cross-species comparisons between the clinical (humans) and pre-clinical (rats) arms will also facilitate the construction of a predictive model of the effects of exercise on central parameters of human health and disease.
Future work in this space should aim to identify how circulatory factors released during exercise affect the brain in females and males, including cognitive and brain health, increases in neurotrophins, changes in synaptic connections in a number of brain regions, vascular support, and the number of new neurons in the hippocampus (Fig. 1). This cross-discipline approach, integrating neuroscience, exercise physiology and geroscience represents a unique opportunity to both uncover new biological paradigms to explain sex-differences in cognitive aging and age-associated neurodegenerative diseases, and to develop novel therapeutic targets against this ever-increasing disease burden in our aging populations.
ACKNOWLEDGMENT
None.
FUNDING
Authors have no funding to report.
CONFLICT OF INTEREST
The authors have no conflict of interest to report.
FINANCIAL DISCLOSURE
The authors declare no competing financial interests.
REFERENCES
[1] | RitchieSJ, CoxSR, ShenX, LombardoMV, ReusLM, AllozaC, et al. Sex Differences in the Adult Human Brain: Evidence from 5216 UK Biobank Participants. Cereb Cortex. (2018) ;28: (8):2959–75. |
[2] | Fang H , Deng X , Disteche CM , X-factors in human disease: impact of gene content and dosage regulation, Human Molecular Genetics (2021) ;30: (R2):R285–r95. |
[3] | Mauvais-Jarvis F , Bairey Merz N , Barnes PJ , Brinton RD , Carrero JJ , DeMeo DL , et al., Sex and gender: modifiers of health, disease, and medicine, Lancet (London, England) (2020) ;396: :(10250)565–82. |
[4] | Nguyen DK , Disteche CM , High expression of the mammalian X chromosome in brain, Brain Research (2006) ;1126: (1):46–9. |
[5] | Westergaard D , Moseley P , Sørup FKH , Baldi P , Brunak S , Population-wide analysis of differences in disease progression patterns in men and women, Nature Communications (2019) ;10: (1):666. |
[6] | Lopez-LeeC, KodamaL, GanL. Sex Differences in Neurodegeneration: The Role of the Immune System in Humans. Biological Psychiatry. (2022) ;91: (1):72–80. |
[7] | Mosconi L , Berti V , Quinn C , McHugh P , Petrongolo G , Varsavsky I , et al., Sex differences in Alzheimer risk: Brain imaging of endocrine vs chronologic aging, Neurology (2017) ;89: (13):1382–90. |
[8] | Lapane KL , Gambassi G , Landi F , Sgadari A , Mor V , Bernabei R , Gender differences in predictors of mortality in nursing home residents with AD, Neurology (2001) ;56: (5):650–4. |
[9] | Sinforiani E , Citterio A , Zucchella C , Bono G , Corbetta S , Merlo P , et al., Impact of Gender Differences on the Outcome of Alzheimer’s Disease, Dementia and Geriatric Cognitive Disorders (2010) ;30: (2):147–54. |
[10] | Ueki A , Shinjo H , Shimode H , Nakajima T , Morita Y , Factors associated with mortality in patients with early-onset Alzheimer’s disease: a five-year longitudinal study, International Journal of Geriatric Psychiatry (2001) ;16: (8):810–5. |
[11] | Lin SJ , Lam J , Beveridge S , Vavasour I , Traboulsee A , Li DKB , et al., Cognitive Performance in Subjects With Multiple Sclerosis Is Robustly Influenced by Gender in Canonical-Correlation Analysis, The Journal of Neuropsychiatry and Clinical Neurosciences (2017) ;29: (2):119–27. |
[12] | Schoonheim MM , Popescu V , Rueda Lopes FC , Wiebenga OT , Vrenken H , Douw L , et al., Subcortical atrophy and cognition: sex effects in multiple sclerosis, Neurology (2012) ;79: (17):1754–61. |
[13] | Voskuhl RR , Gold SM , Sex-related factors in multiple sclerosis susceptibility and progression, Nature Reviews Neurology (2012) ;8: (5):255–63. |
[14] | Reekes TH , Higginson CI , Ledbetter CR , Sathivadivel N , Zweig RM , Disbrow EA , Sex specific cognitive differences in Parkinson disease, npj Parkinson’s Disease (2020) ;6: (1):7. |
