Regulation of Adult Neurogenesis by the Fragile X Family of RNA Binding Proteins
Abstract
The fragile X mental retardation protein (FMRP) has an important role in neural development. Functional loss of FMRP in humans leads to fragile X syndrome, and it is the most common monogenetic contributor to intellectual disability and autism. FMRP is part of a larger family of RNA-binding proteins known as FXRs, which also includes fragile X related protein 1 (FXR1P) and fragile X related protein 2 (FXR2P). Despite the similarities of the family members, the functions of FXR1P and FXR2P in human diseases remain unclear. Although most studies focus on FMRP’s role in mature neurons, all three FXRs regulate adult neurogenesis. Extensive studies have demonstrated important roles of adult neurogenesis in neuroplasticity, learning, and cognition. Impaired adult neurogenesis is implicated in neuropsychiatric disorders, neurodegenerative diseases, and neurodevelopmental disorders. Interventions aimed at regulating adult neurogenesis are thus being evaluated as potential therapeutic strategies. Here, we review and discuss the functions of FXRs in adult neurogenesis and their known similarities and differences. Understanding the overlapping regulatory functions of FXRs in adult neurogenesis can give us insights into the adult brain and fragile X syndrome.
INTRODUCTION
Adult neurogenesis is the process by which new neurons are created from neural stem cells (NSCs) residing in the postnatal brain. This process is limited to very specific regions within the adult brain. In humans and other mammals, the subgranular zone (SGZ) of the dentate gyrus (DG) region of the hippocampus is one of only a few brain regions that is capable of generating new neurons throughout the life of the organism [1]. In the DG, NSCs integrate both extrinsic and intrinsic signals to either maintain their quiescent state (qNSC) or to actively divide (aNSC). The aNSCs can mature and give rise to intermediate progenitor cells (IPCs), which subsequently differentiate into glutamatergic DG granule neurons or astrocytes.
Adult neurogenesis includes proliferation, neuronal fate specification, maturation, and functional integration into neuronal circuits. Each step requires input from a variety of pathways and is influenced by many regulators [2]. Adult NSCs can divide symmetrically to create two identical daughter NSCs through a process called self-renewal. Self-renewal is an essential feature of all stem cells and is critical for maintaining limited stem cell pools and for expanding the populations of stem cells when needed. If stem cell maintenance is properly regulated, the NSCs in the adult brain contribute to brain plasticity during normal brain functions and participate in cellular regeneration upon injuries throughout the life of the organism. However, if aberrant NSC self-renewal occurs and NSCs fail to enter the cell cycle or become overly proliferative, it can deplete the NSC pool and cause declines in neurogenesis [3]. Therefore, cell cycle regulation is key for the delicate balance between NSC maintenance and differentiation in adult neurogenesis. Another feature of adult NSCs is neural differentiation. As described below, a majority of adult NSCs differentiate into neurons in vivo. However, reduced neuronal differentiation has been found in a number of pathological conditions [3]. In addition, cell cycle control of adult NSCs directly impacts neural differentiation of adult NSCs.
This review focuses on the fragile X family (FXRs) of RNA-binding proteins. The FXRs include fragile X mental retardation protein (FMRP), fragile X related protein 1 (FXR1P), and fragile X related protein 2 (FXR2P). All three FXRs are enriched in the brain [4]. Although FXRs were thought to mainly regulate synaptic plasticity of mature neurons, recent studies have demonstrated critical roles of FXRs in NSCs and neurogenesis both during development and in adulthood [4]. In this article, we will first give an overview of adult neurogenesis, its functional importance, and its regulation by cellular signaling pathways, especially cell-cycle regulators. Second, we will review the roles of FXRs in regulating adult neurogenesis, particularly their roles in NSC maintenance. Finally, we will discuss both common and unique mechanisms underlying how FXRs regulate adult neurogenesis, with an emphasis on NSC activation, quiescence, and the cell cycle.
THE FRAGILE X PROTEINS
Fragile X mental retardation protein is well-known for its causative role in FXS, the most common genetic cause of intellectual disability and the largest single genetic contributor to autism [5] However, the other two FXRs are overlooked and underappreciated. FXR1P and FXR2P are highly similar to FMRP and can act as regulatory binding partners for FMRP’s RNA targets [6–10]. Structural and biochemical studies have shown that the three FXRs exhibit high homology in their RNA binding domains (Fig. 2) and can form hetero-multimers, suggesting that FXRs have similar abilities in binding mRNAs and regulating protein translation [11–13]. In fact, the only published genome-wide FXR1P binding (PAR-CLIP) analysis, using HA-tagged FXRs expressed in HEK293 cells, show that FXR1P and FMRP share >95% of their targets [14]. However other studies suggest that FXRs may have distinct functions and mechanisms of action [11]. FXR1P and FXR2P are incapable of rescuing FMRP deficiency in the brain [15–17]. Evidence is slowly accumulating about the unique and important roles of the individual FXRs in the brain and elsewhere. For example, FXR1P plays a critical role in development; it is the only FXR that when deleted leads to neonatal lethality [18].
Fragile X syndrome and FMRP
Fragile X syndrome affects about 1 in 4000 males and 1 in 7000 females [19]. Physically, FXS patients are characterized by an elongated face, large ears, loose joints, flat feet, and macroorchidism [19, 20]. Studies of postmortem tissue from FSX individuals have found that neurons in several regions of the cortex have thin, dense, immature spines, indicative of FMRP’s importance in the brain [20]. FXS individuals typically exhibit some form of intellectual disability and 15–60% of FXS patients exhibits autistic traits [19, 20]. Autism is a complex disease with multiple genetic factors, but FXS serves as a genetic model for autism because FMRP is the largest single-gene contributor to autism [19, 20].
Fragile X mental retardation protein was named and identified by the ‘fragile’ site created on the X chromosome (Xq27.3) due to the expansion of a CGG repeat in its 5’ untranslated region (5’UTR). Most people have 30 to 31 CGG repeats in their FMR1 gene, and up to about 45 repeats is considered normal [21]. If the number of CGG repeats is 55 to 200, it is called a premutation, and the repeats tend to expand through meiosis. Individuals who have a premutation have an increased likelihood of developing fragile X–related primary ovarian insufficiency (FXPOI) and fragile X–associated tremor/ataxia syndrome (FXTAS) [22, 23]. Twenty percent of female premutation carriers have fertility issues early in life associated with FXPOI [23]. On the other hand, FXTAS is a neurodegenerative disorder that occurs more prominently in older males [22]. When the number of CGG repeats is longer than 200, the repeats are methylated and the FMR1 gene is silenced, causing FXS [19]. As of yet, we do not fully understand either the mechanism of CGG-repeat expansion or the biological process controlling FMR1 gene silencing. Studying these mechanisms will require the use of human models because A) mouse models for premutation exhibit significantly less germline instability than human individuals with premutations, and B) mouse models do not exhibit CGG-repeat methylation and silencing.
Mouse models of fragile X syndrome exhibit region-specific alterations in hippocampal adult neurogenesis and altered behavioral phenotypes, such as spatial learning and memory [24]. A majority of FXS symptoms are attributed to the regulation of group 1 metabotropic glutamate receptor-dependent long-term depression (mGluR-LTD) [25]. According to the mGluR theory, FMRP represses translation of its mRNA targets in post-synaptic spines until mGluR5 is stimulated and activates local protein synthesis [25]. This synthesis leads to the internalization of AMPA (α-amino-3-hydoxyl-4-isoxazole propionic acid) receptors leading to LTD in neurons. Without FMRP, protein synthesis is constantly heightened, increasing AMPA internalization and enhancing LTD, which impairs synaptic plasticity, and learning and memory in the brain. This discovery has led to a variety of clinical trials; however very few of these have been successful [26]. It is important to not overlook other potential regulators of FMRP targets, such as FXR1P and FXR2P.
In addition to mGluR, FMRP also targets glutamate signaling through the N-methyl-D-aspartate receptor (NMDAR). NMDAR signaling is highly related to adult neurogenesis in the dentate gyrus [27, 28] and dysfunction of NMDAR has been shown in FXS models [29–32]. FMRP associates with the mRNA of NMDAR subunits, including GluN1, GluN2A, and GluN2B [33] and regulates a number of synaptic proteins involved in NMDA receptor signaling [34]. Fmr1 knockout mice show reduced NMDAR-dependent long-term potentiation (LTP) [29] and levels of NMDAR subunits (GluN1, GluN2A, and GluN2B) in the DG. Treatment with the NMDAR co-agonist glycine can rescue impairments in NMDAR-dependent synaptic plasticity in the DG [30]. Female mice with heterozygous loss of the Fmr1 gene showed impairments in a pattern-separation task known to be related to adult neurogenesis in the DG as well as significant impairments in both NMDAR-dependent LTD and LTP [31]. In the hippocampal region as a whole, GluN2B is hyperactive in Fmr1 knockout mice, GluN2B-specific inhibitors can rescue mGluR-LTD, suggesting dysregulated NMDARs leads to altered mGluR-LTD in the FXS mouse model [32]. The molecular mechanism undergoing the alteration of synaptic plasticity in the hippocampus, especially the DG, induced by dysregulated NMDAR signaling remains to be elucidated. However, the NMDAR agonist and NMDAR subunit-inhibitor treatment have been shown to rescue the altered phenotypes in the FXS mouse model and modulating the NMDAR may serve as a potent treatment for learning and memory impairment in FXS patients.
Structure and function of FXRs
Once FMR1 was sequenced, identification of the human FXR1 (3q28) and FXR2 (17p13.1) via sequence homology and yeast two-hybrid screening quickly followed [8, 35]. Unlike FMR1, the FXR1 and FXR2 genes are autosomal, which requires both copies of the gene to be affected to show a deleterious phenotype. That makes FXR1P and FXR2P less likely to cause disease. Similar to FMR1, the FXR2 gene also contains CGG repeats in the 5’UTR; however, no CGG expansion or silencing of FXR2 gene has been observed [36]. The FXR proteins are highly conserved, even to the point of conserving exon size between the mouse and human version of each gene. Each gene contains 17 exons. Exons 11 and 12 of the FMR1 gene are unique, and FXR1 and FXR2 have similar exons 16 and 17 that are not present in FMR1 [36]. The overall conserved gene structure indicates a shared ancestor. Drosophila melanogaster only has one FXR gene, dfmr1, which is missing exons 11 and 12. It is thought that as Fmr1 gene evolved, it acquired additional exons and duplicated to create the other FXR genes. The FXRs have highly similar gene sequences, but their differences indicate they have unique functions.
Proteins in the FXR family are also very similar in structure. (Fig. 2). Each FXR protein contains a nuclear localization sequence (NLS), an N-terminal protein-binding domain (NTD), two hnRNP homology domains (KH) domains, a nuclear export sequence (NES), and an arginine-glycine-glycine box (RGG) (Fig. 2). The NTD is important for protein-protein interactions and is required for associating with the 60S ribosomal subunit [37–39]. Curiously, even though FXRs are RBPs, the NTD contains two agenet domains. Agenet domains are similar to tudor domains in plants and are typically found in chromatin interactors [39–41]. Structurally, this region is similar to UHRF1, which binds methylated H3K9 [41]. This mystery was partially solved when FMRP was found to bind chromatin and DNA damage response (DDR) proteins that are important in spermatogenesis, which may relate to the macroorchidism seen in FXS patients [42]. Preliminary findings suggest that FXR2P is also capable of binding methylated histones [41, 42]. Investigating the association of DDR proteins and FXRs could be an interesting avenue of study in the brain, as other markers of DNA damage are associated with learning and memory in the hippocampus [43].
All FXRs contain an NLS and NES. The NLS enhances RNA affinity and association of between the FXRs [44]. The NLS is also pathologically relevant, as a mutation in Fmr1’s NLS was identified in a male patient with developmental delay [45]. FXR1P and FXR2P also contain a nucleolar targeting signal (NoS) in their C-termini, but the NoS is absent in some FXR1P isoforms [46]. All FXRs shuttle through exportin-1, with FMRP shuttling from the nucleus to the cytoplasm and FXR2P shuttling from the nucleolus, but FXR1P’s shuttling is isoform-dependent [46, 47]. Although these proteins are highly similar, differences in sub-cellular localization and shuttling could indicate different functional roles for FXRs in RNA metabolism. As of yet, none of these proteins has been directly connected to RNA splicing, but it is a functional possibility.
The FXRs were first classified as RBPs due to their KH and RGG domains. The RGG box is important for binding poly(G) in vitro and binds to G quartets, a secondary RNA structure where the four guanines line up in a plane [37, 48, 49]. The ability to bind G quartets varies between the FXRs; besides FMRP, only the longest isoform of FXR1P has this ability [50]. Methylation of the arginine in the RGG domain can alter the affinity of these RNA targets [48]. However, only a minority of FMRP targets contain RGG structure or the pseudoknot known as the kissing complex [51, 52]. High-throughput sequencing of RNA isolated by crosslinking immunoprecipitation (HITS-CLIP) in HEK293 cells shows that most FXR targets contain ACUK or WGGA, where K is G/U and W is A/U [14]. In fact, all the FXRs can bind throughout an mRNA, including exons and 3’UTRs [48]. A more general binding sequence allows the FXRs to bind a large variety of RNA targets. The KH domain was first identified in heterogeneous nuclear ribonucleoprotein K (hnRNP K) and is found in RBPs from bacteria to humans [53]. The KH domain mediates FXRs binding to RNA, and a single amino acid change is enough to cause FXS [54–56]. The KH domain and the NTD are both essential for FXRs to associate with the 60S ribosomal subunit and polyribosomes [9, 37, 38]. FXRs are also capable of hetero- or homo-dimerizing with each other through the KH domains, existing in RNA granules in axons and presynaptic terminals [6, 7, 9, 10, 37]. This implies that they are capable of co-regulating a subset of RNA targets.