[15] | Haaxma CA , Bloem BR , Borm GF , Oyen WJ , Leenders KL , Eshuis S , et al., Gender differences in Parkinson’s disease, Journal of Neurology, Neurosurgery, and Psychiatry (2007) ;78: (8):819–24. |
[16] | Lucca U , Tettamanti M , Tiraboschi P , Logroscino G , Landi C , Sacco L , et al., Incidence of dementia in the oldest-old and its relationship with age: The Monzino 80-plus population-based study, Alzheimers Dement (2020) ;16: (3):472–81. |
[17] | McCombe PA , Henderson RD , Effects of gender in amyotrophic lateral sclerosis, Gender Medicine (2010) ;7: (6):557–70. |
[18] | Shi L , Zhang Z , Su B , Sex Biased Gene Expression Profiling of Human Brains at Major Developmental Stages, Scientific Reports (2016) ;6: :21181. |
[19] | Wyss-Coray T , Mucke L , Inflammation in neurodegenerative disease–a double-edged sword, Neuron (2002) ;35: (3):419–32. |
[20] | Perry VH , Newman TA , Cunningham C , The impact of systemic infection on the progression of neurodegenerative disease, Nature Reviews Neuroscience (2003) ;4: (2):103–12. |
[21] | Perry VH , Nicoll JA , Holmes C , Microglia in neurodegenerative disease, Nature reviews Neurology (2010) ;6: (4):193–201. |
[22] | Posillico CK , Garcia-Hernandez RE , Tronson NC , Sex differences and similarities in the neuroimmune response to central administration of poly I:C, J Neuroinflammation (2021) ;18: (1):193. |
[23] | Orgeta V , Mukadam N , Sommerlad A , Livingston G , The Lancet Commission on Dementia Prevention, Intervention, and Care: a call for action, Irish Journal of Psychological Medicine (2019) ;36: (2):85–8. |
[24] | Hansson O , Svensson M , Gustavsson AM , Andersson E , Yang Y , Nagga K , et al., Midlife physical activity is associated with lower incidence of vascular dementia but not Alzheimer’s disease, Alzheimers Res Ther (2019) ;11: (1):87. |
[25] | Costello JT , Bieuzen F , Bleakley CM , Where are all the female participants in Sports and Exercise Medicine research? European Journal of Sport Science (2014) ;14: (8):847–51. |
[26] | Barha CK , Hsu C-L , ten Brinke L , Liu-Ambrose T , Biological Sex: A Potential Moderator of Physical Activity Efficacy on Brain Health, Frontiers in Aging Neuroscience (2019) ;11: (329). |
[27] | Barha CK , Liu-Ambrose T , Exercise and the Aging Brain: Considerations for Sex Differences. Brain plasticity (Amsterdam, Netherlands) (2018) ;4: (1):53–63. |
[28] | Loprinzi PD , Frith E , The Role of Sex in Memory Function: Considerations and Recommendations in the Context of Exercise, Journal of Clinical Medicine. (2018) ;7: (6). |
[29] | Hamer M , Chida Y , Physical activity and risk of neurodegenerative disease: a systematic review of prospective evidence, Psychological Medicine (2009) ;39: (1):3–11. |
[30] | Buchman AS , Boyle PA , Yu L , Shah RC , Wilson RS , Bennett DA , Total daily physical activity and the risk of AD and cognitive decline in older adults, Neurology (2012) ;78: (17):1323–9. |
[31] | Roig M , Nordbrandt S , Geertsen SS , Nielsen JB , The effects of cardiovascular exercise on human memory: a review with meta-analysis, Neurosci Biobehav Rev (2013) ;37: (8):1645–66. |
[32] | Sanders LMJ , Hortobagyi T , la Bastide-van Gemert S , van der Zee EA , van Heuvelen MJG , Dose-response relationship between exercise and cognitive function in older adults with and without cognitive impairment: A systematic review and meta-analysis, PloS One (2019) ;14: (1):e0210036. |
[33] | Xue Y , Yang Y , Huang T , Effects of chronic exercise interventions on executive function among children and adolescents: a systematic review with meta-analysis, Br J Sports Med (2019) ;53: (22):1397–404. |
[34] | Northey JM , Cherbuin N , Pumpa KL , Smee DJ , Rattray B , Exercise interventions for cognitive function in adults older than a systematic review with meta-analysis, Br J Sports Med (2018) ;52: (3):154–60. |
[35] | Aghjayan SL , Lesnovskaya A , Esteban-Cornejo I , Peven JC , Stillman CM , Erickson KI , Aerobic exercise, cardiorespiratory fitness, and the human hippocampus, Hippocampus (2021) ;31: (8):817–44. |
[36] | Groot C , Hooghiemstra AM , Raijmakers PG , van Berckel BN , Scheltens P , Scherder EJ , et al., The effect of physical activity on cognitive function in patients with dementia: A meta-analysis of randomized control trials, Ageing Res Rev (2016) ;25: :13–23. |
[37] | Carone M , Asgharian M , Jewell NP , Estimating the lifetime risk of dementia in the Canadian elderly population using cross-sectional cohort survival data, J Am Stat Assoc (2014) ;109: (505):24–35. |
[38] | Ludyga S , Gerber M , Puhse U , Looser VN , Kamijo K , Systematic review and meta-analysis investigating moderators of long-term effects of exercise on cognition in healthy individuals, Nat Hum Behav (2020) ;4: (6):603–12. |
[39] | Baker LD , Frank LL , Foster-Schubert K , Green PS , Wilkinson CW , McTiernan A , et al., Effects of aerobic exercise on mild cognitive impairment: a controlled trial, Archives of Neurology (2010) ;67: (1):71–9. |
[40] | Sofi F , Valecchi D , Bacci D , Abbate R , Gensini GF , Casini A , et al., Physical activity and risk of cognitive decline: a meta-analysis of prospective studies, Journal of Internal Medicine (2011) ;269: (1):107–17. |
[41] | Barha CK , Davis JC , Falck RS , Nagamatsu LS , Liu-Ambrose T , Sex differences in exercise efficacy to improve cognition: A systematic review and meta-analysis of randomized controlled trials in older humans, Frontiers in Neuroendocrinology (2017) ;46: :71–85. |
[42] | Barha CK , Falck RS , Davis JC , Nagamatsu LS , Liu-Ambrose T , Sex differences in aerobic exercise efficacy to improve cognition: A systematic review and meta-analysis of studies in older rodents, Front Neuroendocrinol (2017) ;46: :86–105. |
[43] | Varma VR , Chuang YF , Harris GC , Tan EJ , Carlson MC , Low-intensity daily walking activity is associated with hippocampal volume in older adults, Hippocampus (2015) ;25: (5):605–15. |
[44] | Lindberg O , Mårtensson G , Stomrud E , Palmqvist S , Wahlund L-O , Westman E , et al., Atrophy of the Posterior Subiculum Is Associated with Memory Impairment, Tau- and Aβ Pathology in Non-demented Individuals, Frontiers in Aging Neuroscience (2017) ;9: (306). |
[45] | Barha CK , Best JR , Rosano C , Yaffe K , Catov JM , Liu-Ambrose T , Sex-Specific Relationship Between Long-Term Maintenance of Physical Activity and Cognition in the Health ABC Study: Potential Role of Hippocampal and Dorsolateral Prefrontal Cortex Volume, The Journals of Gerontology Series A, Biological Sciences and Medical Sciences (2020) ;75: (4):764–70. |
[46] | Sanford JA , Nogiec CD , Lindholm ME , Adkins JN , Amar D , Dasari S , et al., Molecular Transducers of Physical Activity Consortium (MoTrPAC): Mapping the Dynamic Responses to Exercise, Cell (2020) ;181: (7):1464–74. |
[47] | Kaplan MS , Newsom JT , McFarland BH , Lu L , Demographic and psychosocial correlates of physical activity in late life, American Journal of Preventive Medicine (2001) ;21: (4):306–12. |
[48] | Lee YS , Gender differences in physical activity and walking among older adults, Journal of Women & Aging (2005) ;17: (1-2):55–70. |
[49] | Gleeson M , Bishop NC , Stensel DJ , Lindley MR , Mastana SS , Nimmo MA , The anti-inflammatory effects of exercise: mechanisms and implications for the prevention and treatment of disease, Nature Reviews Immunology (2011) ;11: (9):607–15. |
[50] | Pedersen BK , Febbraio MA , Muscle as an endocrine organ: focus on muscle-derived interleukin-6, Physiological Reviews (2008) ;88: (4):1379–406. |