Localization and expression patterns
The FXRs are ubiquitously expressed proteins. However, their levels of expression vary greatly between tissues. FXRs have the highest expression in the brain and testes [57–60]. FXR1P is the only FXR that is highly expressed in heart and skeletal muscles [57, 60, 61]. This is indicative of FXR1P’s unique regulatory function in muscle cells and their myogenic precursors [62]. In the human brain, FXRs are most highly expressed in cortical neurons, cerebellar Purkinje neurons, and the brain stem. FXRs are commonly expressed in the same tissues, but subtle expression differences in cell types and cellular compartments throughout development indicate separate targets and functions [57]. Examining neurons at a subcellular level, FXRs are found in dendrites and presynaptic spines [6, 10, 57, 63, 64]. In the cytoplasm, FXRs associate with ribosomes, RNA, and other proteins in ribonuclear particles (RNPs) [37, 65–68]. FXRs are also found presynaptically in axons, specifically in the thalamus, the CA3 region, and the olfactory bulb [6, 10, 64]. In axons, FXRs associate with one another in fragile X granules (FXGs) [10]. FXGs are dependent on FXR2P and disappear if Fxr2 is deleted [10]. The association of different combinations of FXRs could modulate or alter target mRNA expression.
RNA regulation by FXRs
The FXRs can regulate RNAs in several ways. They can affect RNA stability or translational efficiency. More specifically, FMRP is typically thought to inhibit translation initiation or translation elongation. FXRs also have the potential to utilize the microRNA pathway to regulate their targets. Most of these mechanisms were discovered through FMRP’s role in neurons and have not been demonstrated for FXR1P and FXR2P. But as more is learned about the other FXRs, many of these regulatory mechanisms appear to be similar, although their targets may be different.
FMRP regulates translation by two different mechanisms: either by inhibiting translation initiation or by inhibiting elongation. FMRP interacts directly with cytoplasmic FMR1 interacting protein (CYFIP1), which is structurally similar to 4E-BP [69–71]. This protein binds the translation initiation factor, eIF4E, to stop the formation of the translation initiation complex, regulating MAP1B, APP, and αCaMKII protein levels in neurons [69]. The FMRP/CYFIP1 interaction is regulated in an activity-dependent manner, and dissociates when neurons are stimulated with BDNF or DHPG [69]. This is unlikely to be an exact mechanism for FXR1P or FXR2P action, since they bind CYFIP2 and not CYFIP1 [70], although a related mechanism is possible. Elongation stalling is the most accepted mechanism for FMRP’s regulation of translation. This is most likely because of a large-scale HITS-CLIP study on RNA isolated from the whole brain, which found that FMRP binds throughout the open reading frame (ORF) and not just the UTRs [72]. FMRP can associate with stalled polyribosomes when it is phosphorylated, and de-phosphorylation leads to active translation [73]. Phosphorylation of FMRP is activity-dependent in neurons [74]. De-phosphorylation and active translation is required for synaptic pruning [75]. This results in decreased pruning in neurons of Fmr1 knockout mice, which are characterized by dense immature spines [76–81]. Activity-dependent elongation stalling has not been shown for the other FXRs, even though they have many of the characteristics needed for this process. FXR1P and FXR2P both bind throughout mRNAs, associate with polyribosomes, and have the same conserved phosphorylation residue as FMRP [7, 14, 65]. Therefore, it should be examined whether FXR1P and FXR2P utilize the same regulatory mechanisms used by FMRP.
FXRs can also regulate translation or mRNA stability through the microRNA (miR) pathway. All three FXRs can associate with miRs and/or their precursors in human cells via their KH domains [82, 83]. Although an early study in mouse embryonic fibroblasts suggested that FMRP and RNA-induced silencing complex (RISC) targets do not overlap, studies in neurons found that FMRP associates with miRs to affect spine morphology and regulate PSD-95 [84–86]. In an added level of regulation, translational repression of PSD-95 through the miR pathway is enhanced by FMRP phosphorylation, which can be reverted with neuronal stimulation [85]. Independent of FMRP, FXR1P controls miR-9 and miR-124 levels in the embryonic brain [87]. But the details and differences in FMRP and FXR1P targets and mechanisms still need to be explored. In 293 cells, FXR1P and Argonaute 2 (AGO2) associate with AU-rich elements (AREs) for translational activation that induces cell cycle arrest [88]. These proteins can switch from translational activation to repression during the cell cycle in association with miR369-3 or Let-7 [89]. FXR1P isoform a and AGO2 also activate translation to maintain quiescence in Xenopus laevis oocytes [90, 91]. Although p21’s regulation has not been directly connected to the RISC pathway yet, FXR1P does regulate p21 mRNA stability through AREs in its 3’UTR [92, 93]. FXR1P’s regulation of p21 controls proliferation in myoblasts, as well as quiescence in cancer [92, 93]. In addition, there are miRs that inhibit p21 mRNA and promote cell cycle progression [94]. It is possible that FXR1P is an important cell cycle regulator in proliferative cell types, such as NSCs [192]. No further work has been done to examine this regulatory function in the brain.
ADULT NEUROGENESIS
Neurogenesis is the process of creating new neurons. For years, neurogenesis was thought to occur only in the developing brain and not in mature mammals. In 1965, Altman and Das published the first evidence of neurogenesis in the adult brain [95]. They injected 3H-thymidine into adult rats, which was incorporated into newly synthesized DNA. The only cells that can incorporate 3H-thymidine are ones that are pre-mitotic and capable of dividing, such as stem or progenitor cells. After examining the hippocampus two months later, they found labeling in cells with the morphology of mature neurons, suggesting the existence of proliferating precursors that are capable of neuronal differentiation. At the time there was no way to confirm that these cells were neurons. Therefore, these findings were largely ignored until additional studies supported this claim.
After years of controversy, and numerous studies in a variety of species, the concept of adult neurogenesis is largely accepted in the neuroscience field [1]. In mammals, there are two main sites of adult neurogenesis: the subgranular zone of the dentate gyrus of the hippocampus, and the subventricular zone (SVZ) of the lateral ventricles. Each site contains a small population of mainly quiescent adult NSCs. These cells are multi-potent, meaning that they can differentiate into glia and neurons, the main cell types of the brain. During neurogenesis, NSCs divide to produce proliferative intermediates, which differentiate into immature neurons before integrating into the neuronal circuitry. In mice, this process takes 4–8 weeks. Although new neurons are continuously generated, they need to receive the proper signaling both intrinsically and extrinsically to survive.
Neurogenesis in the SVZ supplies new neurons to the olfactory bulb (OB). This process is highly active in rodents that rely on smell for social cues and to find food. The stem cell population of the SVZ is made up of type B cells. These cells cluster together, surrounded by a ‘pinwheel’ of ependymal cells, and project their single cilium into the lateral ventricle [96]. This cilium probably acts as a sensor, detecting environmental cues for proliferation. Type B cells are similar to astrocytes and express several astrocyte proteins, such as glial fibrillary acidic protein (GFAP), glutamate aspartate lipid transporter (GLAST) and brain lipid-binding protein (BLBP) [97–99]. Quiescent type B cells can be isolated using a cell surface marker, CD133 [100], and activated type B cells going through the cell cycle are marked by the intermediate filament, NESTIN [97]. Type B cells can divide asymmetrically and give rise to one type B and one type C daughter cell [101]. However, none of these markers is unique to NSCs. Therefore, one of the challenges of studying adult neurogenesis is the lack of a single unique marker to identify the entire population of NSCs. Type C cells are a population of intermediate progenitor cells (IPCs), also known as transient amplifying progenitors. Type C cells express the transcription factors MASH1 and DLX2. These cells divide symmetrically several times and then produce type A cells, also known as neuroblasts (NBs), which express Doublecortin (DCX) and polysialylated neural-cell-adhesion molecule (PSA-NCAM) [101]. Type A cells migrate from the lateral ventricle to the OB in a long chain ensheathed by glia, in what is known as the rostral migratory stream (RMS) [102, 103]. Despite traveling long distances at a rapid rate, relatively few of these cells migrate off course. Once they reach the OB, these cells disperse radially, and the majority mature into γ-aminobutyric acid (GABA)-expressing GABAergic interneurons.
In the SGZ of the DG, the NSCs are a mostly quiescent population of radial glia-like cells (RGLs), also known as type I cells, that express both NESTIN and GFAP. These cells have a pyramidal cell body that sends a single projection through the granular zone, which terminates in a bushy top for morphogen sensing. In recent years, advancement in clonal analysis and single cell sequencing methods have led to many more discoveries about this small number of NSCs. Clonal analysis has confirmed that RGLs in the adult hippocampus are proliferative and multi-potent [104]. RGLs can either divide symmetrically to produce two RGLs to maintain the stem cell pool or divide asymmetrically to produce RGLs, IPCs, and/or terminally differentiated astrocytes [105–107]. As RGLs reach their final division and transition to astrocytes, their single projection becomes shorter and bushier [104, 107]. Many factors can affect RGL division. For example, seizures can induce RGL activation and their symmetric division into reactive astrocytes leading to depletion of the stem cell pool [106]. Once activated, RGLs can only divide 2-3 times before terminal differentiation into astrocytes, which contributes to the decline of neurogenesis with age [105]. Therefore, NSC activation must be tightly regulated so that a stem cell pool can be maintained for the continuous generation of new neurons into old age.
Intermediate progenitor cells express TBR2 and can proliferate or transition to NBs that express DCX and PSA-NCAM [104]. NBs have limited proliferative ability and can differentiate into immature neurons that are no longer capable of proliferation. Immature neurons require survival signals from their surrounding environment, including other neurons, to mature and integrate into the neuronal network. Bromodeoxyuridine (BrdU) labeling studies have shown that only about half of proliferating cells (NSCs, IPCs, NBs) survive after 4 weeks and a great majority of these cells are neurons [108]. Maturation of new neurons is a long process requiring a variety of signaling inputs as well as changes in morphology and physiology. During the first week after a new neuron is ‘born’ – meaning after it commits to the neuronal lineage and becomes post-mitotic – it migrates a short distance into the granular layer and is tonically activated by GABA [109–111]. In the next week, the dendrite begins to grow and extend toward the molecular layer, while the mossy fiber (axon) grows into the hilus of the DG and extends toward the (carbonic anhydrase 3) CA3 region of the hippocampus [109, 112]. At this point, GABA signals from the local granular cells are important for the new neuron’s survival, as well as the creation of dendritic arbors and synapses [110, 111, 113–115]. In the third week, the new neuron starts to make synapses with its newly formed dendritic spines and integrates into the neuronal network by connecting to CA3 neurons, and also creates local connections [116, 117]. The third week is also when GABA switches from being an activating to an inhibitory signal and glutamatergic signals become key for synaptic integration and survival [110, 111]. Neurons that are 2-3 weeks old are highly excitable, having both high resting potentials and membrane resistance [110, 118, 119]. At 4–6 weeks, these neurons have a lower activation threshold for long-term potentiation (LTP) and give higher LTP signals than mature neurons, translating into stronger synaptic plasticity [118]. Once neurons are 8 weeks old, they have mostly finished their complex development and may be indistinguishable from mature neurons.
Adult neurogenesis in humans
Adult neurogenesis in rodents was first discovered over fifty years ago, but evidence of adult human neurogenesis has been considerably more difficult to demonstrate [95]. The first direct evidence came from examining the brains of cancer patients who had previously been injected with the thymidine analog, BrdU, during adulthood [120]. These patients had sparse BrdU labeling in their hippocampal but not cortical neurons, demonstrating the neurogenic potential of the adult DG. Additional evidence came from the study of healthy post-mortem tissue using progenitor and immature neuron markers. It has been shown that the brains of humans from birth to age 100 can be labeled with stem cell markers Sex Determining Region Y-Box 2 (SOX2) and NESTIN, but the frequency of positive labeling declines with age [121]. Most studies of human neurogenesis used markers for cell proliferation, such as Ki67, MCM2 (minichromosome maintenance complex component 2), PCNA (proliferating cell nuclear antigen), and markers for neuroblasts and immature neurons (DCX, PSA-NCAM) [121, 122]. The numbers of these immature cells decrease dramatically during the first year of life and then further decline fivefold from age 20 to 100 [123]. This is consistent with observations that the total number of hippocampal neurons decline with age, but the decline is less pronounced in the DG region, most likely due to its neurogenic capacity [124–128].
In the SVZ, evidence of adult neurogenesis is relatively weak. Initial work identified GFAP-positive cells lining the lateral ventricle that could be cultured as neurospheres and would differentiate into neurons, astrocytes, and oligodendrocytes [129]. But this group could not identify any migrating NBs using classical markers. Following this work, another group identified a hollow tube of PCNA-positive cells extending from the lateral ventricle to the olfactory bulb [130]. Since then, however, demonstration of an RMS-like structure has only been successful in fetal and infant tissues, where short chains of DCX and PSA-NCAM-positive cells migrate to the ventromedial prefrontal cortex [131, 132]. DCX and proliferation markers appear to drop off after birth, with very few cells seen after 8 months of age [131]. It is possible that a small number of NBs could have a larger effect in humans or that this difference may stem from the greater importance of the olfactory system in rodents versus humans. Humans have one-third the number of olfactory receptors that mice do, and yet have three times the amount of glomeruli in their olfactory bulbs [133]. This disparity, coupled with the social and food-gathering importance of smell in mice, supports the organizational and neurogenic differences seen between the two species.