[51] | Safdar A , Tarnopolsky MA , Exosomes as Mediators of the Systemic Adaptations to Endurance Exercise, Cold Spring Harbor Perspectives in Medicine (2018) ;8: (3). |
[52] | Gleeson M , McFarlin B , Flynn M , Exercise and Toll-like receptors, Exercise Immunology Review (2006) ;12: , 34–53. |
[53] | Timmerman KL , Flynn MG , Coen PM , Markofski MM , Pence BD , Exercise training-induced lowering of inflammatory (CD14+CD16+) monocytes: a role in the anti-inflammatory influence of exercise? Journal of Leukocyte Biology (2008) ;84: (5):1271–8. |
[54] | Yeh SH , Chuang H , Lin LW , Hsiao CY , Eng HL , Regular tai chi chuan exercise enhances functional mobility and CD4CD25 regulatory T cells, British Journal of Sports Medicine (2006) ;40: (3):239–43. |
[55] | Wang J , Song H , Tang X , Yang Y , Vieira VJ , Niu Y , et al., Effect of exercise training intensity on murine T-regulatory cells and vaccination response, Scandinavian Journal of Medicine & Science in Sports (2012) ;22: (5):643–52. |
[56] | Klein SL , Flanagan KL , Sex differences in immune responses, Nature Reviews Immunology (2016) ;16: (10):626–38. |
[57] | Schurz H , Salie M , Tromp G , Hoal EG , Kinnear CJ , Möller M , The X chromosome and sex-specific effects in infectious disease susceptibility, Human Genomics (2019) ;13: (1):2;. |
[58] | Rettew JA , Huet YM , Marriott I , Estrogens augment cell surface TLR4 expression on murine macrophages and regulate sepsis susceptibility in vivo , Endocrinology (2009) ;150: (8):3877–84. |
[59] | Safdar A , Saleem A , Tarnopolsky MA , The potential of endurance exercise-derived exosomes to treat metabolic diseases, Nature Reviews Endocrinology (2016) ;12: (9):504–17. |
[60] | Fiuza-Luces C , Garatachea N , Berger NA , Lucia A , Exercise is the real polypill, Physiology (Bethesda, Md) (2013) ;28: (5):330–58. |
[61] | Hawley JA , Hargreaves M , Joyner MJ , Zierath JR , Integrative biology of exercise, Cell (2014) ;159: (4):738–49. |
[62] | Islam MR , Valaris S , Young MF , Haley EB , Luo R , Bond SF , et al., Exercise hormone irisin is a critical regulator of cognitive function, Nature Metabolism (2021) ;3: (8):1058–70. |
[63] | Moon HY , Becke A , Berron D , Becker B , Sah N , Benoni G , et al., Running-Induced Systemic Cathepsin B Secretion Is Associated with Memory Function, Cell Metabolism (2016) ;24: (2):332–40. |
[64] | Trejo JL , Carro E , Torres-Aleman I , Circulating insulin-like growth factor I mediates exercise-induced increases in the number of new neurons in the adult hippocampus, The Journal of Neuroscience: The Official Journal of the Society for Neuroscience (2001) ;21: (5):1628–34. |
[65] | Fabel K , Fabel K , Tam B , Kaufer D , Baiker A , Simmons N , et al., VEGF is necessary for exercise-induced adult hippocampal neurogenesis, The European Journal of Neuroscience (2003) ;18: (10):2803–12. |
[66] | El Hayek L , Khalifeh M , Zibara V , Abi Assaad R , Emmanuel N , Karnib N , et al., Lactate Mediates the Effects of Exercise on Learning and Memory through SIRT1-Dependent Activation of Hippocampal Brain-Derived Neurotrophic Factor (BDNF), The Journal of Neuroscience: The Official Journal of the Society for Neuroscience (2019) ;39: (13):2369–82. |
[67] | Ferreira JC , Rolim NP , Bartholomeu JB , Gobatto CA , Kokubun E , Brum PC , Maximal lactate steady state in running mice: effect of exercise training, Clinical and Experimental Pharmacology & Physiology (2007) ;34: (8):760–5. |
[68] | De Miguel Z , Khoury N , Betley MJ , Lehallier B , Willoughby D , Olsson N , et al., Exercise plasma boosts memory and dampens brain inflammation via clusterin, Nature (2021) ;600: (7889):494–9. |
[69] | Horowitz AM , Fan X , Bieri G , Smith LK , Sanchez-Diaz CI , Schroer AB , et al., Blood factors transfer beneficial effects of exercise on neurogenesis and cognition to the aged brain, Science (New York, NY) (2020) ;369: (6500):167–73. |
[70] | Leiter O , Seidemann S , Overall RW , Ramasz B , Rund N , Schallenberg S , et al., Exercise-Induced Activated Platelets Increase Adult Hippocampal Precursor Proliferation and Promote Neuronal Differentiation, Stem Cell Reports (2019) ;12: (4):667–79. |
[71] | Mathur N , Pedersen BK , Exercise as a mean to control low-grade systemic inflammation, Mediators of Inflammation. (2008) ;2008: :109502. |
[72] | Hoffmann C , Weigert C , Skeletal Muscle as an Endocrine Organ: The Role of Myokines in Exercise Adaptations, Cold Spring Harbor Perspectives in Medicine (2017) ;7: (11). |
[73] | Pedersen BK , Akerström TC , Nielsen AR , Fischer CP , Role of myokines in exercise and metabolism, Journal of Applied Physiology (Bethesda, Md) (2007) ;103: (3):1093–8. |
[74] | Steensberg A , Febbraio MA , Osada T , Schjerling P , van Hall G , Saltin B , et al., Interleukin-6 production in contracting human skeletal muscle is influenced by pre-exercise muscle glycogen content, J Physiol (2001) ;537: (Pt 2):633–9. |
[75] | Steensberg A , Keller C , Starkie RL , Osada T , Febbraio MA , Pedersen BK , IL-6 and TNF-alpha expression in, and release from, contracting human skeletal muscle, Am J Physiol Endocrinol Metab (2002) ;283: (6):E1272–8. |
[76] | Fischer CP , Interleukin-6 in acute exercise and training: what is the biological relevance? Exercise Immunology Review (2006) ;12: :6–33. |
[77] | Pedersen BK , Steensberg A , Fischer C , Keller C , Keller P , Plomgaard P , et al., Searching for the exercise factor: is IL-6 a candidate? J Muscle Res Cell Motil (2003) ;24: (2-3):113–9. |
[78] | Rothaug M , Becker-Pauly C , Rose-John S , The role of interleukin-6 signaling in nervous tissue, Biochimica et Biophysica Acta (2016) ;1863: (6 Pt A)1218–27. |
[79] | Rose-John S , Heinrich PC , Soluble receptors for cytokines and growth factors: generation and biological function, Biochemical Journal (1994) ;300: (2):281–90. |
[80] | Monje ML , Toda H , Palmer TD , Inflammatory blockade restores adult hippocampal neurogenesis, Science (New York, NY) (2003) ;302: (5651)1760–5. |
[81] | Nakanishi M , Niidome T , Matsuda S , Akaike A , Kihara T , Sugimoto H , Microglia-derived interleukin-6 and leukaemia inhibitory factor promote astrocytic differentiation of neural stem/progenitor cells, The European Journal of Neuroscience (2007) ;25: (3):649–58. |
[82] | Oh J , McCloskey MA , Blong CC , Bendickson L , Nilsen-Hamilton M , Sakaguchi DS , Astrocyte-derived interleukin-6 promotes specific neuronal differentiation of neural progenitor cells from adult hippocampus, Journal of Neuroscience Research (2010) ;88: (13):2798–809. |
[83] | Benini R , Nunes PRP , Orsatti CL , Portari GV , Orsatti FL , Influence of sex on cytokines, heat shock protein and oxidative stress markers in response to an acute total body resistance exercise protocol, Journal of Exercise Science and Fitness (2015) ;13: (1):1–7. |
[84] | Abbasi A , de Paula Vieira R , Bischof F , Walter M , Movassaghi M , Berchtold NC , et al., Sex-specific variation in signaling pathways and gene expression patterns in human leukocytes in response to endotoxin and exercise, Journal of Neuroinflammation (2016) ;13: (1):289. |
[85] | Casaletto KB , Lindbergh C , Memel M , Staffaroni A , Elahi F , Weiner-Light S , et al., Sexual dimorphism of physical activity on cognitive aging: Role of immune functioning, Brain, Behavior, and Immunity (2020) ;88: :699–710. |
[86] | Bostrom P , Wu J , Jedrychowski MP , Korde A , Ye L , Lo JC , et al., A PGC1-alpha-dependent myokine that drives brown-fat-like development of white fat and thermogenesis, Nature (2012) ;481: (7382)463–8. |
[87] | Zügel M , Qiu S , Laszlo R , Bosnyák E , Weigt C , Müller D , et al., The role of sex, adiposity, and gonadectomy in the regulation of irisin secretion, Endocrine (2016) ;54: (1):101–10. |