Recently, a new method providing direct evidence for human adult neurogenesis was developed. This technique, called cell birth dating, is based on the radiocarbon-dating method used predominantly in paleontology to date dinosaur bones and other artifacts. Historically, C14 levels have been extremely low in the atmosphere. During the Cold War, with above ground nuclear testing, C14 levels spiked until the nuclear armistice and have been declining ever since [134]. Carbon dioxide in the atmosphere contains C14 and is taken up by plants during photosynthesis and by humans consuming plants. Once it enters the human body, C14 is stably incorporated into DNA. This method of carbon dating compares the level of C14 in cells to known yearly levels of C14 in the atmosphere to determine the year a cell was born. It was found that hippocampal DG neurons exhibit significant turnover after birth, providing the second piece of direct evidence for adult neurogenesis since the Eriksson study in 1998 [120, 135]. As controls, cortical neurons were found to have birthdates similar to their subjects, whereas intestinal cells with fast turnover rates were found to have birthdates close to the year of the subjects’ deaths [135]. Using computer simulations, it is estimated that 1.75% of DG neurons are replaced every year, implying that 700 new neurons are created time.
Considering the large number of new DG neurons generated daily and the importance of adult neurogenesis in learning and memory, it is not surprising that this process is affected in several neuropsychological and neurodevelopmental diseases. Patients with major depressive disorders (MDD), schizophrenia, addiction, or anxiety disorders exhibit decreased hippocampal volumes [3, 136]. MDD patients who received treatment with antidepressants have larger DG and granular cell layer volumes [137]. Hippocampal volumes are also smaller in patients with memory disorders [138–144]. Further targeted molecular studies in mouse models have lent support for disease-related proteins causing changes in adult neurogenesis [145]. These include disease genes for intellectual disabilities such as Rett syndrome and fragile X syndrome (FXS) [1, 145]. Better understanding the changes in adult neurogenesis could have promising implications for disease treatments as well as understanding learning and memory.
Rodent models are essential for determining the molecular mechanisms of adult neurogenesis. Further studies in human patients are also needed to fully understand the impact of neurological disease on adult neurogenesis. This requires advances in imaging techniques to examine neurogenesis in living patients. Measurement of hippocampal volume is a crude estimate of neurogenesis and could be indicative of other changes, including neuronal soma size, dendritic changes, or changes in glia [146]. Besides magnetic resonance imaging (MRI), which is used for volume measurements, there are two other technologies used to visualize adult neurogenesis. Magnetic resonance spectroscopy (MRS) detects neurogenic-specific metabolites that decline with age [147]. Based on the close association of neurogenesis and angiogenesis, measuring cerebral blood volume (CBV) is also used to map exercise-induced neurogenesis [148]. All these methods, however, only offer indirect support for human neurogenesis. We are still lacking an independent method to validate these findings [149]. At this point, rodent models continue to be our best option for a detailed examination of adult neurogenesis.
Functional importance of adult neurogenesis
New neurons are hyper-excitable and highly plastic, making them perfect for learning and creating new memories. When newborn neurons are 4-6 weeks old they have the greatest impact on memory [150]. Adult neurogenesis was first associated with behavior in canaries, which create new neurons as they learn new songs [151, 152]. Additional neurogenesis-associated behaviors were learned from hippocampal lesions and neurogenesis ablation studies in rodents [153]. Initially, neurogenesis-associated behaviors were difficult to identify. The behavioral tests needed to be properly timed to the emergence of new neurons [153]. Differences in species and strains also confounded these results [108, 136]. Tasks with simple associations are largely independent of neurogenesis, whereas tasks that require complex associations or distinguishing between similar objects or locations, have higher neurogenesis components. Now there are a set of learning and memory tasks that are considered dependent on hippocampal adult neurogenesis. These include complex spatial learning tasks (radial arm maze, Morris water maze, Barnes maze, novel location test), and contextual and trace learning tasks [1]. In addition to learning and memory tasks, adult neurogenesis has also been implicated in anxiety, depression, and sociability [154–162].
Interestingly, there is a reciprocal effect of learning and adult neurogenesis. New neurons are required to learn and recall. At the same time, hippocampal-dependent learning also increases the number of new DG neurons [117, 163–165]. Other physical stimuli, such as enriched environments and voluntary running, also impact neurogenesis and subsequent learning, perhaps by activating mature neurons in the hippocampal network. Enriched environments, which for rodents can mean cages with extra stimuli beyond the standard food, water, and bedding, are found to enhance survival of new neurons [150, 166, 167]. Survival benefits seem to affect neurons that are under three weeks old, which need the most stimulation from surrounding neurons [168]. Voluntary running increases proliferation of aNSCs and can rescue the age-dependent decline in neurogenesis [169, 170], which is coupled with increased survival for new neurons [171, 172]. The exact mechanism underlying exercise-induced effect is unknown but may be caused by stimulation of the neuronal network or increased angiogenesis and blood flow. Whatever the reason, neuronal maturation, connectivity, and integration are altered by experience and environment [109, 153, 173, 174].
Adult neurogenesis regulates learning and memory, which require subtle distinctions in place, patterns or associations. The very acts of learning and memorization also affect neurogenesis, altering the proliferation of aNSCs and the survival of new neurons in need-based ways [175, 176]. Neurogenesis may also contribute emotional stability by affecting anxiety and depression [135, 157]. Although the majority of the studies referenced here are based on rodent models, the connections between adult neurogenesis and higher order learning should prompt us to examine adult neurogenesis in humans.
Cell cycle regulation of adult neurogenesis
The cell cycle process is an ordered series of events that allows for the cell to grow, replicate its DNA, and divide to produce two daughter cells. The cell cycle is divided into four phases that repeat as follows: G1 (growth phase 1), S (DNA synthesis), G2 (growth phase 2), and M (mitosis). When cells, such as neurons, exit the cell cycle, they enter the G0 phase and are generally considered post-mitotic or quiescent. It is important to note that quiescent stem cells can be stimulated to re-enter the cell cycle with the correct signals. Pairs of cyclins and cyclin-dependent kinases (CDKs) drive the progression of the cell cycle. Two families of cyclin-dependent kinase inhibitors (CKIs) negatively regulate Cyclin/CDK pairs: cytokine inducible proteins (CIPs), also known as kinase interacting proteins (KIPs), and inhibitors of CDK4s (INK4s). G1 is the longest phase of the cell cycle and is when the cell prepares for DNA replication. In this phase, CDK4/6 binds Cyclin D to phosphorylate the retinoblastoma protein (RB). This prevents RB from binding and inhibiting the E2F transcription factors allowing E2F to enter the nucleus and initiate the expression of genes required for cell cycle progression, including Cyclin E. At the checkpoint between G1 and S phases, when the cell is ready to replicate its DNA, a Cdk2-Cyclin E complex is formed, which further phosphorylates and inactivates RB. This allows for transcription of genes needed for S phase. In S phase, CDK2 complexes with Cyclin A to continue to drive the cell cycle forward. As S phase transitions to G2, CDK1 binds Cyclin A. To reach the M phase, CDK1 binds Cyclin B in a complex known as the mitosis-promoting factor (MPF).
The cell cycle and the proteins involved in it have a great deal of influence on adult NSC fate, particularly their proliferation, maintenance, quiescence, and differentiation. Each NSC is able to undergo a limited number of divisions. If NSCs divide too often without maintaining the stem cell pool, then neurogenesis cannot be sustained. Changes in cell cycle regulation also affect neuronal differentiation. In fact, there is a fairly well-documented theory known as the cell cycle length hypothesis [177], which states that the length of the G1 phase of the cell cycle determines whether a cell will proliferate or differentiate. NSCs with short G1 phases continue to proliferate, whereas those with lengthened G1 phases have time to switch over to neurogenesis. Taken together, it is not surprising that both the cell signaling pathways mentioned above and the FXRs regulate cell cycle proteins. Examples of cell cycle factors regulating adult neurogenesis are illustrated in Fig. 1.
Fig.1
Schematic illustration of cell cycle factors of adult hippocampal neurogenesis. Cell lineage-specific markers are shown at the bottom. Cell cycle regulators in orange promote proliferation or differentiation. Cell cycle factors in purple inhibit proliferation and promote quiescence. RGL, radial glia-like cell; IPC, intermediate progenitor cell.
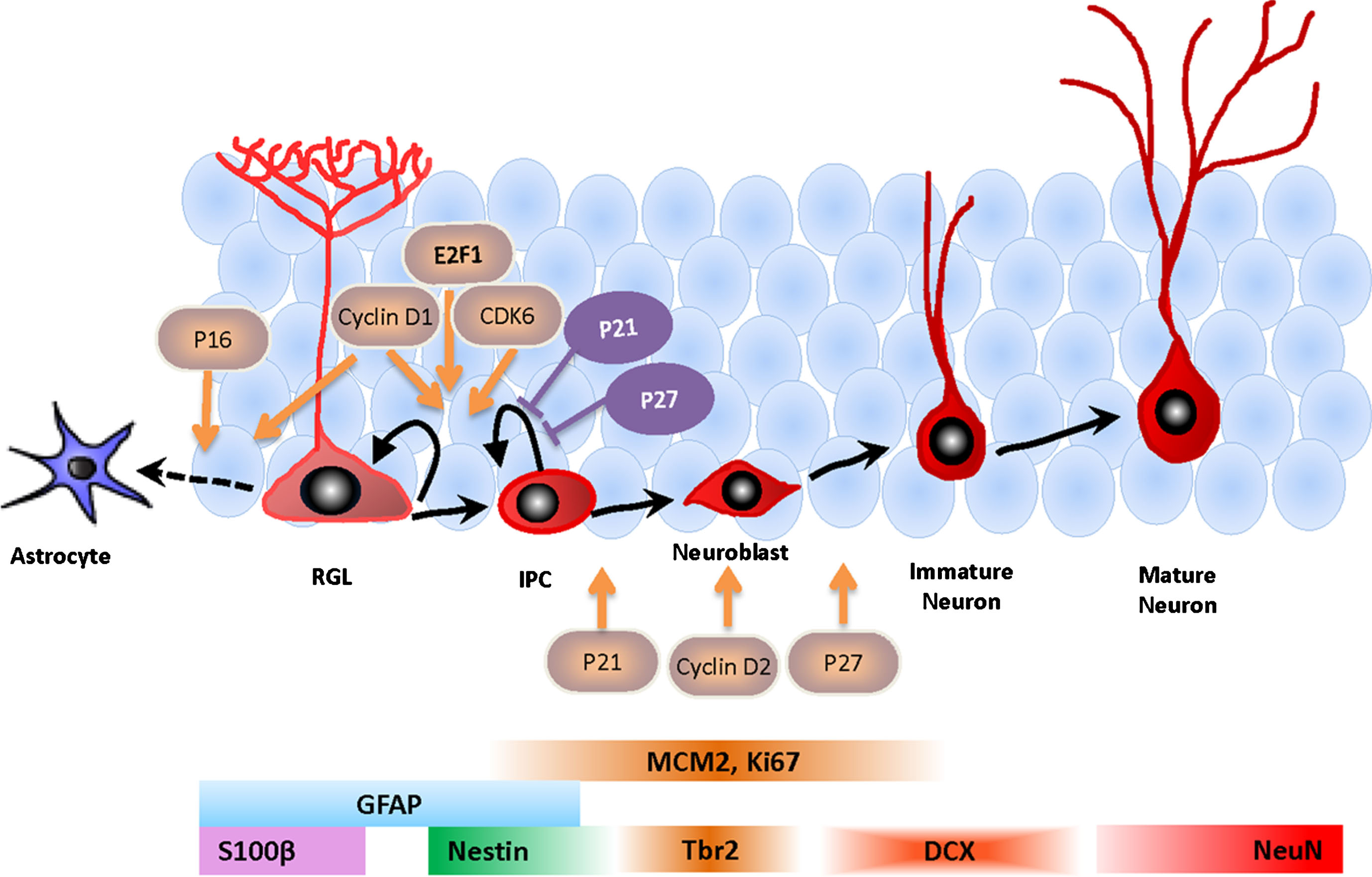
Fig.2
Comparison of human FXR proteins. NTD: N-terminal protein binding domain that contains two agenet domains; NLS: Nuclear localization sequence; KH1 & KH2: RNA-binding domains; NES: Nuclear export sequence; RGG: RNA-binding domain; NoS: Nucleolar localization sequence.