[88] | Fatouros IG , Is irisin the new player in exercise-induced adaptations or not? A update, Clinical Chemistry and Laboratory Medicine (CCLM) (2018) ;56: (4):525–48. |
[89] | Singhal V , Lawson EA , Ackerman KE , Fazeli PK , Clarke H , Lee H , et al., Irisin levels are lower in young amenorrheic athletes compared with eumenorrheic athletes and non-athletes and are associated with bone density and strength estimates, PloS one (2014) ;9: (6):e100218. |
[90] | Boström P , Wu J , Jedrychowski MP , Korde A , Ye L , Lo JC , et al., A PGC1-α-dependent myokine that drives brown-fat-like development of white fat and thermogenesis, Nature (2012) ;481: (7382):463–8. |
[91] | Magliulo L , Bondi D , Pini N , Marramiero L , Di Filippo ES , Thewonder exerkines—novel insights: a critical state-of-the-art review. Molecular and Cellular Biochemistry. 2021. |
[92] | Pedersen BK , Febbraio MA , Muscles, exercise and obesity: skeletal muscle as a secretory organ, Nature reviews Endocrinology (2012) ;8: (8):457–65. |
[93] | Wrann C , White J , Salogiannis J , Laznik-Bogoslavski D , Wu J , Ma D , et al.,Exercise induces hippocampal BDNF through a PGC-1alpha/FNDC5 pathway, Cell metabolism 2013;18. |
[94] | Asai K , Hiki N , Mimura Y , Ogawa T , Unou K , Kaminishi M , Gender differences in cytokine secretion by human peripheral blood mononuclear cells: role of estrogen in modulating LPS-induced cytokine secretion in an ex vivo septic model, Shock (2001) ;16: (5):340–3. |
[95] | Moxley G , Posthuma D , Carlson P , Estrada E , Han J , Benson LL , et al., Sexual dimorphism in innate immunity, Arthritis Rheum (2002) ;46: (1):250–8. |
[96] | Torcia MG , Nencioni L , Clemente AM , Civitelli L , Celestino I , Limongi D , et al., Sex differences in the response to viral infections: TLR8 and TLR9 ligand stimulation induce higher IL10 production in males, PloS One (2012) ;7: (6):e39853. |
[97] | Blacker D , Wilcox MA , Laird NM , Rodes L , Horvath SM , Go RC , et al., Alpha-2 macroglobulin is genetically associated with Alzheimer disease, Nature Genetics (1998) ;19: (4):357–60. |
[98] | Contreras O , Rossi FMV , Theret M , Origins, potency, and heterogeneity of skeletal muscle fibro-adipogenic progenitors— time for new definitions, Skeletal Muscle (2021) ;11: (1):16. |
[99] | Rubenstein AB , Smith GR , Raue U , Begue G , Minchev K , Ruf-Zamojski F , et al., Single-cell transcriptional profiles in human skeletal muscle, Scientific Reports (2020) ;10: (1):229. |
[100] | Oliva M , Muñoz-Aguirre M , Kim-Hellmuth S , Wucher V , Gewirtz ADH , Cotter DJ , et al., The impact of sex on gene expression across human tissues, Science (New York, NY) (2020) ;369: (6509)eaba3066. |
[101] | Landen S , Jacques M , Hiam D , Alvarez-Romero J , Harvey NR , Haupt LM , et al., Skeletal muscle methylome and transcriptome integration reveals profound sex differences related to muscle function and substrate metabolism, Clinical Epigenetics (2021) ;13: (1):202. |
[102] | Kaisho T , Akira S , Toll-like receptor function and signaling, The Journal of Allergy and Clinical Immunology (2006) ;117: (5):979–87quiz 88. |
[103] | Leulier F , Lemaitre B , Toll-like receptors–taking an evolutionary approach, Nature reviews Genetics (2008) ;9: (3):165–78. |
[104] | Medzhitov R , Toll-like receptors and innate immunity, Nature Reviews Immunology (2001) ;1: (2):135–45. |
[105] | Kawai T , Akira S , The role of pattern-recognition receptors in innate immunity: update on Toll-like receptors, Nature Immunology (2010) ;11: (5):373–84. |
[106] | Pasare C , Medzhitov R , Toll pathway-dependent blockade of CD4+CD25+T cell-mediated suppression by dendritic cells, Science (New York, NY) (2003) ;299: (5609):1033–6. |
[107] | Yamamoto M , Sato S , Hemmi H , Sanjo H , Uematsu S , Kaisho T , et al., Essential role for TIRAP in activation of the signalling cascade shared by TLR2 and TLR4, Nature(2003) ;420: (6913): 4–9. |
[108] | Oliveira M , Gleeson M , The influence of prolonged cycling on monocyte Toll-like receptor 2 and 4 expression in healthy men, European Journal of Applied Physiology (2010) ;109: (2):251–7. |
[109] | Stewart LK , Flynn MG , Campbell WW , Craig BA , Robinson JP , McFarlin BK , et al., Influence of exercise training and age on CD14+cell-surface expression of toll-like receptor 2 and 4, Brain, Behavior, and Immunity (2005) ;19: (5):389–97. |
[110] | Lancaster GI , Khan Q , Drysdale P , Wallace F , Jeukendrup AE , Drayson MT , et al., The physiological regulation of toll-like receptor expression and function in humans, The Journal of Physiology (2005) ;563: (Pt 3):945–55. |
[111] | Souyris M , Cenac C , Azar P , Daviaud D , Canivet A , Grunenwald S , et al., TLR7 escapes X chromosome inactivation in immune cells, Science Immunology (2018) ;3: (19) |
[112] | Souyris M , Mejía JE , Chaumeil J , Guéry JC , Female predisposition to TLR7-driven autoimmunity: gene dosage and the escape from X chromosome inactivation, Seminars in Immunopathology (2019) ;41: (2):153–64. |
[113] | Berghöfer B , Frommer T , Haley G , Fink L , Bein G , Hackstein H , TLR7 ligands induce higher IFN-alpha production in females, Journal of Immunology (Baltimore, Md (2006) ;177: (4):2088–96. |
[114] | Meier A , Chang JJ , Chan ES , Pollard RB , Sidhu HK , Kulkarni S , et al., Sex differences in the Toll-like receptor-mediated response of plasmacytoid dendritic cells to HIV-1, Nature Medicine (2009) ;15: (8):955–9. |
[115] | Khan N , Summers CW , Helbert MR , Arkwright PD , Effects of age, gender, and immunosuppressive agents on in vivo toll-like receptor pathway responses, Human Immunology (2010) ;71: (4):372–6. |
[116] | Fink AL , Engle K , Ursin RL , Tang WY , Klein SL , Biological sex affects vaccine efficacy and protection against influenza in mice, Proceedings of the National Academy of Sciences of the United States of America (2018) ;115: (49):12477–82. |
[117] | Marriott I , Bost KL , Huet-Hudson YM , Sexual dimorphism in expression of receptors for bacterial lipopolysaccharides in murine macrophages: A possible mechanism for gender-based differences in endotoxic shock susceptibility, Journal of Reproductive Immunology (2006) ;71: (1):12–27. |
[118] | Scotland RS , Stables MJ , Madalli S , Watson P , Gilroy DW , Sex differences in resident immune cell phenotype underlie more efficient acute inflammatory responses in female mice, Blood (2011) ;118: (22):5918–27. |
[119] | Cameron JS , Alexopoulou L , Sloane JA , DiBernardo AB , Ma Y , Kosaras B , et al., Toll-like receptor 3 is a potent negative regulator of axonal growth in mammals, The Journal of Neuroscience: The Official Journal of the Society for Neuroscience (2007) ;27: (47):13033–41. |
[120] | Covacu R , Arvidsson L , Andersson A , Khademi M , Erlandsson-Harris H , Harris RA , et al., TLR activation induces TNF-alpha production from adult neural stem/progenitor cells, Journal of Immunology (Baltimore, Md (2009) ;182: (11):6889–95. |
[121] | Farina C , Krumbholz M , Giese T , Hartmann G , Aloisi F , Meinl E , Preferential expression and function of Toll-like receptor 3 in human astrocytes, Journal of Neuroimmunology (2005) ;159: (1-2):12–9. |
[122] | Kielian T ,Toll-like receptors in central nervous system glial inflammation and homeostasis, Journal of Neuroscience Research (2006) ;83: (5):711–30. |
[123] | Lafon M , Megret F , Lafage M , Prehaud C , The innate immune facet of brain: human neurons express TLR-3 and sense viral dsRNA. Journal of Molecular Neuroscience: MN (2006) ;29: (3):185–94. |
[124] | Cunningham C , Campion S , Lunnon K , Murray CL , Woods JF , Deacon RM , et al., Systemic inflammation induces acute behavioral and cognitive changes and accelerates neurodegenerative disease, Biological Psychiatry (2009) ;65: (4):304–12. |