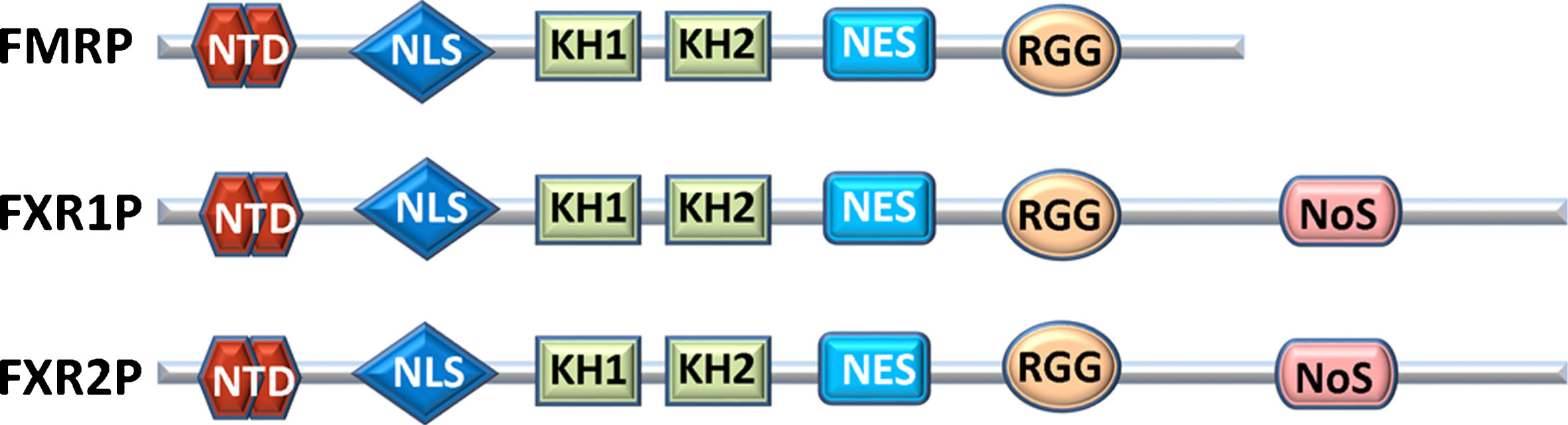
REGULATION OF ADULT NEUROGENESIS BY FRAGILE X PROTEINS
FMRP
It is no surprise that the best-studied FXR in both embryonic and adult neurogenesis is FMRP [4]. In mouse embryonic neurogenesis, the loss of FMRP increased the proliferation capacity of isolated stem cells, which was coupled with an increase in neuronal differentiation [178]. This effect may be more pronounced in human cells, but this finding needs further confirmation [178, 179]. In the mouse neocortex, loss of FMRP increased the proliferation of TBR2 + cells, leading to an increase in the number of pyramidal neurons, specifically in layer V of the cortex [180]. These findings were recapitulated in Drosophila melanogaster brain development; deletion of its only FXR gene, dFmr1, resulted in an increase in proliferative neuroblasts, which increased the number of new neurons [181]. It was hypothesized that dFMR1 regulates quiescence in neuroblasts, allowing for the maintenance of the stem cell pool [181]. A similar mechanism was proposed in mice, where FMRP controls the transition of radial glia cells into intermediate progenitors in embryonic neurogenesis. When FMRP was down-regulated, the number of radial glia cells was depleted by the increasing rate of this transition [182]. In the embryonic brain, FMRP appears to be responsible for maintenance of the stem cell pool, and this regulation is conserved across species. Without FMRP, more embryonic stem cells complete neurogenesis, resulting in more neurons. The signaling pathways and RNA targets involved in this regulation, however, remain unclear.
In adult mice, FMRP also inhibits NSC proliferation, specifically affecting the number of RGLs and IPCs [183–186]. FMRP regulates proliferation, in part by affecting the cell cycle through inhibiting translation of CyclinD1 and Cdk4 mRNA [185]. FMRP also regulates the cell cycle through the P53 pathway by decreasing the stability of Mdm2 mRNA [186]. Without FMRP, P53 is inhibited and degraded by increased levels of phosphorylated MDM2, leading to increases in proliferation and decreased neurogenesis. Treating animals with the MDM2 antagonist, Nutlin-3 can rescue learning deficits on hippocampus-dependent recognition tests [186]. Adult neurogenesis is notably different than embryonic development in that FMRP deletion causes decreases in neurogenesis and an up-regulation of glia [183]. In addition to regulation of the P53 pathway, FMRP’s control of differentiation effects are partly due to regulation of the WNT/β-catenin pathway [185]. FMRP binds GSK3β mRNA and inhibits its translation. GSK3β is a β-catenin inhibitor, and FMRP’s neurogenesis deficits can be rescued using exogenous WNT3a or a GSK3β inhibitor [185]. In addition, GSK3β inhibition can rescue hippocampal spatial and contextual learning deficits [187]. Hippocampal dysfunctions are apparent in adult Fmr1 mutant mice, and these dysfunctions can be attributed to both altered synaptic plasticity of maturation neurons and defective neurogenesis [188, 189]. As an RBP, FMRP can regulate multiple pathways and factors that converge on a single functional outcome. A broad range of RNA targets should be considered when studying any process regulated by the FXRs.
FXR2P
FXR2P also regulates adult neurogenesis, but its regulatory role is specific to the DG [184]. Like FMRP, deletion of FXR2P increases proliferation. Unlike FMRP, FXR2P promotes gliogenesis, and its deletion increases neuron numbers and decreases astrocyte numbers. FXR2P regulates the BMP pathway by decreasing the stability of Noggin mRNA. In DG NSCs without FXR2P, NOGGIN protein levels rise, and the BMP pathway is inhibited. This leads to increased NSC proliferation and neuronal differentiation but decreased astrocyte differentiation. Exogenous BMP2 rescues both proliferation and differentiation phenotypes [184]. Interestingly, this FXR2P regulation of Noggin/BMP signaling only occurs in DG NSCs. In the SVZ, FXR2P and NOGGIN are expressed in different cell types. Therefore, FXR2P deficiency has no impact on NOGGIN protein levels in SVZ NSCs. The fact that FXR2P has a unique phenotype in neurogenesis compared to FMRP is indicative of the functional differences between the FXRs. During maturation of adult new neurons, FXR2P and FMRP both regulate the AMPA receptor subunit GluA1, albeit through different mechanisms [190]. FXR2P binds and stabilizes GluA1 mRNA, whereas FMRP promotes GluA1 protein expression at the cell surface by an unknown mechanism [190]. The additive regulatory effects of these two proteins on a molecular level may partly explain the increase in behavioral deficits seen in double knockout mice [191]. It is unclear how FXR1P fits into this regulatory puzzle, but it is essential that the individual effect of FXR1P on neurogenesis be elucidated before the interaction among these three proteins can be understood.
FXR1P
FXR1P appears very similar to the other FXRs in structure and expression. FXR1P is highly expressed in neurons of the cortex and the hippocampus throughout postnatal development and in adults [63, 192]. Its differences from the other FXRs, however, make it a good candidate for further study in stem cells in the brain and elsewhere. FXR1P is the only FXR that is neonatal lethal in mouse knockouts, indicating a definitive role in development [193]. This discovery led to a wave of studies of FXR1P in muscle development, where it clearly has a function in muscle organization and maturation [103]. FXR1P promotes proliferation in myoblasts (muscle stem cells) by reducing the stability of p21 mRNA and promoting cell cycle progression [194]. In facioscapulohumeral muscular dystrophy (FSHD), an inherited myopathy characterized by alternative splicing of muscle-specific isoforms, altered FXR1P expression in myoblasts contributes to disease pathology [195]. FXR1P is implicated in other aspects of development, such as maintaining quiescence in oocytes in association with AGO2 and microRNAs [90, 91]. It is also important in early embryogenesis and spermatogenesis [196–198]. FXR1P’s capacity to regulate the cell cycle may also explain why it is classified as an oncogene or tumor suppressor in several types of cancer [199, 200]. Thus far, most roles for FXR1P are in developmental cell types, which coupled with its high expression in the brain, makes FXR1P a prime candidate for further study in aNSCs.
Although FXR1P is not known to directly cause diseases, protein interactome analysis suggests that FXR1P is highly connected with other autism-related proteins, more so than FMRP [201]. In addition, several large-scale genome-wide association studies (GWAS) have revealed that single nucleotide polymorphisms (SNPs) within the region containing FXR1 gene are associated with increased risks for bipolar disorder and schizophrenia [202–204]. In addition, a SNP associated with higher FXR1 expression levels correlates with better mood stability in both normal human populations [205] and in patients with mood disorders [206]. Although mutations in FXR1P have not yet been found in human mental disorders, neonatal lethality of Fxr1-null mice and absolute intolerance (the probability of being loss-of-function intolerant or pLI = 1.0) for loss of FXR1 gene function in humans [207] suggest that FXR1P is important in human development.
In addition to interacting with FMRP, FXR1P can also bind a large number of other autism-associated proteins [201]. SNPs in FXR1 enhance the severity of autistic behaviors [208]. A common impairment in autism and FXS is intellectual disability, which can be modeled in mouse models of learning and memory [209]. One study examined the role of FXR1P in learning and memory by deleting FXR1P in excitatory neurons in the CA1 and DG region using an CaMKIIα-Cre line of mice [210]. They found that a loss of FXR1P in these mature excitatory neurons increased long-term potentiation and led to better recall in the Morris water maze. Conversely, reversal learning, a task with a neurogenic component, was impaired. FXR1P repressed the translation of the AMPA subunit GluA2 through a GU-rich element it the 5’UTR [210]. GluA2 is important for spatial learning and long-term memory retrieval and is unaffected in either FMRP or FXR2P-deficient neurons. To assess the role of FXR1P in adult neurogenesis, we used FXR1P-floxed mice crossed with Nestin promoter-driven inducible Cre-expressing mice. We showed that deleting FXR1P in adult NSCs led to fewer NSCs and mature neurons being generated in the adult DG. We showed that deletion of FXR1P in adult NSCs leads to decreased generation of new cells from NSCs to mature new neurons in the adult DG. The decreases in the number of cells are due to reduced cell proliferation of both NSCs and IPCs rather than changes in cell fate specification. Lack of FXR1P alters the expression of cell cycle genes. We found that FXR1P binds the mRNA of the cell cycle inhibitor p21 (Cdkn1A, P21Cip1 or P21Waf1) and represses p21 mRNA expression. Restoration of p21 in FXR1P-deficient NSCs rescues the proliferation deficit. Therefore, FXR1P maintains the population of adult NSCs by regulating NSC cell-cycle proteins. The behavioral consequence of FXR1P deficiency in adult neurogenesis remains to be evaluated.
CONCLUSIONS
Adult neurogenesis is a progressive process to produce new neurons in the brain. Largely studied in rodents, where detailed mechanistic studies can be carried out, this process has been documented in all mammals, including humans [149]. Humans create 700 new neurons per day in the DG, replacing almost two percent of neurons in this region every year [135]. It is likely that this significant number of cells has a significant impact on brain function. New neurons are highly plastic, and their addition to the brain impacts certain types of higher order learning and memory. Adult neurogenesis is important for discerning subtle changes or complex associations. It is also a way to individually encode very similar memories so that they remain distinct [211]. Defects in neurogenesis have been correlated to learning and memory deficits as well as the pathology of neurological diseases [108]. In order to truly understand the importance of neurogenesis in humans, more technological advances, particularly in the use of human neural models, are needed [146].
Adult neurogenesis is a multi-step process with each step requiring proper regulation. RBPs, such as the FXRs, have significant roles in adult neurogenesis through coordinated regulation of key components. Among the FXR family members, FMRP, FXR1P, and FXR2P, FMRP has been extensively studied due to its role in FXS, while the other FXRs have remained largely overlooked. These proteins are highly conserved and contain similar domains and expression patterns. The seemingly small differences between the FXRs lead to individual functions that are just starting to be discovered. In adult neurogenesis, both FMRP and FXR2P negatively regulate proliferation but direct NSCs to divergent cell fates [184, 212]. Despite having important roles in developmental processes and cell cycle regulation, as well as genetic associations with several human diseases, the functions of FXR1P and FXR2P in neurogenesis and in the rest of the brain remain poorly understood. Because FXRs are highly expressed in the brain and are capable of binding thousands of RNA targets, they could be key coordinators of adult neurogenesis. However the genome-wide identification of FMRP targets have only been done in the mouse forebrain and in human HEK293 cells lines [14, 48]. Identification of cell type-specific FXR targets, especially in human NSCs and neurons will be critically important for understanding the function of FXRs in neurogenesis and defining both shared and unique functions of each FXR in neurodevelopment.
CONFLICT OF INTEREST STATEMENT
The authors declare no competing interests.
ACKNOWLEDGEMENTS
We thank A. Chakravorty for technical editing. This work was supported by grants from NIH (MH080434, MH078972, R21NS095632), John Merck Fund, and Brain Research Foundation to X.Z.; NIH (P30HD03352, U54HD090256) to Waisman Center IDDRC; fellowships (Molecular and Cellular Pharmacology Training Grant NIH T32 GM008688, NSRA F31MH103945, the Wayne and Jean Roper Memorial Wisconsin Distinguished Graduate Student Fellowship) to N.E.P.