[125] | Okun E , Griffioen K , Barak B , Roberts NJ , Castro K , Pita MA , et al., Toll-like receptor 3 inhibits memory retention and constrains adult hippocampal neurogenesis, Proceedings of the National Academy of Sciences of the United States of America (2010) ;107: (35):15625–30. |
[126] | Tahara K , Kim HD , Jin JJ , Maxwell JA , Li L , Fukuchi K , Role of toll-like receptor signalling in Abeta uptake and clearance, Brain: A Journal of Neurology (2006) ;129: (Pt 11):3006–19. |
[127] | Tang SC , Arumugam TV , Xu X , Cheng A , Mughal MR , Jo DG , et al., Pivotal role for neuronal Toll-like receptors in ischemic brain injury and functional deficits, Proceedings of the National Academy of Sciences of the United States of America (2007) ;104: (34):13798–803. |
[128] | Kim C , Lee HJ , Masliah E , Lee SJ , Non-cell-autonomous Neurotoxicity of α-synuclein Through Microglial Toll-like Receptor 2, Experimental Neurobiology (2016) ;25: (3):113–9. |
[129] | Rocha EM , De Miranda B , Sanders LH . Alpha-synuclein: Pathology, mitochondrial dysfunction and neuroinflammation in Parkinson’s disease. Neurobiology of Disease (2018) ;109: (Pt B):249–57. |
[130] | Zhang QS , Heng Y , Yuan YH , Chen NH , Pathological α-synuclein exacerbates the progression of Parkinson’s disease through microglial activation, Toxicology Letters (2017) ;265: :30–7. |
[131] | Koo JH , Jang YC , Hwang DJ , Um HS , Lee NH , Jung JH , et al., Treadmill exercise produces neuroprotective effects in a murine model of Parkinson’s disease by regulating the TLR2/MyD88/NF-κB signaling pathway, Neuroscience (2017) ;356: :102–13. |
[132] | Kang EB , Koo JH , Jang YC , Yang CH , Lee Y , Cosio-Lima LM , et al., Neuroprotective Effects of Endurance Exercise Against High-Fat Diet-Induced Hippocampal Neuroinflammation, Journal of Neuroendocrinology (2016) ;28: (5). |
[133] | Connolly MG , Potter OV , Sexton AR , Kohman RA , Effects of Toll-like receptor 4 inhibition on spatial memory and cell proliferation in male and female adult and aged mice, Brain, Behavior, and Immunity (2021) ;97: :383–93. |
[134] | Rolls A , Shechter R , London A , Ziv Y , Ronen A , Levy R , et al., Toll-like receptors modulate adult hippocampal neurogenesis, Nature Cell Biology (2007) ;9: (9):1081–8. |
[135] | Connolly MG , Yost OL , Potter OV , Giedraitis ME , Kohman RA , Toll-like receptor 4 differentially regulates adult hippocampal neurogenesis in an age- and sex-dependent manner, Hippocampus (2020) ;30: (9):958–69. |
[136] | Okun E , Barak B , Saada-Madar R , Rothman SM , Griffioen KJ , Roberts N , et al., Evidence for a developmental role for TLR4 in learning and memory, PloS One (2012) ;7: (10):e47522. |
[137] | Potter OV , Giedraitis ME , Johnson CD , Cox MN , Kohman RA , Young and aged TLR4 deficient mice show sex-dependent enhancements in spatial memory and alterations in interleukin-1 related genes, Brain, Behavior, and Immunity (2019) ;76: :37–47. |
[138] | Zhang Z , Qin P , Deng Y , Ma Z , Guo H , Guo H , et al., The novel estrogenic receptor GPR30 alleviates ischemic injury by inhibiting TLR4-mediated microglial inflammation, Journal of Neuroinflammation (2018) ;15: (1):206. |
[139] | Bonet IJM , Araldi D , Green PG , Levine JD , Sexually Dimorphic Role of Toll-like Receptor 4 (TLR4) in High Molecular Weight Hyaluronan (HMWH)-induced Anti-hyperalgesia, The Journal of Pain (2021) ;22: (10):1273–82. |
[140] | Arnegard ME , Whitten LA , Hunter C , Clayton JA , Sex as a Biological Variable: A 5-Year Progress Report and Call to Action, Journal of Women’s Health (2020) ;29: (6):858–64. |
[141] | Pillon NJ , Gabriel BM , Dollet L , Smith JAB , Sardón Puig L , Botella J , et al., Transcriptomic profiling of skeletal muscle adaptations to exercise and inactivity, Nature Communications (2020) ;11: (1):470. |