REFERENCES
[1] | Kempermann G , Song H , Gage FH . Neurogenesis in the Adult Hippocampus. Cold Spring Harb Perspect Biol. (2015) ;7: (9):018812. |
[2] | Hsieh J , Zhao X . Genetics and Epigenetics in Adult Neurogenesis. Cold Spring Harbor Perspectives in Biology. (2016) ;8: (6). |
[3] | Christian KM , Song H , Ming GL . Functions and dysfunctions of adult hippocampal neurogenesis. Annu Rev Neurosci. (2014) ;37: :243–62. |
[4] | Li Y , Zhao X . Concise review: Fragile X proteins in stem cell maintenance and differentiation. Stem Cells. (2014) ;32: (7):1724–33. |
[5] | Hagerman PJ , Hagerman RJ . Fragile X-associated tremor/ataxia syndrome. Annals of the New York Academy of Sciences. (2015) ;1338: :58–70. |
[6] | Akins MR , Leblanc HF , Stackpole EE , Chyung E , Fallon JR . Systematic mapping of fragile X granules in the mouse brain reveals a potential role for presynaptic FMRP in sensorimotor functions. J Comp Neurol. (2012) ;520: (16):3687–706. |
[7] | Tamanini F , Van Unen L , Bakker C , Sacchi N , Galjaard H , Oostra BA , Hoogeveen AT . Oligomerization properties of fragile-X mental-retardation protein (FMRP) and the fragile-X-related proteins FXR1P and FXR2P. Biochem J. (1999) ;343: Pt 3:517–23. |
[8] | Zhang Y , O’Connor JP , Siomi MC , Srinivasan S , Dutra A , Nussbaum RL , Dreyfuss G . The fragile X mental retardation syndrome protein interacts with novel homologs FXR1 and FXR2. Embo j. (1995) ;14: (21):5358–66. |
[9] | Siomi MC , Zhang Y , Siomi H , Dreyfuss G . Specific sequences in the fragile X syndrome protein FMR1 and the FXR proteins mediate their binding to 60S ribosomal subunits and the interactions among them. Mol Cell Biol. (1996) ;16: (7):3825–32. |
[10] | Christie SB , Akins MR , Schwob JE , Fallon JR . The FXG: A presynaptic fragile X granule expressed in a subset of developing brain circuits. J Neurosci. (2009) ;29: (5):1514–24. |
[11] | Cheever A , Ceman S . Translation regulation of mRNAs by the fragile X family of proteins through the microRNA pathway. RNA Biology. (2009) ;6: (2):175–8. |
[12] | Hoogeveen AT , Willemsen R , Oostra BA . Fragile X syndrome, the Fragile X related proteins, and animal models. Microscopy Research and Technique. (2002) ;57: (3):148–55. |
[13] | Darnell JC , Fraser CE , Mostovetsky O , Darnell RB . Discrimination of common and unique RNA-binding activities among Fragile X mental retardation protein paralogs. Hum Mol Genet. (2009) ;18: (17):3164–77. |
[14] | Ascano M Jr , Mukherjee N , Bandaru P , Miller JB , Nusbaum JD , Corcoran DL , Langlois C , Munschauer M , Dewell S , Hafner M , Williams Z , Ohler U , Tuschl T . FMRP targets distinct mRNA sequence elements to regulate protein expression. Nature. (2012) ;492: (7429)382–6. |
[15] | Coffee RL Jr , Tessier CR , Woodruff EA 3rd , Broadie K . Fragile X mental retardation protein has a unique, evolutionarily conserved neuronal function not shared with FXR1P or FXR2P. Dis Model Mech. (2010) ;3: (7-8):471–85. |
[16] | Richter JD , Bassell GJ , Klann E . Dysregulation and restoration of translational homeostasis in fragile X syndrome. Nature reviews. Neuroscience. (2015) ;16: (10):595–605. |
[17] | Darnell JC , Klann E . The translation of translational control by FMRP: Therapeutic targets for FXS. Nature Neuroscience. (2013) ;16: (11):1530–6. |
[18] | Mientjes EJ , Nieuwenhuizen I , Kirkpatrick L , Zu T , Hoogeveen-Westerveld M , Severijnen L , Rife M , Willemsen R , Nelson DL , Oostra BA . The generation of a conditional Fmr1 knock out mouse model to study Fmrp function in vivo. Neurobiol Dis. (2006) ;21: (3):549–55. |
[19] | Santoro MR , Bray SM , Warren ST . Molecular mechanisms of fragile X syndrome: A twenty-year perspective. Annu Rev Pathol. (2012) ;7: :219–45. |
[20] | Garber KB , Visootsak J , Warren ST . Fragile X syndrome. Eur J Hum Genet. (2008) ;16: (6):666–72. |
[21] | Fu YH , Kuhl DP , Pizzuti A , Pieretti M , Sutcliffe JS , Richards S , Verkerk AJ , Holden JJ , Fenwick RG Jr , Warren ST , et al. Variation of the CGG repeat at the fragile X site results in genetic instability: Resolution of the Sherman paradox. Cell. (1991) ;67: (6):1047–58. |
[22] | Jacquemont S , Hagerman RJ , Leehey M , Grigsby J , Zhang L , Brunberg JA , Greco C , Des Portes V , Jardini T , Levine R , Berry-Kravis E , Brown WT , Schaeffer S , Kissel J , Tassone F , Hagerman PJ . Fragile X premutation tremor/ataxia syndrome: Molecular, clinical, and neuroimaging correlates. Am J Hum Genet. (2003) ;72: (4):869–78. |
[23] | Pirozzi F , Tabolacci E , Neri G . The FRAXopathies: Definition, overview, and update. Am J Med Genet A. (2011) ;155a: (8):1803–16. |
[24] | Eadie BD , Zhang WN , Boehme F , Gil-Mohapel J , Kainer L , Simpson JM , Christie BR . Fmr1 knockout mice show reduced anxiety and alterations in neurogenesis that are specific to the ventral dentate gyrus. Neurobiol Dis. (2009) ;36: (2):361–73. |
[25] | Bear MF , Huber KM , Warren ST . The mGluR theory of fragile X mental retardation. Trends Neurosci. (2004) ;27: (7):370–7. |
[26] | Berry-Kravis E . Mechanism-based treatments in neurodevelopmental disorders: Fragile X syndrome. Pediatr Neurol. (2014) ;50: (4):297–302. |
[27] | Cameron HA , McEwen BS , Gould E . Regulation of adult neurogenesis by excitatory input and NMDA receptor activation in the dentate gyrus. J Neurosci. (1995) ;15: (6):4687–92. |
[28] | Gotz M , Nakafuku M , Petrik D . Neurogenesis in the Developing and Adult Brain-Similarities and Key Differences. Cold Spring Harb Perspect Biol. (2016) ;8: (7). |
[29] | Eadie BD , Cushman J , Kannangara TS , Fanselow MS , Christie BR . NMDA receptor hypofunction in the dentate gyrus and impaired context discrimination in adult Fmr1 knockout mice. Hippocampus. (2012) ;22: (2):241–54. |
[30] | Bostrom CA , Majaess NM , Morch K , White E , Eadie BD , Christie BR . Rescue of NMDAR-dependent synaptic plasticity in Fmr1 knock-out mice. Cerebral Cortex. (2015) ;25: (1):271–9. |
[31] | Yau SY , Bostrom CA , Chiu J , Fontaine CJ , Sawchuk S , Meconi A , Wortman RC , Truesdell E , Truesdell A , Chiu C , Hryciw BN , Eadie BD , Ghilan M , Christie BR . Impaired bidirectional NMDA receptor dependent synaptic plasticity in the dentate gyrus of adult female Fmr1 heterozygous knockout mice. Neurobiol Dis. (2016) ;96: :261–70. |
[32] | Toft AK , Lundbye CJ , Banke TG . Dysregulated NMDA-Receptor Signaling Inhibits Long-Term Depression in a Mouse Model of Fragile X Syndrome. J Neurosci. (2016) ;36: (38):9817–27. |
[33] | Schutt J , Falley K , Richter D , Kreienkamp HJ , Kindler S . Fragile X mental retardation protein regulates the levels of scaffold proteins and glutamate receptors in postsynaptic densities. The Journal of Biological Chemistry. (2009) ;284: (38):25479–87. |
[34] | Darnell JC , Van Driesche SJ , Zhang C , Hung KY , Mele A , Fraser CE , Stone EF , Chen C , Fak JJ , Chi SW , Licatalosi DD , Richter JD , Darnell RB . FMRP stalls ribosomal translocation on mRNAs linked to synaptic function and autism. Cell. (2011) ;146: (2):247–61. |
[35] | Coy JF , Sedlacek Z , Bachner D , Hameister H , Joos S , Lichter P , Delius H , Poustka A . Highly conserved 3’ UTR and expression pattern of FXR1 points to a divergent gene regulation of FXR1 and FMR1. Hum Mol Genet. (1995) ;4: (12):2209–18. |
[36] | Kirkpatrick LL , McIlwain KA , Nelson DL . Comparative genomic sequence analysis of the FXR gene family: FMR1, FXR1, and FXR2. Genomics. (2001) ;78: (3):169–77. |
[37] | Mazroui R , Huot ME , Tremblay S , Boilard N , Labelle Y , Khandjian EW . Fragile X Mental Retardation protein determinants required for its association with polyribosomal mRNPs. Hum Mol Genet. (2003) ;12: (23):3087–96. |
[38] | Adinolfi S , Ramos A , Martin SR , Dal Piaz F , Pucci P , Bardoni B , Mandel JL , Pastore A . The N-terminus of the fragile X mental retardation protein contains a novel domain involved in dimerization and RNA binding. Biochemistry. (2003) ;42: (35):10437–44. |
[39] | Maurer-Stroh S , Dickens NJ , Hughes-Davies L , Kouzarides T , Eisenhaber F , Ponting CP . The Tudor domain ‘Royal Family’: Tudor, plant Agenet, Chromo, PWWP and MBT domains. Trends Biochem Sci. (2003) ;28: (2):69–74. |
[40] | Ramos A , Hollingworth D , Adinolfi S , Castets M , Kelly G , Frenkiel TA , Bardoni B , Pastore A . The structure of the N-terminal domain of the fragile X mental retardation prote: A platform for protein-protein interaction. Structure. (2006) ;14: (1):21–31. |
[41] | Adams-Cioaba MA , Guo Y , Bian C , Amaya MF , Lam R , Wasney GA , Vedadi M , Xu C , Min J . Structural studies of the tandem Tudor domains of fragile X mental retardation related proteins FXR1 and FXR2. PLoS One. (2010) ;5: (11):e13559. |
[42] | Alpatov R , Lesch BJ , Nakamoto-Kinoshita M , Blanco A , Chen S , Stutzer A , Armache KJ , Simon MD , Xu C , Ali M , Murn J , Prisic S , Kutateladze TG , Vakoc CR , Min J , Kingston RE , Fischle W , Warren ST , Page DC , Shi Y . A chromatin-dependent role of the fragile X mental retardation protein FMRP in the DNA damage response. Cell. (2014) ;157: (4):869–81. |
[43] | Suberbielle E , Sanchez PE , Kravitz AV , Wang X , Ho K , Eilertson K , Devidze N , Kreitzer AC , Mucke L . Physiologic brain activity causes DNA double-strand breaks in neurons, with exacerbation by amyloid-beta. Nat Neurosci. (2013) ;16: (5):613–21. |
[44] | Kim M , Bellini M , Ceman S . Fragile X mental retardation protein FMRP binds mRNAs in the nucleus. Mol Cell Biol. (2009) ;29: (1):214–28. |
[45] | Collins SC , Bray SM , Suhl JA , Cutler DJ , Coffee B , Zwick ME , Warren ST . Identification of novel FMR1 variants by massively parallel sequencing in developmentally delayed males. Am J Med Genet A. (2010) ;152a: (10):2512–20. |
[46] | Tamanini F , Kirkpatrick LL , Schonkeren J , van Unen L , Bontekoe C , Bakker C , Nelson DL , Galjaard H , Oostra BA , Hoogeveen AT . The fragile X-related proteins FXR1P and FXR2P contain a functional nucleolar-targeting signal equivalent to the HIV-1 regulatory proteins. Hum Mol Genet. (2000) ;9: (10):1487–93. |
[47] | Feng Y , Gutekunst CA , Eberhart DE , Yi H , Warren ST , Hersch SM . Fragile X mental retardation prote: Nucleocytoplasmic shuttling and association with somatodendritic ribosomes. J Neurosci. (1997) ;17: (5):1539–47. |
[48] | Darnell JC , Jensen KB , Jin P , Brown V , Warren ST , Darnell RB . Fragile X mental retardation protein targets G quartet mRNAs important for neuronal function. Cell. (2001) ;107: (4):489–99. |
[49] | Blackwell E , Ceman S . Arginine methylation of RNA-binding proteins regulates cell function and differentiation. Mol Reprod Dev. (2012) ;79: (3):163–75. |
[50] | Bechara E , Davidovic L , Melko M , Bensaid M , Tremblay S , Grosgeorge J , Khandjian EW , Lalli E , Bardoni B . Fragile X related protein 1 isoforms differentially modulate the affinity of fragile X mental retardation protein for G-quartet RNA structure. Nucleic Acids Res. (2007) ;35: (1):299–306. |
[51] | Brown MR , Kronengold J , Gazula VR , Chen Y , Strumbos JG , Sigworth FJ , Navaratnam D , Kaczmarek LK . Fragile X mental retardation protein controls gating of the sodium-activated potassium channel Slack. Nat Neurosci. (2010) ;13: (7):819–21. |
[52] | Darnell JC , Mostovetsky O , Darnell RB . FMRP RNA targets: Identification and validation. Genes Brain Behav. (2005) ;4: (6):341–9. |
[53] | Adinolfi S , Bagni C , Musco G , Gibson T , Mazzarella L , Pastore A . Dissecting FMR1, the protein responsible for fragile X syndrome, in its structural and functional domains. Rna. (1999) ;5: (9):1248–58. |
[54] | Siomi H , Choi M , Siomi MC , Nussbaum RL , Dreyfuss G . Essential role for KH domains in RNA binding: Impaired RNA binding by a mutation in the KH domain of FMR1 that causes fragile X syndrome. Cell. (1994) ;77: (1):33–9. |
[55] | Tamanini F , Meijer N , Verheij C , Willems PJ , Galjaard H , Oostra BA , Hoogeveen AT . FMRP is associated to the ribosomes via RNA. Hum Mol Genet. (1996) ;5: (6):809–13. |
[56] | De Boulle K , Verkerk AJ , Reyniers E , Vits L , Hendrickx J , Van Roy B , Van den Bos F , de Graaff E , Oostra BA , Willems PJ . A point mutation in the FMR-1 gene associated with fragile X mental retardation. Nat Genet. (1993) ;3: (1):31–5. |
[57] | Tamanini F , Willemsen R , van Unen L , Bontekoe C , Galjaard H , Oostra BA , Hoogeveen AT . Differential expression of FMR1, FXR1 and FXR2 proteins in human brain and testis. Hum Mol Genet. (1997) ;6: (8):1315–22. |
[58] | Khandjian E , Fortin A , Thibodeau A , Tremblay S , Côté F , Devys D , Mandel JL , Rousseau F . A heterogeneous set of FMR1 proteins is widely distributed in mouse tissues and is modulated in cell culture. (1995) ;4: :783–9. |
[59] | Huot ME , Mazroui R , Leclerc P , Khandjian EW . Developmental expression of the fragile X-related 1 proteins in mouse testis: Association with microtubule elements. Hum Mol Genet. (2001) ;10: (24):2803–11. |
[60] | Bakker CE , Kooy RF , D’Hooge R , Tamanini F , Willemsen R , Nieuwenhuizen I , De Vries BBA , Reyniers E , Hoogeveen AT , Willems PJ , De Deyn PP , Oostra BA . Introduction of a FMR1 transgene in the fragile X knockout mouse. Neuroscience Research Communications. (2000) ;26: (3):265–77. |
[61] | Khandjian EW , Bardoni B , Corbin F , Sittler A , Giroux S , Heitz D , Tremblay S , Pinset C , Montarras D , Rousseau F , Mandel J . Novel isoforms of the fragile X related protein FXR1P are expressed during myogenesis. Hum Mol Genet. (1998) ;7: (13):2121–8. |
[62] | Zarnescu DC , Gregorio CC . Fragile hearts: New insights into translational control in cardiac muscle. Trends Cardiovasc Med. (2013) ;23: (8):275–81. |
[63] | Cook D , Sanchez-Carbente Mdel R , Lachance C , Radzioch D , Tremblay S , Khandjian EW , DesGroseillers L , Murai KK . Fragile X related protein 1 clusters with ribosomes and messenger RNAs at a subset of dendritic spines in the mouse hippocampus. PLoS One. (2011) ;6: (10):e26120. |
[64] | Antar LN , Li C , Zhang H , Carroll RC , Bassell GJ . Local functions for FMRP in axon growth cone motility and activity-dependent regulation of filopodia and spine synapses. Mol Cell Neurosci. (2006) ;32: (1-2):37–48. |
[65] | Khandjian EW , Huot ME , Tremblay S , Davidovic L , Mazroui R , Bardoni B . Biochemical evidence for the association of fragile X mental retardation protein with brain polyribosomal ribonucleoparticles. Proc Natl Acad Sci U S A. (2004) ;101: (36):13357–62. |
[66] | Willemsen R , Bontekoe C , Tamanini F , Galjaard H , Hoogeveen A , Oostra B . Association of FMRP with ribosomal precursor particles in the nucleolus. Biochem Biophys Res Commun. (1996) ;225: (1):27–33. |
[67] | Tamanini F , Bontekoe C , Bakker CE , van Unen L , Anar B , Willemsen R , Yoshida M , Galjaard H , Oostra BA , Hoogeveen AT . Different targets for the fragile X-related proteins revealed by their distinct nuclear localizations. Hum Mol Genet. (1999) ;8: (5):863–9. |
[68] | Chyung E , LeBlanc HF , Fallon JR , Akins MR . Fragile X granules are a family of axonal ribonucleoprotein particles with circuit-dependent protein composition and mRNA cargos. The Journal of Comparative Neurology. (2018) ;526: (1):96–108. |
[69] | Napoli I , Mercaldo V , Boyl PP , Eleuteri B , Zalfa F , De Rubeis S , Di Marino D , Mohr E , Massimi M , Falconi M , Witke W , Costa-Mattioli M , Sonenberg N , Achsel T , Bagni C . The fragile X syndrome protein represses activity-dependent translation through CYFIP1, a new 4E-BP. Cell. (2008) ;134: (6):1042–54. |
[70] | Schenck A , Bardoni B , Langmann C , Harden N , Mandel JL , Giangrande A . CYFIP/Sra-1 controls neuronal connectivity in Drosophila and links the Rac1 GTPase pathway to the fragile X protein. Neuron. (2003) ;38: (6):887–98. |
[71] | Schenck A , Bardoni B , Moro A , Bagni C , Mandel JL . A highly conserved protein family interacting with the fragile X mental retardation protein (FMRP) and displaying selective interactions with FMRP-related proteins FXR1P and FXR2P. Proc Natl Acad Sci U S A. (2001) ;98: (15):8844–9. |
[72] | Darnell RB . HITS-CLIP: Panoramic views of protein-RNA regulation in living cells. Wiley Interdiscip Rev RNA. (2010) ;1: (2):266–86. |
[73] | Ceman S , O’Donnell WT , Reed M , Patton S , Pohl J , Warren ST . Phosphorylation influences the translation state of FMRP-associated polyribosomes. Hum Mol Genet. (2003) ;12: (24):3295–305. |
[74] | Wang H , Wu LJ , Kim SS , Lee FJ , Gong B , Toyoda H , Ren M , Shang YZ , Xu H , Liu F , Zhao MG , Zhuo M . FMRP acts as a key messenger for dopamine modulation in the forebrain. Neuron. (2008) ;59: (4):634–47. |
[75] | Pfeiffer BE , Huber KM . Fragile X mental retardation protein induces synapse loss through acute postsynaptic translational regulation. J Neurosci. (2007) ;27: (12):3120–30. |
[76] | Comery TA , Harris JB , Willems PJ , Oostra BA , Irwin SA , Weiler IJ , Greenough WT . Abnormal dendritic spines in fragile X knockout mice: Maturation and pruning deficits. Proc Natl Acad Sci U S A. (1997) ;94: (10):5401–4. |
[77] | Irwin SA , Swain RA , Christmon CA , Chakravarti A , Weiler IJ , Greenough WT . Evidence for altered Fragile-X mental retardation protein expression in response to behavioral stimulation. Neurobiol Learn Mem. (2000) ;74: (1):87–93. |
[78] | Nimchinsky EA , Oberlander AM , Svoboda K . Abnormal development of dendritic spines in FMR1 knock-out mice. J Neurosci. (2001) ;21: (14):5139–46. |
[79] | Tessier CR , Broadie K . Drosophila fragile X mental retardation protein developmentally regulates activity-dependent axon pruning. Development. (2008) ;135: (8):1547–57. |
[80] | Patel AB , Loerwald KW , Huber KM , Gibson JR . Postsynaptic FMRP promotes the pruning of cell-to-cell connections among pyramidal neurons in the L5A neocortical network. J Neurosci. (2014) ;34: (9):3413–8. |
[81] | Galvez R , Lynn Smith R , Greenough WT . Olfactory bulb mitral cell dendritic pruning abnormalities in a mouse model of the Fragile-X mental retardation syndrome: Further support for FMRP’s involvement in dendritic development. (2005) ;157: :214–6. |
[82] | Jin P , Zarnescu DC , Ceman S , Nakamoto M , Mowrey J , Jongens TA , Nelson DL , Moses K , Warren ST . Biochemical and genetic interaction between the fragile X mental retardation protein and the microRNA pathway. Nat Neurosci. (2004) ;7: (2):113–7. |
[83] | Plante I , Provost P . Hypothesis: A role for fragile X mental retardation protein in mediating and relieving microRNA-guided translational repression? J Biomed Biotechnol. (2006) ;2006: (4):16806. |
[84] | Edbauer D , Neilson JR , Foster KA , Wang CF , Seeburg DP , Batterton MN , Tada T , Dolan BM , Sharp PA , Sheng M . Regulation of synaptic structure and function by FMRP-associated microRNAs miR-125b and miR-132. Neuron. (2010) ;65: (3):373–84. |
[85] | Muddashetty RS , Nalavadi VC , Gross C , Yao X , Xing L , Laur O , Warren ST , Bassell GJ . Reversible inhibition of PSD-95 mRNA translation by miR-125a, FMRP phosphorylation, and mGluR signaling. Mol Cell. (2011) ;42: (5):673–88. |
[86] | Didiot MC , Tian Z , Schaeffer C , Subramanian M , Mandel JL , Moine H . The G-quartet containing FMRP binding site in FMR1 mRNA is a potent exonic splicing enhancer. Nucleic Acids Res. (2008) ;36: (15):4902–12. |
[87] | Xu XL , Zong R , Li Z , Biswas MH , Fang Z , Nelson DL , Gao FB . FXR1P but not FMRP regulates the levels of mammalian brain-specific microRNA-9 and microRNA-124. J Neurosci. (2011) ;31: (39):13705–9. |
[88] | Vasudevan S , Steitz JA . AU-rich-element-mediated upregulation of translation by FXR1 and Argonaute 2. Cell. (2007) ;128: (6):1105–18. |
[89] | Vasudevan S , Tong Y , Steitz JA . Switching from repression to activation: MicroRNAs can up-regulate translation. Science. (2007) ;318: (5858):1931–4. |
[90] | Mortensen RD , Serra M , Steitz JA , Vasudevan S . Posttranscriptional activation of gene expression in Xenopus laevis oocytes by microRNA-protein complexes (microRNPs). Proc Natl Acad Sci U S A. (2011) ;108: (20):8281–6. |
[91] | Truesdell SS , Mortensen RD , Seo M , Schroeder JC , Lee JH , LeTonqueze O , Vasudevan S . MicroRNA-mediated mRNA translation activation in quiescent cells and oocytes involves recruitment of a nuclear microRNP. Sci Rep. (2012) ;2: :842. |
[92] | Davidovic L , Durand N , Khalfallah O , Tabet R , Barbry P , Mari B , Sacconi S , Moine H , Bardoni B . A novel role for the RNA-binding protein FXR1P in myoblasts cell-cycle progression by modulating p21/Cdkn1a/Cip1/Waf1 mRNA stability. PLoS Genet. (2013) ;9: (3):e1003367. |
[93] | Majumder M , House R , Palanisamy N , Qie S , Day TA , Neskey D , Diehl JA , Palanisamy V . Correction: RNA-Binding Protein FXR1 Regulates p21 and TERC RNA to Bypass p53-Mediated Cellular Senescence in OSCC. PLoS Genet. (2016) ;12: (10):e1006411. |
[94] | Ivanovska I , Ball AS , Diaz RL , Magnus JF , Kibukawa M , Schelter JM , Kobayashi SV , Lim L , Burchard J , Jackson AL , Linsley PS , Cleary MA . MicroRNAs in the miR-106b family regulate p21/CDKN1A and promote cell cycle progression. Mol Cell Biol. (2008) ;28: (7):2167–74. |
[95] | Altman J , Das GD . Autoradiographic and histological evidence of postnatal hippocampal neurogenesis in rats. J Comp Neurol. (1965) ;124: (3):319–35. |
[96] | Mirzadeh Z , Merkle FT , Soriano-Navarro M , Garcia-Verdugo JM , Alvarez-Buylla A . Neural stem cells confer unique pinwheel architecture to the ventricular surface in neurogenic regions of the adult brain. Cell Stem Cell. (2008) ;3: (3):265–78. |
[97] | Doetsch F , Garcia-Verdugo JM , Alvarez-Buylla A . Cellular composition and three-dimensional organization of the subventricular germinal zone in the adult mammalian brain. J Neurosci. (1997) ;17: (13):5046–61. |
[98] | Doetsch F , Caille I , Lim DA , Garcia-Verdugo JM , Alvarez-Buylla A . Subventricular zone astrocytes are neural stem cells in the adult mammalian brain. Cell. (1999) ;97: (6):703–16. |
[99] | Garcia-Verdugo JM , Doetsch F , Wichterle H , Lim DA , Alvarez-Buylla A . Architecture and cell types of the adult subventricular zone: In search of the stem cells. J Neurobiol. (1998) ;36: (2):234–48. |
[100] | Codega P , Silva-Vargas V , Paul A , Maldonado-Soto AR , Deleo AM , Pastrana E , Doetsch F . Prospective identification and purification of quiescent adult neural stem cells from their in vivo niche. Neuron. (2014) ;82: (3):545–59. |
[101] | Costa MR , Ortega F , Brill MS , Beckervordersandforth R , Petrone C , Schroeder T , Gotz M , Berninger B . Continuous live imaging of adult neural stem cell division and lineage progression in vitro. Development. (2011) ;138: (6):1057–68. |
[102] | Lois C , Garcia-Verdugo JM , Alvarez-Buylla A . Chain migration of neuronal precursors. Science. (1996) ;271: (5251):978–81. |
[103] | Whitman SA , Cover C , Yu L , Nelson DL , Zarnescu DC , Gregorio CC . Desmoplakin and talin2 are novel mRNA targets of fragile X-related protein-1 in cardiac muscle. Circ Res. (2011) ;109: (3):262–71. |
[104] | Bonaguidi MA , Wheeler MA , Shapiro JS , Stadel RP , Sun GJ , Ming GL , Song H . In vivo clonal analysis reveals self-renewing and multipotent adult neural stem cell characteristics. Cell. (2011) ;145: (7):1142–55. |
[105] | Encinas JM , Michurina TV , Peunova N , Park JH , Tordo J , Peterson DA , Fishell G , Koulakov A , Enikolopov G . Division-coupled astrocytic differentiation and age-related depletion of neural stem cells in the adult hippocampus. Cell Stem Cell. (2011) ;8: (5):566–79. |
[106] | Sierra A , Martin-Suarez S , Valcarcel-Martin R , Pascual-Brazo J , Aelvoet SA , Abiega O , Deudero JJ , Brewster AL , Bernales I , Anderson AE , Baekelandt V , Maletic-Savatic M , Encinas JM . Neuronal hyperactivity accelerates depletion of neural stem cells and impairs hippocampal neurogenesis. Cell Stem Cell. (2015) ;16: (5):488–503. |
[107] | Gebara E , Bonaguidi MA , Beckervordersandforth R , Sultan S , Udry F , Gijs PJ , Lie DC , Ming GL , Song H , Toni N . Heterogeneity of Radial Glia-Like Cells in the Adult Hippocampus. Stem Cells. (2016) ;34: (4):997–1010. |
[108] | Deng W , Aimone JB , Gage FH . New neurons and new memories: How does adult hippocampal neurogenesis affect learning and memory? Nat Rev Neurosci. (2010) ;11: (5):339–50. |
[109] | Zhao C , Teng EM , Summers RG Jr , Ming GL , Gage FH . Distinct morphological stages of dentate granule neuron maturation in the adult mouse hippocampus. J Neurosci. (2006) ;26: (1):3–11. |
[110] | Esposito MS , Piatti VC , Laplagne DA , Morgenstern NA , Ferrari CC , Pitossi FJ , Schinder AF . Neuronal differentiation in the adult hippocampus recapitulates embryonic development. J Neurosci. (2005) ;25: (44):10074–86. |
[111] | Ge S , Goh EL , Sailor KA , Kitabatake Y , Ming GL , Song H . GABA regulates synaptic integration of newly generated neurons in the adult brain. Nature. (2006) ;439: (7076):589–93. |
[112] | Hastings NB , Seth MI , Tanapat P , Rydel TA , Gould E . Granule neurons generated during development extend divergent axon collaterals to hippocampal area CA3. J Comp Neurol. (2002) ;452: (4):324–33. |
[113] | Overstreet Wadiche L , Bromberg DA , Bensen AL , Westbrook GL . GABAergic signaling to newborn neurons in dentate gyrus. J Neurophysiol. (2005) ;94: (6):4528–32. |
[114] | Markwardt SJ , Wadiche JI , Overstreet-Wadiche LS , Input-specific GABAergic signaling to newborn neurons in adult dentate gyrus. J Neurosci. (2009) ;29: (48):15063–72. |
[115] | Jagasia R , Steib K , Englberger E , Herold S , Faus-Kessler T , Saxe M , Gage FH , Song H , Lie DC . GABA-cAMP response element-binding protein signaling regulates maturation and survival of newly generated neurons in the adult hippocampus. J Neurosci. (2009) ;29: (25):7966–77. |
[116] | Toni N , Teng EM , Bushong EA , Aimone JB , Zhao C , Consiglio A , van Praag H , Martone ME , Ellisman MH , Gage FH . Synapse formation on neurons born in the adult hippocampus. Nat Neurosci. (2007) ;10: (6):727–34. |
[117] | Leuner B , Mendolia-Loffredo S , Kozorovitskiy Y , Samburg D , Gould E , Shors TJ . Learning enhances the survival of new neurons beyond the time when the hippocampus is required for memory. J Neurosci. (2004) ;24: (34):7477–81. |
[118] | Ge S , Yang CH , Hsu KS , Ming GL , Song H . A critical period for enhanced synaptic plasticity in newly generated neurons of the adult brain. Neuron. (2007) ;54: (4):559–66. |
[119] | Schmidt-Hieber C , Jonas P , Bischofberger J . Enhanced synaptic plasticity in newly generated granule cells of the adult hippocampus. Nature. (2004) ;429: (6988):184–7. |
[120] | Eriksson PS , Perfilieva E , Bjork-Eriksson T , Alborn AM , Nordborg C , Peterson DA , Gage FH . Neurogenesis in the adult human hippocampus. Nat Med. (1998) ;4: (11):1313–7. |
[121] | Knoth R , Singec I , Ditter M , Pantazis G , Capetian P , Meyer RP , Horvat V , Volk B , Kempermann G . Murine features of neurogenesis in the human hippocampus across the lifespan from 0 to 100 years. PLoS One. (2010) ;5: (1):e8809. |
[122] | Curtis MA , Low VF , Faull RL . Neurogenesis and progenitor cells in the adult human bra: A comparison between hippocampal and subventricular progenitor proliferation. Dev Neurobiol. (2012) ;72: (7):990–1005. |
[123] | Goritz C , Frisen J . Neural stem cells and neurogenesis in the adult. Cell Stem Cell. (2012) ;10: (6):657–9. |
[124] | West MJ , Gundersen HJ . Unbiased stereological estimation of the number of neurons in the human hippocampus. J Comp Neurol. (1990) ;296: (1):1–22. |
[125] | West MJ . Regionally specific loss of neurons in the aging human hippocampus. Neurobiol Aging. (1993) ;14: (4):287–93. |
[126] | Harding AJ , Halliday GM , Kril JJ . Variation in hippocampal neuron number with age and brain volume. Cereb Cortex. (1998) ;8: (8):710–8. |
[127] | Korbo L , Amrein I , Lipp HP , Wolfer D , Regeur L , Oster S , Pakkenberg B . No evidence for loss of hippocampal neurons in non-Alzheimer dementia patients. Acta Neurol Scand. (2004) ;109: (2):132–9. |
[128] | Simic G , Kostovic I , Winblad B , Bogdanovic N . Volume and number of neurons of the human hippocampal formation in normal aging and Alzheimer’s disease. J Comp Neurol. (1997) ;379: (4):482–94. |
[129] | Sanai N , Tramontin AD , Quinones-Hinojosa A , Barbaro NM , Gupta N , Kunwar S , Lawton MT , McDermott MW , Parsa AT , Manuel-Garcia Verdugo J , Berger MS , Alvarez-Buylla A . Unique astrocyte ribbon in adult human brain contains neural stem cells but lacks chain migration. Nature. (2004) ;427: (6976):740–4. |
[130] | Curtis MA , Kam M , Nannmark U , Anderson MF , Axell MZ , Wikkelso C , Holtas S , van Roon-Mom WM , Bjork-Eriksson T , Nordborg C , Frisen J , Dragunow M , Faull RL , Eriksson PS . Human neuroblasts migrate to the olfactory bulb via a lateral ventricular extension. Science. (2007) ;315: (5816):1243–9. |
[131] | Sanai N , Nguyen T , Ihrie RA , Mirzadeh Z , Tsai HH , Wong M , Gupta N , Berger MS , Huang E , Garcia-Verdugo JM , Rowitch DH , Alvarez-Buylla A . Corridors of migrating neurons in the human brain and their decline during infancy. Nature. (2011) ;478: (7369):382–6. |
[132] | Wang KC , Chang HY . Molecular mechanisms of long noncoding RNAs. Mol Cell. (2011) ;43: (6):904–14. |
[133] | Maresh A , Rodriguez Gil D , Whitman MC , Greer CA . Principles of glomerular organization in the human olfactory bulb–implications for odor processing. PLoS One. (2008) ;3: (7):e2640. |
[134] | Spalding KL , Bhardwaj RD , Buchholz BA , Druid H , Frisen J . Retrospective birth dating of cells in humans. Cell. (2005) ;122: (1):133–43. |
[135] | Spalding KL , Bergmann O , Alkass K , Bernard S , Salehpour M , Huttner HB , Bostrom E , Westerlund I , Vial C , Buchholz BA , Possnert G , Mash DC , Druid H , Frisen J . Dynamics of hippocampal neurogenesis in adult humans. Cell. (2013) ;153: (6):1219–27. |
[136] | Kang E , Wen Z , Song H , Christian KM , Ming GL . Adult Neurogenesis and Psychiatric Disorders. Cold Spring Harb Perspect Biol. (2016) ;8: (9). |
[137] | Boldrini M , Santiago AN , Hen R , Dwork AJ , Rosoklija GB , Tamir H , Arango V , John Mann J . Hippocampal granule neuron number and dentate gyrus volume in antidepressant-treated and untreated major depression. Neuropsychopharmacology. (2013) ;38: (6):1068–77. |
[138] | Bremner JD . Alterations in brain structure and function associated with post-traumatic stress disorder. Semin Clin Neuropsychiatry. (1999) ;4: (4):249–55. |
[139] | Lie DC , Song H , Colamarino SA , Ming GL , Gage FH . Neurogenesis in the adult brain: New strategies for central nervous system diseases. Annu Rev Pharmacol Toxicol. (2004) ;44: :399–421. |
[140] | Mirescu C , Gould E . Stress and adult neurogenesis. Hippocampus. (2006) ;16: (3):233–8. |
[141] | Revest JM , Dupret D , Koehl M , Funk-Reiter C , Grosjean N , Piazza PV , Abrous DN . Adult hippocampal neurogenesis is involved in anxiety-related behaviors. Mol Psychiatry. (2009) ;14: (10):959–67. |
[142] | Morris SA , Eaves DW , Smith AR , Nixon K . Alcohol inhibition of neurogenesis: A mechanism of hippocampal neurodegeneration in an adolescent alcohol abuse model. Hippocampus. (2010) ;20: (5):596–607. |
[143] | Nixon K , Morris SA , Liput DJ , Kelso ML . Roles of neural stem cells and adult neurogenesis in adolescent alcohol use disorders. Alcohol. (2010) ;44: (1):39–56. |
[144] | Reif A , Fritzen S , Finger M , Strobel A , Lauer M , Schmitt A , Lesch KP . Neural stem cell proliferation is decreased in schizophrenia, but not in depression. Mol Psychiatry. (2006) ;11: (5):514–22. |
[145] | Clelland CD , Choi M , Romberg C , Clemenson GD Jr , Fragniere A , Tyers P , Jessberger S , Saksida LM , Barker RA , Gage FH , Bussey TJ . A functional role for adult hippocampal neurogenesis in spatial pattern separation. Science. (2009) ;325: (5937):210–3. |
[146] | Ho NF , Hooker JM , Sahay A , Holt DJ , Roffman JL . In vivo imaging of adult human hippocampal neurogenesis: Progress, pitfalls and promise. Mol Psychiatry. (2013) ;18: (4):404–16. |
[147] | Manganas LN , Zhang X , Li Y , Hazel RD , Smith SD , Wagshul ME , Henn F , Benveniste H , Djuric PM , Enikolopov G , Maletic-Savatic M . Magnetic resonance spectroscopy identifies neural progenitor cells in the live human brain. Science. (2007) ;318: (5852)980–5. |
[148] | Licht T , Keshet E . The vascular niche in adult neurogenesis. Mech Dev. (2015) ;138 Pt 1: :56–62. |
[149] | Kempermann G . New neurons for ‘survival of the fittest’. Nat Rev Neurosci. (2012) ;13: (10):727–36. |
[150] | Kempermann G , Kuhn HG , Gage FH . More hippocampal neurons in adult mice living in an enriched environment. Nature. (1997) ;386: (6624):493–5. |
[151] | Goldman SA , Nottebohm F . Neuronal production, migration, and differentiation in a vocal control nucleus of the adult female canary brain. Proc Natl Acad Sci U S A. (1983) ;80: (8):2390–4. |
[152] | Scharff C , Kirn JR , Grossman M , Macklis JD , Nottebohm F . Targeted neuronal death affects neuronal replacement and vocal behavior in adult songbirds. Neuron. (2000) ;25: (2):481–92. |
[153] | Goncalves JT , Schafer ST , Gage FH . Adult Neurogenesis in the Hippocampus: From Stem Cells to Behavior. Cell. (2016) ;167: (4):897–914. |
[154] | Jacobs BL , van Praag H , Gage FH . Adult brain neurogenesis and psychiatry: A novel theory of depression. Mol Psychiatry. (2000) ;5: (3):262–9. |
[155] | Sahay A , Hen R . Adult hippocampal neurogenesis in depression. Nat Neurosci. (2007) ;10: (9):1110–5. |
[156] | Kheirbek MA , Drew LJ , Burghardt NS , Costantini DO , Tannenholz L , Ahmari SE , Zeng H , Fenton AA , Hen R . Differential control of learning and anxiety along the dorsoventral axis of the dentate gyrus. Neuron. (2013) ;77: (5):955–68. |
[157] | Snyder JS , Soumier A , Brewer M , Pickel J , Cameron HA . Adult hippocampal neurogenesis buffers stress responses and depressive behaviour. Nature. (2011) ;476: (7361):458–61. |
[158] | Santarelli L , Saxe M , Gross C , Surget A , Battaglia F , Dulawa S , Weisstaub N , Lee J , Duman R , Arancio O , Belzung C , Hen R . Requirement of hippocampal neurogenesis for the behavioral effects of antidepressants. Science. (2003) ;301: (5634):805–9. |
[159] | Jarrard LE . Behavior of hippocampal lesioned rats in home cage and novel situations. Physiology & Behavior. (1968) ;3: (1):65–70. |
[160] | Bannerman DM , Rawlins JN , McHugh SB , Deacon RM , Yee BK , Bast T , Zhang WN , Pothuizen HH , Feldon J . Regional dissociations within the hippocampus–memory and anxiety. Neurosci Biobehav Rev. (2004) ;28: (3):273–83. |
[161] | Deacon RM , Bannerman DM , Rawlins JN . Anxiolytic effects of cytotoxic hippocampal lesions in rats. Behav Neurosci. (2002) ;116: (3):494–7. |
[162] | Kjelstrup KG , Tuvnes FA , Steffenach HA , Murison R , Moser EI , Moser MB . Reduced fear expression after lesions of the ventral hippocampus. Proc Natl Acad Sci U S A. (2002) ;99: (16):10825–30. |
[163] | Epp JR , Spritzer MD , Galea LAM . Hippocampus-dependent learning promotes survival of new neurons in the dentate gyrus at a specific time during cell maturation. Neuroscience. (2007) ;149: (2):273–85. |
[164] | Gould E , Beylin A , Tanapat P , Reeves A , Shors TJ . Learning enhances adult neurogenesis in the hippocampal formation. Nat Neurosci. (1999) ;2: (3):260–5. |
[165] | Leuner B , Waddell J , Gould E , Shors TJ . Temporal discontiguity is neither necessary nor sufficient for learning-induced effects on adult neurogenesis. J Neurosci. (2006) ;26: (52):13437–42. |
[166] | van Praag H , Kempermann G , Gage FH . Neural consequences of environmental enrichment. Nat Rev Neurosci. (2000) ;1: (3):191–8. |
[167] | Eisinger BE , Zhao X . Identifying molecular mediators of environmentally enhanced neurogenesis. Cell and Tissue Research. (2018) ;371: (1):7–21. |
[168] | Tashiro A , Makino H , Gage FH . Experience-specific functional modification of the dentate gyrus through adult neurogenesis: A critical period during an immature stage. J Neurosci. (2007) ;27: (12):3252–9. |
[169] | van Praag H , Kempermann G , Gage FH . Running increases cell proliferation and neurogenesis in the adult mouse dentate gyrus. Nat Neurosci. (1999) ;2: (3):266–70. |
[170] | van Praag H , Shubert T , Zhao C , Gage FH . Exercise enhances learning and hippocampal neurogenesis in aged mice. J Neurosci. (2005) ;25: (38):8680–5. |
[171] | Muotri AR , Zhao C , Marchetto MC , Gage FH . Environmental influence on L1 retrotransposons in the adult hippocampus. Hippocampus. (2009) ;19: (10):1002–7. |
[172] | Snyder JS , Choe JS , Clifford MA , Jeurling SI , Hurley P , Brown A , Kamhi JF , Cameron HA . Adult-born hippocampal neurons are more numerous, faster maturing, and more involved in behavior in rats than in mice. J Neurosci. (2009) ;29: (46):14484–95. |
[173] | Bergami M , Masserdotti G , Temprana SG , Motori E , Eriksson TM , Gobel J , Yang SM , Conzelmann KK , Schinder AF , Gotz M , Berninger B . A critical period for experience-dependent remodeling of adult-born neuron connectivity. Neuron. (2015) ;85: (4):710–7. |
[174] | Piatti VC , Davies-Sala MG , Esposito MS , Mongiat LA , Trinchero MF , Schinder AF . The timing for neuronal maturation in the adult hippocampus is modulated by local network activity. J Neurosci. (2011) ;31: (21):7715–28. |
[175] | Dupret D , Fabre A , Dobrossy MD , Panatier A , Rodriguez JJ , Lamarque S , Lemaire V , Oliet SH , Piazza PV , Abrous DN . Spatial learning depends on both the addition and removal of new hippocampal neurons. PLoS Biol. (2007) ;5: (8):e214. |
[176] | Dobrossy MD , Drapeau E , Aurousseau C , Le Moal M , Piazza PV , Abrous DN . Differential effects of learning on neurogenesis: Learning increases or decreases the number of newly born cells depending on their birth date. Mol Psychiatry. (2003) ;8: (12):974–82. |
[177] | Calegari F , Huttner WB . An inhibition of cyclin-dependent kinases that lengthens, but does not arrest, neuroepithelial cell cycle induces premature neurogenesis. J Cell Sci. (2003) ;116: (Pt 24):4947–55. |
[178] | Castren M , Tervonen T , Karkkainen V , Heinonen S , Castren E , Larsson K , Bakker CE , Oostra BA , Akerman K . Altered differentiation of neural stem cells in fragile X syndrome. Proc Natl Acad Sci U S A. (2005) ;102: (49):17834–9. |
[179] | Bhattacharyya A , McMillan E , Wallace K , Tubon TC Jr , Capowski EE , Svendsen CN . Normal Neurogenesis but Abnormal Gene Expression in Human Fragile X Cortical Progenitor Cells. Stem Cells Dev. (2008) ;17: (1):107–17. |
[180] | Tervonen TA , Louhivuori V , Sun X , Hokkanen ME , Kratochwil CF , Zebryk P , Castren E , Castren ML . Aberrant differentiation of glutamatergic cells in neocortex of mouse model for fragile X syndrome. Neurobiol Dis. (2009) ;33: (2):250–9. |
[181] | Callan MA , Cabernard C , Heck J , Luois S , Doe CQ , Zarnescu DC . Fragile X protein controls neural stem cell proliferation in the Drosophila brain. Hum Mol Genet. (2010) ;19: (15):3068–79. |
[182] | Saffary R , Xie Z . FMRP regulates the transition from radial glial cells to intermediate progenitor cells during neocortical development. J Neurosci. (2011) ;31: (4):1427–39. |
[183] | Lazarov O , Demars MP , Zhao Kda T , Ali HM , Grauzas V , Kney A , Larson J . Impaired survival of neural progenitor cells in dentate gyrus of adult mice lacking fMRP. Hippocampus. (2012) ;22: (6):1220–4. |
[184] | Guo W , Allan AM , Zong R , Zhang L , Johnson EB , Schaller EG , Murthy AC , Goggin SL , Eisch AJ , Oostra BA , Nelson DL , Jin P , Zhao X . Ablation of Fmrp in adult neural stem cells disrupts hippocampus-dependent learning. Nat Med. (2011) ;17: (5):559–65. |
[185] | Luo Y , Shan G , Guo W , Smrt RD , Johnson EB , Li X , Pfeiffer RL , Szulwach KE , Duan R , Barkho BZ , Li W , Liu C , Jin P , Zhao X . Fragile x mental retardation protein regulates proliferation and differentiation of adult neural stem/progenitor cells. PLoS Genet. (2010) ;6: (4):e1000898. |
[186] | Li Y , Stockton ME , Bhuiyan I , Eisinger BE , Gao Y , Miller JL , Bhattacharyya A , Zhao X , MDM2 inhibition rescues neurogenic and cognitive deficits in a mouse model of fragile X syndrome. Sci Transl Med. (2016) ;8: (336):336ra61. |
[187] | Guo W , Murthy AC , Zhang L , Johnson EB , Schaller EG , Allan AM , Zhao X . Inhibition of GSK3beta improves hippocampus-dependent learning and rescues neurogenesis in a mouse model of fragile X syndrome. Hum Mol Genet. (2012) ;21: (3):681–91. |
[188] | Bostrom C , Yau SY , Majaess N , Vetrici M , Gil-Mohapel J , Christie BR . Hippocampal dysfunction and cognitive impairment in Fragile-X Syndrome. Neurosci Biobehav Rev. (2016) ;68: :563–74. |
[189] | Contractor A , Klyachko VA , Portera-Cailliau C . Altered Neuronal and Circuit Excitability in Fragile X Syndrome. Neuron. (2015) ;87: (4):699–715. |
[190] | Guo W , Polich ED , Su J , Gao Y , Christopher DM , Allan AM , Wang M , Wang F , Wang G , Zhao X . Fragile X Proteins FMRP and FXR2P Control Synaptic GluA1 Expression and Neuronal Maturation via Distinct Mechanisms. Cell Re. (2015) ;11: (10):1651–66. |
[191] | Spencer CM , Serysheva E , Yuva-Paylor LA , Oostra BA , Nelson DL , Paylor R . Exaggerated behavioral phenotypes in Fmr1/Fxr2 double knockout mice reveal a functional genetic interaction between Fragile X-related proteins. Hum Mol Genet. (2006) ;15: (12):1984–94. |
[192] | Patzlaff NE , Nemec KM , Malone SG , Li Y , Zhao X . Fragile X related protein 1 (FXR1P) regulates proliferation of adult neural stem cells. Hum Mol Genet. (2017) ;26: (7):1340–52. |
[193] | Mientjes EJ , Willemsen R , Kirkpatrick LL , Nieuwenhuizen IM , Hoogeveen-Westerveld M , Verweij M , Reis S , Bardoni B , Hoogeveen AT , Oostra BA , Nelson DL . Fxr1 knockout mice show a striated muscle phenotype: Implications for Fxr1p function in vivo. Hum Mol Genet. (2004) ;13: (13):1291–302. |
[194] | Zongaro S , Hukema R , D’Antoni S , Davidovic L , Barbry P , Catania MV , Willemsen R , Mari B , Bardoni B . The 3’ UTR of FMR1 mRNA is a target of miR-101, miR-129-5p and miR- Implications for the molecular pathology of FXTAS at the synapse. Hum Mol Genet. (2013) ;22: (10):1971–82. |
[195] | Davidovic L , Sacconi S , Bechara EG , Delplace S , Allegra M , Desnuelle C , Bardoni B . Alteration of expression of muscle specific isoforms of the fragile X related protein 1 (FXR1P) in facioscapulohumeral muscular dystrophy patients. J Med Genet. (2008) ;45: (10):679–85. |
[196] | Yang Q , Feng B , Zhang K , Guo YY , Liu SB , Wu YM , Li XQ , Zhao MG . Excessive astrocyte-derived neurotrophin-3 contributes to the abnormal neuronal dendritic development in a mouse model of fragile X syndrome. PLoS Genet. (2012) ;8: (12):e1003172. |
[197] | Li XY , Cui XS , Kim NH . Transcription profile during maternal to zygotic transition in the mouse embryo. Reprod Fertil Dev. (2006) ;18: (6):635–45. |
[198] | Zheng L , Tanaka H , Abe S . Proteomic analysis of inviable salmonid hybrids between female masu salmon Oncorhynchus masou masou and male rainbow trout Oncorhynchus mykiss during early embryogenesis. J Fish Biol. (2011) ;78: (5):1508–28. |
[199] | Comtesse N , Keller A , Diesinger I , Bauer C , Kayser K , Huwer H , Lenhof HP , Meese E . Frequent overexpression of the genes FXR1, CLAPM1 and EIF4G located on amplicon 3q26-27 in squamous cell carcinoma of the lung. Int J Cancer. (2007) ;120: (12):2538–44. |
[200] | Qian J , Hassanein M , Hoeksema MD , Harris BK , Zou Y , Chen H , Lu P , Eisenberg R , Wang J , Espinosa A , Ji X , Harris FT , Rahman SM , Massion PP . The RNA binding protein FXR1 is a new driver in the 3q26-29 amplicon and predicts poor prognosis in human cancers. Proc Natl Acad Sci U S A. (2015) ;112: (11):3469–74. |
[201] | Sakai Y , Shaw CA , Dawson BC , Dugas DV , Al-Mohtaseb Z , Hill DE , Zoghbi HY . Protein interactome reveals converging molecular pathways among autism disorders. Sci Transl Med. (2011) ;3: (86):86ra49. |
[202] | Schizophrenia-Working-Group, Biological insights from 108 schizophrenia-associated genetic loci. Nature. (2014) ;511: (7510):421–7. |
[203] | Takata A , Matsumoto N , Kato T . Genome-wide identification of splicing QTLs in the human brain and their enrichment among schizophrenia-associated loci. Nature Communications. (2017) ;8: :14519. |
[204] | Liu X , Kelsoe JR , Greenwood TA . A genome-wide association study of bipolar disorder with comorbid eating disorder replicates the SOX2-OT region. Journal of Affective Disorders. (2016) ;189: :141–9. |
[205] | Del’Guidice T , Latapy C , Rampino A , Khlghatyan J , Lemasson M , Gelao B , Quarto T , Rizzo G , Barbeau A , Lamarre C , Bertolino A , Blasi G , Beaulieu JM . FXR1P is a GSK3beta substrate regulating mood and emotion processing. Proc Natl Acad Sci U S A.E. (2015) ;112: (33):4610–9. |
[206] | Bureau A , Beaulieu JM , Paccalet T , Chagnon YC , Maziade M . The interaction of GSK3B and FXR1 genotypes may influence the mania and depression dimensions in mood disorders. Journal of Affective Disorders. (2017) ;213: :172–7. |
[207] | Exome Aggregation Consortium, e.a., Analysis of protein-coding genetic variation in 60,706 humans. biorxiv. (2015) . http://biorxiv.org/content/early/2016/05/10/030338. |
[208] | Stepniak B , Kastner A , Poggi G , Mitjans M , Begemann M , Hartmann A , Van der Auwera S , Sananbenesi F , Krueger-Burg D , Matuszko G , Brosi C , Homuth G , Volzke H , Benseler F , Bagni C , Fischer U , Dityatev A , Grabe HJ , Rujescu D , Fischer A , Ehrenreich H . Accumulated common variants in the broader fragile X gene family modulate autistic phenotypes. EMBO Mol Med. (2015) ;7: (12):1565–79. |
[209] | Crawley JN . Mouse behavioral assays relevant to the symptoms of autism. Brain Pathol. (2007) ;17: (4):448–59. |
[210] | Cook D , Nuro E , Jones EV , Altimimi HF , Farmer WT , Gandin V , Hanna E , Zong R , Barbon A , Nelson DL , Topisirovic I , Rochford J , Stellwagen D , Beique JC , Murai KK . FXR1P limits long-term memory, long-lasting synaptic potentiation, and de novo GluA2 translation. Cell Rep. (2014) ;9: (4):1402–16. |
[211] | Aimone JB , Deng W , Gage FH . Resolving new memories: A critical look at the dentate gyrus, adult neurogenesis, and pattern separation. Neuron. (2011) ;70: (4):589–96. |
[212] | Guo W , Zhang L , Christopher DM , Teng ZQ , Fausett SR , Liu C , George OL , Klingensmith J , Jin P , Zhao X . RNA-binding protein FXR2 regulates adult hippocampal neurogenesis by reducing Noggin expression. Neuron. (2011) ;70: (5):924–38. |